- 1Department of Materials Science, Lomonosov Moscow State University, Moscow, Russia
- 2Department of Chemistry, Lomonosov Moscow State University, Moscow, Russia
- 3Lebedev Physical Institute of the Russian Academy of Sciences, Moscow, Russia
- 4Department of Chemistry, School of Sciences, University of Tokyo, Tokyo, Japan
Structure and properties of an inorganic perovskite Cs2SnI6 demonstrated its potential as a light-harvester or electron-hole transport material; however, its optoelectronic properties are poorer than those of lead-based perovskites. Here, we report the way of light tuning of absorption and transport properties of cesium iodostannate(IV) Cs2SnI6 via partial heterovalent substitution of tin for indium. Light absorption and optical bandgaps of materials have been investigated by UV-vis absorption and photoluminescent spectroscopies. Low-temperature electron paramagnetic resonance spectroscopy was used to study the kind of paramagnetic centers in materials.
Introduction
The efficiency of third-generation solar cells (SCs) based on materials with a perovskite structure is growing every year (Elumalai et al., 2016; Li et al., 2016; Powalla et al., 2018; Sani et al., 2018; Ajay et al., 2019). Such rapid success of using these materials in various fields of solar photovoltaics is due to their unique optoelectronic properties. Today, lead-based perovskite materials (APbX3) are the most efficient in terms of converting solar radiation into electricity (Brandt et al., 2015; Dimesso et al., 2017; Yang et al., 2017; Zhu Z. et al., 2019), and it is caused by low binding energy of excitons, charge carrier mobility, long diffusion length, high absorption coefficient, and direct bandgap (Xiao and Yan, 2017; Deng et al., 2019). The theoretically calculated highest power conversion efficiency (PCE) (Shockley–Queisser limit) achieved by lead-based perovskite is 31.4% for CH3NH3PbI3 (Wan-Jian et al., 2015) and the experimental efficiency (light converting efficiency) of SCs with this compound has exceeded 25% (Yang et al., 2017; Jeon et al., 2018; Powalla et al., 2018). However, the chemical and thermal stability of lead perovskites are not sufficiently well; moreover, lead is a toxic element (Kulbak et al., 2016; Wu Y. et al., 2017; Pisanu et al., 2019). These problems motivate finding new alternatives among lead-free materials with optimal optoelectronic characteristics (Hoefler et al., 2017; Fu, 2019; Pisanu et al., 2019). Instead of lead perovskites, materials with general formulas of ABX3 [where A = CH3, , or Cs+, Rb+, K+; B = divalent inorganic cation; X = Cl, Br, I] and A2BX6 (where A and X the same cations and anions as in the case of ABX3; B = tetravalent inorganic cations) are in focus for investigations (Huang and Lambrecht, 2013; Stoumpos et al., 2016; Cai et al., 2017; Ju et al., 2017). Among all lead-free compounds with the ABX3 formula, cesium triiodostannate CsSnI3 turned out to be the most promising material with good optoelectronic performance (Kumar et al., 2014, 2017; da Silva et al., 2015; Stoumpos et al., 2016). The first SCs based on Sn2+ perovskites had a very low efficiency (3 × 10−4%−2%) (Chen et al., 2012; Kumar et al., 2014). Nevertheless, CsSnI3 has excellent properties [optimal bandgap of 1.4 eV, higher theoretical short-circuit photocurrent (JSC) density of 34.3 mA cm−2, highest hole mobility ~585 cm2 V−1 s−1 among p-type materials] for photovoltaic application as light harvesting or p-type semiconductor (Chung et al., 2012; Stoumpos et al., 2013). Thereby, the CsSnI3 phase is still being actively studied (Song et al., 2018; Wijesekara et al., 2018; Pisanu et al., 2019) and the PCE of SCs based on it has achieved 5.03% (Wang Y. et al., 2019), but these results are still less satisfactory than those of MAPbI3.
Another type of compounds are double perovskites, which are obtained by replacing the tetravalent cation B4+ and a vacancy in A2BX6 with the B+/B3+ pair (where B+ = Cu, Ag, Ga, In, Tl, etc.; B3+ = Sb, Bi etc.) so that the charge neutrality is preserved (Yin et al., 2019). According to Savory et al. (2016), the theoretical calculations show a PCE limit <8% for the double perovskite compound Cs2AgBiX6; however, higher PCE and suitable bandgaps have been predicted for other materials such as Cs2InSbCl6, Cs2AgInBr6, Rb2AgInBr6, and Rb2CuInCl6 (Zhao et al., 2017a,b). According to Xiao et al. (2019), the most promising double perovskites are based on cations such as Ag, In, Bi, and Sb (Greul et al., 2017; Wu C. et al., 2017; Gao et al., 2018; Liang and Gao, 2018; Fan et al., 2019; Igbari et al., 2019).
A promising lead-free non-toxic and stable perovskite-like material with a face-centered cubic cell is A2SnX6, where the tin atom is strongly covalently bonded and stabilizes the crystal lattice. Among all compounds in the A2SnX6 group, the Cs2SnI6 phase turned out to be a suitable material for photovoltaics (Cai et al., 2017; Maughan et al., 2018). Cesium iodostannate(IV) has promising optoelectronic properties (electron mobility up to 509 cm2 V−1 s−1; Guo et al., 2017; JSC = 13.97 mA cm−2; VOC = 0.58 V; Eg = ~1.2 eV) (Kaltzoglou et al., 2016), which makes it a candidate as a light-harvesting material in SCs. Mixed cations A+((AxA1−x)2BX6) (Ganesan et al., 2019) and anions X−(A2B(X'xX1−x)6) (Lee et al., 2017; Ke et al., 2018; Yuan et al., 2019; Zhu W. et al., 2019) were considered to improve stability and properties of Cs2SnX6. Upon replacement of Cl− by I−, the optical absorption coefficient increases, the bandgap decreases, stability decreases, and the materials color changes from pale yellow (or white) to black. The Cs2SnI6 phase is also used as photodetectors (Han et al., 2019), for photoelectrochemical water splitting (Dang et al., 2019), as photocatalysts (Wang X.-D. et al., 2019), and the efficiency of the SCs based on it has reached ~11.2%. In this work, we have demonstrated the preparation of solid solutions based on Cs2SnI6 by doping with indium. Heterovalent substitution of tin (Sn4+ ionic radii 0.69 Å, Pauling electronegativity = 1.96) in the structure of Cs2SnI6 by indium (In3+ ionic radii 0.74 Å, Pauling electronegativity = 1.78) leads to the formation of solid solutions with improved optical properties. Here, for the In3+ → Sn4+ substitution, the difference in electronegativities of cations is 0.18 and the difference in ion sizes is 7.24%, which corresponds well to the Goldschmidt rule (Goldschmidt, 1926).
Recently, the new wave of enthusiasm among researchers has arrived from coming perspectives of nanocrystalline Cs2SnI6 phase and its derivatives for optoelectronics (Wang et al., 2016; Veronese et al., 2020). Because of this, development of nanochemistry of complex halides requires preliminary fundamental studies of phase equilibrium and analysis of optical and transport characteristics in polycrystalline materials.
Experimental Section
Syntheses of Materials
The sintering was carried out by solid-state sintering method in evacuated quartz ampules (RT pressure of 1.6 · 10−2 Torr). The compositions were prepared by grinding cesium iodide CsI (Sigma-Aldrich 99.99%), tin iodide SnI4 [direct synthesis from elementary tin (“Ruschim,” 99.90+, O-1) and iodine in CCl4 (purum, “Irea2000”) with further purification by sublimation at 270°C], and elementary iodine (purum, “Reachim”) and metallic indium (“Ruschim,” 99.999%) with the stoichiometric mass ratios. The pristine Cs2SnI6 phase was obtained with the stoichiometric mass of CsI and SnI4 (2:1). The mixtures were sealed in preliminarily dried quartz ampoules and heated with the rate of ~0.2°C/min to 300°C and then annealed at this temperature for 48 h. All samples were kept in double closed zip-lock bags in nitrogen.
All materials were characterized with powder XRD method for phase composition definition. We assumed that the obtaining materials have the composition related to the solid solutions Cs2−xSn1−xInxI6−2x based on Cs2SnI6 or CsInI4 phases. The following chemical equation shows the phase composition and yield of the reaction products.
Characterization Methods
The ampoules with samples were open right before the following analyses. The samples were transferred to the closely packed cells for further storing. XRD, Raman, and UV-vis measurements were performed in air for 15 min.
X-ray diffraction measurements (XRD) were performed on a Rigaku D/MAX 2500 diffractometer equipped with a rotating copper anode (Cu-Kα radiation) and operated at 45 kV and 250 mA from 5° to 80° in 2Θ; at the continuous scanning speed 5° min−1 with a measuring step of 0.02°. The experimental data were analyzed using WinXPow (database PDF2) to define the phase composition, and Jana2006 software was used for unit cell parameter calculations.
To analyze the optical properties, the samples were studied by diffuse reflection spectroscopy. One gram of each compound was placed in the cell of the spectrophotometer and pressed tightly with a quartz glass and then measured in the range of 1,400–200 nm with a scan step of 1 nm. UV-vis diffuse reflectance spectra were collected using a UV-vis spectrometer Lambda 950 (PerkinElmer). All measurements were performed at 298 K with a scanning rate of 2 nm/s using quartz glass as a reference. Reflectance (R) was converted to absorption (α) data in accordance with the Kubelka–Munk model: α/S = (1 – R)2/(2·R). The optical energy bandgap (Eg) was acquired using a Tauc plot, the dependence of (αhν)2 on energy (hν).
Emission spectra were collected with a multichannel spectrometer S2000 (Ocean Optics) with a nitrogen LGI-21 (λex = 337 nm) as an excitation source at 293 K and 77 K. All spectra were corrected for the wavelength response of the system. Additionally, the photoluminescence emission spectra of the sample CsInI4 was investigated with a diode source of 365 nm.
EPR spectra were recorded using a X-band JES-FA200 (JEOL) spectrometer at the temperature of 294–4.2 K. The modulation frequency is 100 kHz and microwave frequencies are around 9.00 GHz. The samples are put in a quartz tube with an upper glass part, then purged by Ar gas and vacuum three times, and finally sealed in vacuum (about 20 Pa).
Results and Discussion
As shown in Figure 1A, about 10% of tin atoms in the structure of Cs2SnI6 can be substituted by indium atoms. All reflections on the corresponding XRD patterns belong to the Cs2SnI6 with a cubic structure and space group Fm-3m(225) (PDF2 file #73-330). Diffraction patterns for the compositions with x from 0 to 0.15 display a shift of the reflections toward lower 2θ upon increasing the substitution rate. The increase of the unit cell parameter a compared to pure Cs2SnI6 phase indicates a slight increase of the unit cell volume. According to the fact that the ionic radius of the In3+ (0.74 Å) is slightly larger than that of Sn4+ (0.69 Å), the cell volume increases. At higher substitution rates, x of 0.3 and 0.5, reflections of the CsInI4 phase (PDF2 file #76-2101) with a monoclinic structure [P21/c (14) space group] are observed on diffraction patterns of the respective samples. The estimated cell parameters of the F-centered cubic Cs2SnI6 are given in Table 1. Such gentle change of the unit cell parameters is probably due to the formation of iodine vacancies in the anion sublattice.
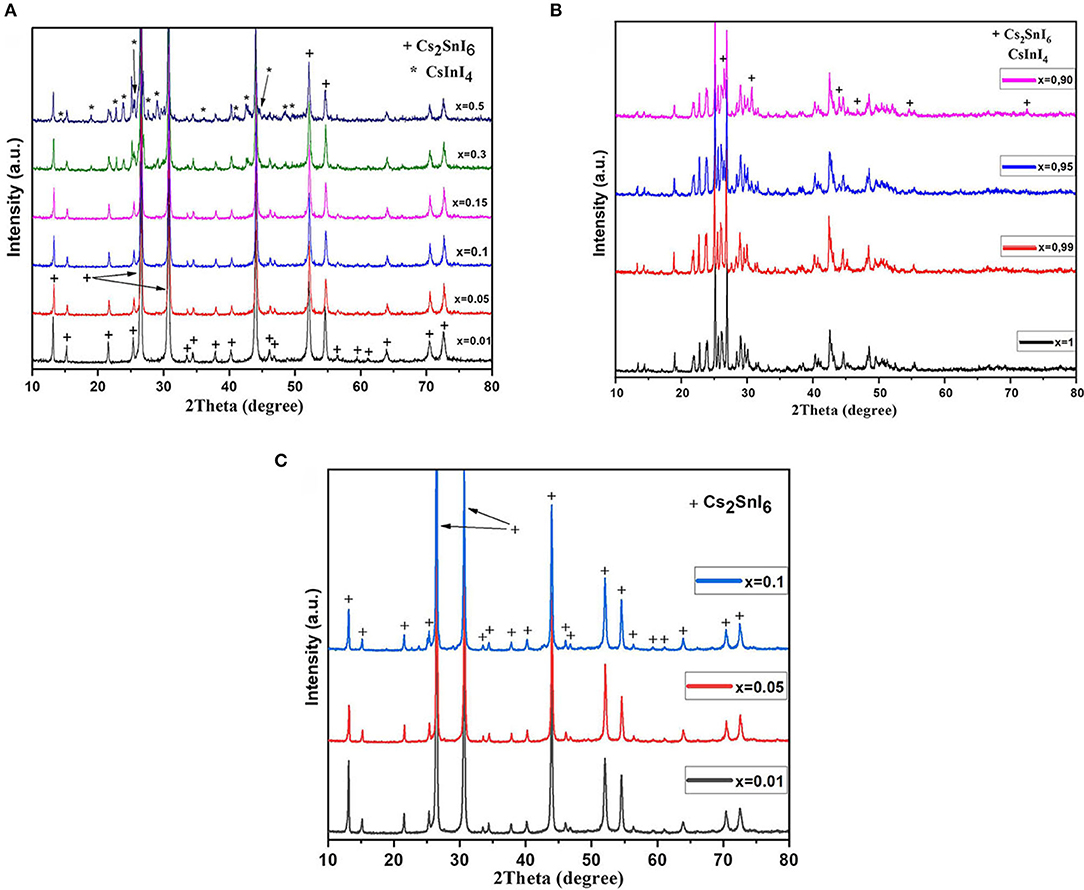
Figure 1. (A) Powder XRD data for Cs2−xSn1−xInxI6−2x (x = 0.01–0.1) compounds. (B) XRD data of Cs2−xSn1−xInxI6−2x with high substitution rate x (x = 0.9 – 1). All unmarked reflections belong to the CsInI4 phase. (C) XRD data of three samples with x = 0.01, 0.05, 0.1 ratio after a year of storage in air. “+” denotes Cs2SnI6 reflections, and “*” indicates reflections of CsInI4.
Samples (polycrystalline powders) were stored in air under laboratory conditions for 1 year and investigated by powder XRD repeatedly during this period. According to the XRD results (Figure 1), the indium-substituted samples remain single-phase with the cubic Cs2SnI6 structure, while the pristine Cs2SnI6 phase (without doping) decomposed already after a month of storage into CsI and volatile SnI4, which turned into SnO2 as a result of hydrolysis. The stability of the substituted samples is probably due to the fact that indium with iodine form stronger ionic bonds than tin; therefore, the lattice of solid solutions is more stable than the pure phase lattice. At the same time, the sample with x = 0.10 has very weak reflections of admixture at 18.77°, 22.65°, and 23.66° in this XRD pattern. These reflections most probably belong to the CsInI4 phase. For comparison, the strongest reflections of cesium iodide CsI should be at 22.87°, 32.56°, and 52.63°. The impurity is probably a result of the segregation process.
The samples of In-substituted Cs2SnI6 were studied by Raman spectroscopy. As can be seen from optical microscopy (Figure 2), the samples are homogeneous in appearance and all crystallites have a similar color. The spectra contain the strongest modes ν(A1g)—122 cm−1, ν(Eg)—83 cm−1, and δ(F2g)—78 cm−1, which are related to vibrations of [SnI6]2− octahedra [namely, ν(A1g) is a symmetric stretching of Sn–X bonds; δ(F2g) is a X–Sn–X asymmetric bending]. Substitution of Sn4+ with In3+ resulted in shortening of M–I bonds and distortion of [MI6]2− octahedrons. Since Cs2SnI6 perfectly absorbs the visible part of the spectrum, the excitation by a green laser (wavelength, 514.4 nm) excites second- and third-order harmonics at 244 and 366 cm−1 due to strong resonance. The relative shift of the lines relative to the theoretical ones in the direction of smaller or larger wave numbers is associated with a changing energy and lengths of the Sn–I bonds in the [SnI6]2− octahedra. Substitution of tin by indium results in small “left shift” and broadening of ν(A1g), and δ(F2g) Raman modes show the increase of element–iodine bond length. Raman spectra of the In-doped Cs2SnI6 differ also from the spectrum reported by Qui et al. for pure Cs2SnI6 (Qiu et al., 2017).
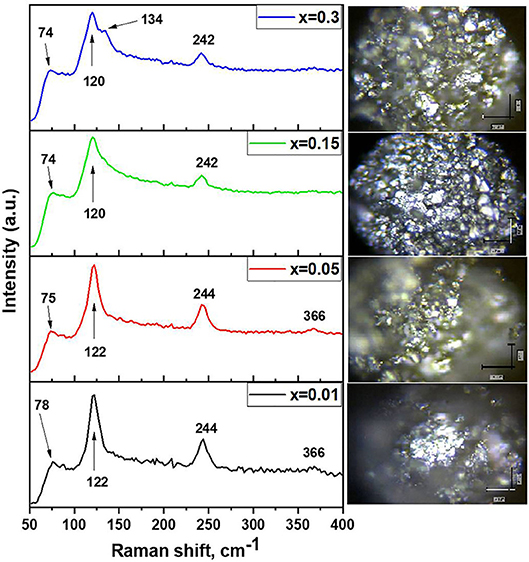
Figure 2. Raman spectra of samples Cs2−xSn1−xInxI6−2x (x = 0.01–0.3) and their optical microscopy data.
In the Raman spectrum of the two-phase sample x = 0.3, a band observed at 134 cm−1 corresponds to the vibration of the [InI4]− tetrahedron. Namely, ν(A1g) is a symmetric stretching vibration of In–I bonds in [InI4]− tetrahedral in CsInI4 phase. Other vibration modes of [InI4]− tetrahedra do not appear in the spectra. It was found that Raman spectrum of the x = 0.15 sample had an additional shoulder at 134 cm−1. The possible reason for this is a fine and evenly distributed admixture of CsInI4. Its XRD reflections do not present in diffractograms, but Raman spectroscopy revealed its presence as the surface admixture. Eventually, this admixture recrystallizes, leading to larger crystallites of cesium iodogallate.
The optical absorption spectra shown in Figure 3 consist of two maxima (at ~800 nm and at ~600 nm). The materials absorb from the near infrared (from 1,000 nm) to ultraviolet (UV) (380 nm). The first local maximum (~800 nm) is due to the transition of the electrons from the maximum of valence band (which is formed from I 5p orbitals) to the minimum of conduction band formed by hybridized I 5p–Sn 5s orbitals. This transition is characteristic for all the samples excluding x = 1. The estimated bandgap energy grows slightly from 1.27 to 1.31 eV for the single-phase samples with x = 0–0.15 according to the corresponding Tauc plots (Figure 3B). The second peak in energy is approximately equal to the electron transfer energy from slightly hybridized I 5p–Sn 5p orbitals localized below the top of the valence band to hybridized I 5p–Sn 5s orbitals of the conduction band.
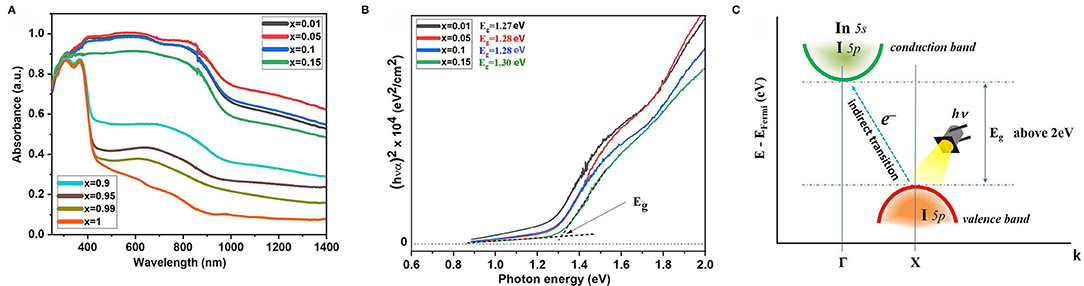
Figure 3. (A) Optical absorption spectra of the samples Cs2−xSn1−xInxI6−2x (x = 0.01–1.0). (B) Tauc plot for Cs2SnI6-based compounds, and (C) scheme of the electronic band structure of CsInI4.
As the indium concentration in the composition of the materials increases, the content of the CsInI4 phase increases, as can be seen from the XRD data, and this is manifested in the absorption spectrum of the sample x = 0.5 (~370 nm). This absorption edge is approximately equal to ~3.0 eV and relates to transitions in the electronic structure of the CsInI4 phase (Figure 3B), namely, the electron transition from the I 5p orbitals (top valence band) to the hybridized I 5p–In 5s orbitals (conduction band bottom). The experimental Eg differs from the calculated bandgap value presented in Persson (2016).
Figure 4A shows photoluminescence spectra of the samples. The C2SnI6 perovskite phase demonstrates rather moderate intensity of luminescence with the 337-nm (3.68 eV) excitation laser, while its In-doped analog shows an intensive violet band at 410 ± 10 nm. Its intensity increases with an increase in the doping level x. Weaker broad maxima in 450–480 nm regions are observed for the samples with lower substitution rate.
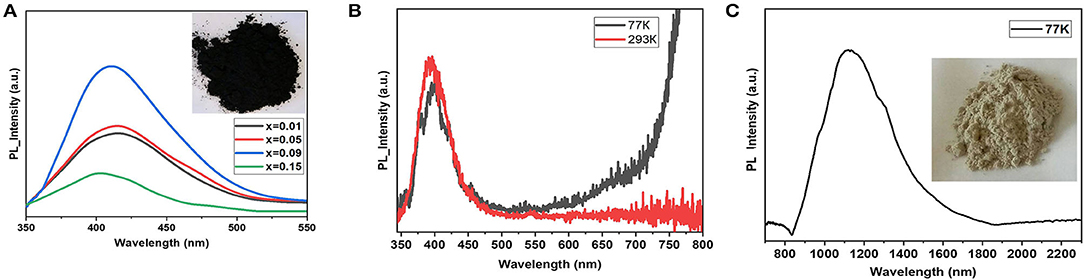
Figure 4. Photoluminescence emission spectra of (A) the samples Cs2−xSn1−xInxI6−2x (x = 0.01–0.15) at 293 K, and (B) and (C) PL emission of pure phase CsInI4 at 293 and 77 K in visible and infrared (IR) range, respectively.
Most likely, the PL of the solid solutions Cs2−xSn1−xInxI6−2x (x = [0; 0.1]) corresponds to cascade relaxation of electrons from the high free levels (anti-bonding derivative of Sn5p0 orbital) to the valence band (Xiao et al., 2015). It has been demonstrated that decrease of the temperature up to 77 K leads to devolution of the PL process. Such behavior is typical for materials with a gap slightly larger than the excitation laser wavelength. The visible photoluminescence in Cs2SnI6 phase is observed as a result of complex relaxation processes. The relaxation of the excited electron to the bottom of the conduction band occurs with visible luminescent process, and the following transition to the top of the valence band has a much lower energy than the observed PL process. Participation of deep levels originated from the point defects (VI) and is another possible reason for the PL effect in the visible diapason (Maughan et al., 2018).
For comparison, the gray cesium iodoindate(III) shows intensive PL bands at 380 ± 10 nm and 400 ± 10 nm in the visible region and intensive PL process in IR diapason at 1,170 ± 20 nm (1.16 eV) (Figures 4B,C). The 380-nm (3.26 eV) band correlates with the experimental band gap transition above while the second maximum in the visible range probably corresponds to shallow defects or self-trapped exciton processes. Intensive IR photoluminescence also originates from self-defect in cesium iodoindate, but the deep level is attributed to deficiency in iodine sublattice.
It is remarkable that the electron spin resonance effect is more significant for the cesium iodostannate(IV) phase with doped indium than for the pure phase. The ESR spectra for the Cs2−xSn1−xInxI6−2x (x = 0.01, 0.05, 0.09, 0.9, and 1) shown in Figure 5 demonstrate week shift of the resonance towards lower field values by the doping of indium from x = 0.01 to x = 0.9, resulting in the shift of the g-factor from 2.0045 to 2.0065. In the range of x = 0.01 to x = 0.09, the integral intensity of the ESR spectra grows up with increase of the substitution level x in the solid solution (Table 2), suggesting increment of the defects. The values of the g-factors and the areas calculated by the integration are given in Table 2. The spectra of the two-phase composition with x of 0.9 include less intensive and broad maximum at 321.2 mT. Probably, there could be an input shoulder related to the tin-doped cesium tetraiodoindate phase while the largest maximum corresponds to the spectrum of the solid solution Cs2−xSn1−xInxI6−2x saturated by In3+. Lower ESR resonance in CsInI4 phase, likely, attributed to lower defect concentration in cesium tetraiodoindate as a result of tetrahedral environment of indium and lower doping level.
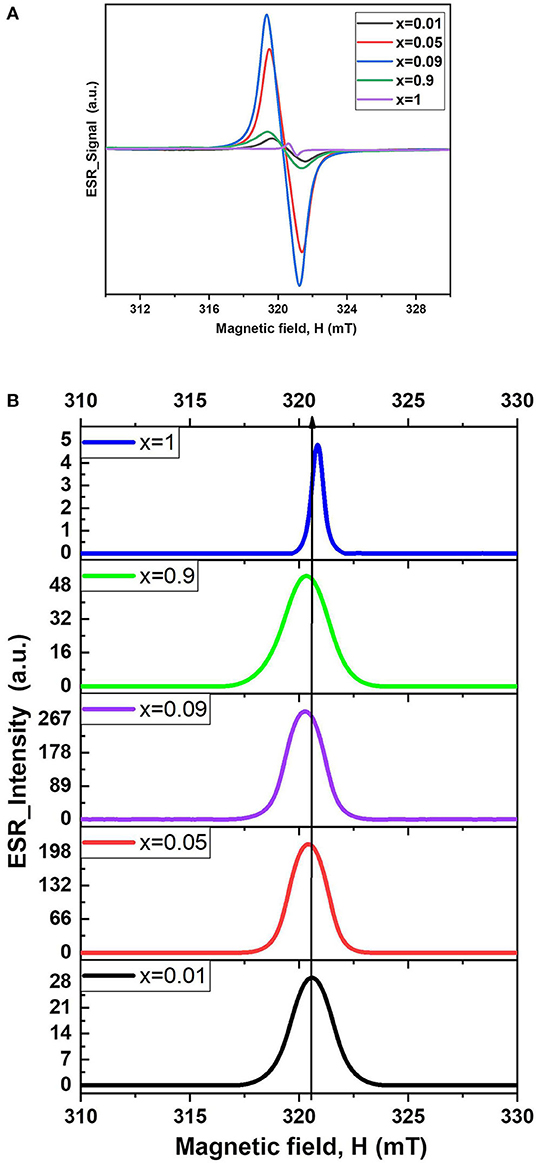
Figure 5. ESR spectra of Cs2−xSn1−xInxI6−2x (with different ratio of x) at 4.2 K (A) in derivative form and (B) in integrated form.
Conclusions
The perovskite-like phase Cs2SnI6 forms substitution solid solutions, changing tin to indium, forming a substitution solid solution of about 10 at.% of dopant. Increase of the indium percentage leads to growth of ESR and photoluminescence effects for the material. The successful experience in heterovalent substitution of tin(IV) with elementary indium and iodine as precursors opens new challenges for “improving” the characteristics of cesium iodostannate for its application in photovoltaics or optoelectronic devices. Weak degradation of doped phase in comparison to pure Cs2SnI6 is a strong advantage of the new investigated materials. Analysis of optical properties has shown an increase of absorption coefficient of the material that originated from growth of occupancy in the valence band. The intensity of blue photoluminescence also grows with a substitution rate up to 15%.
Data Availability Statement
All datasets generated for this study are included in the article/supplementary material.
Author Contributions
SU: synthesis of the samples, Raman spectroscopy experiments and discussion, optical spectroscopy and discussion, ESR experiments. AG: PL spectroscopy and ESR spectroscopy data discussion, correction of the text, and funding. LL: PL spectroscopy experiments. AK: XRD measurements and discussion. KN: ESR experiments and correction of the text. SO: ESR experiment discussion. AS: XRD discussion and correction of the text.
Funding
This project has been performed using the equipment and setups of the collective resource center of Moscow State University Technologies for synthesis of new nanostructured materials and their complex characterization. The reported study was funded by the Russian Foundation for Basic Research according to the research project N 19-03-00849_a.
Conflict of Interest
The authors declare that the research was conducted in the absence of any commercial or financial relationships that could be construed as a potential conflict of interest.
Acknowledgments
The authors are grateful to colleagues Dr. Tatyana A. Shestimerova, and Dr. Valery Yu. Verchenko (Lomonosov Moscow State University) for their assistance in XRD experiments.
References
Ajay, K. J., Ashish, K., and Tsutomu, M. (2019). Halide perovskite photovoltaics: background, status, and future prospects. Chem. Rev. 119, 3036–3103. doi: 10.1021/acs.chemrev.8b00539
Brandt, R. E., Stevanović, V., Ginley, D. S., and Buonassisi, T. (2015). Identifying defect-tolerant semiconductors with high minority-carrier lifetimes: beyond hybrid lead halide perovskites. MRS Commun. 5, 265–275. doi: 10.1557/mrc.2015.26
Cai, Y., Xie, W., Ding, H., Chen, Y., Thirumal, K., Wong, L. H., et al. (2017). Computational study of halide perovskite-derived A2BX6 inorganic compounds: chemical trends in electronic structure and structural stability. Chem. Mater. 29, 7740–7749. doi: 10.1021/acs.chemmater.7b02013
Chen, Z., Wang, J. J., Ren, Y., Yu, C., and Shum, K. (2012). Schottky solar cells based on CsSnI3 thin-films. Appl. Phys. Lett. 101:093901. doi: 10.1063/1.4748888
Chung, I., Song, J. H., Im, J., Androulakis, J., Malliakas, C. D., Li, H., et al. (2012). CsSnI3: semiconductor or metal? High electrical conductivity and strong near-infrared photoluminescence from a single material. High hole mobility and phase-transitions. J. Am. Chem. Soc. 134, 8579–8587. doi: 10.1021/ja301539s
da Silva, E. L., Skelton, J. M., Parker, S. C., and Walsh, A. (2015). Phase stability and transformations in the halide perovskite CsSnI3. Phys. Rev. B 91:144107. doi: 10.1103/PhysRevB.91.144107
Dang, T. C., Le, H. C., Pham, D. L., Nguyen, S. H., Nguyen, T. T. O., Nguyen, T. T., et al. (2019). Synthesis of perovskite Cs2SnI6 film via the solution processed approach: first study on the photoelectrochemical water splitting application. J. Alloys Compd. 805, 847–851. doi: 10.1016/j.jallcom.2019.07.122
Deng, J., Li, J., Yang, Z., and Wang, M. (2019). All-inorganic lead halide perovskites: a promising choice for photovoltaics and detectors. J. Mater. Chem. C 7, 12415–12440. doi: 10.1039/C9TC04164H
Dimesso, L., Das, C., Stöhr, M., and Jaegermann, W. (2017). Investigation of cesium tin/lead iodide (CsSn1−xPbxI3) systems. Mater. Res. Bull. 85, 80–89. doi: 10.1016/j.materresbull.2016.08.052
Elumalai, N. K., Mahmud, M. A., Wang, D., and Uddin, A. (2016). Perovskite solar cells: progress and advancements. Energies 861, 1–20. doi: 10.3390/en9110861
Fan, Q., McGee, G. V. B., Ma, J., Xu, Q., Pan, S., Peng, J., et al. (2019). Lead-free halide perovskite nanocrystals: crystal structures, synthesis, stabilities, and optical properties. Angew. Chem. Int. Ed. 59, 1030–1046. doi: 10.1002/anie.201904862
Fu, H. (2019). Review of lead-free halide perovskites as light-absorbers for photovoltaic applications: from materials to solar cells. Solar Energy Mater. Solar Cells 193, 107–132. doi: 10.1016/j.solmat.2018.12.038
Ganesan, R., Vinodhini, S. P., Balasubramani, V., Parthipan, G., Sridhar, T. M., Arulmozhi, M., et al. (2019). Tuning the band gap of hybrid lead free defect perovskite nano crystals for solar cell applications. New J. Chem. 43, 15258–15266. doi: 10.1039/C9NJ03902C
Gao, W., Ran, C., Xi, J., Jiao, B., Zhang, W., Wu, M., et al. (2018). High-quality Cs2AgBiBr6 double perovskite film for lead-free inverted planar heterojunction solar cells with 2.2 % efficiency. ChemPhysChem 19, 1696–1700. doi: 10.1002/cphc.201800346
Greul, E., Petrus, M. L., Binek, A., Docampo, P., and Bein, T. (2017). Highly stable, phase pure Cs2AgBiBr6 double perovskite thin films for optoelectronic applications. J. Mater. Chem. A 37, 5, 19972–19981. doi: 10.1039/C7TA06816F
Guo, F., Lu, Z., Mohanty, D., Wang, T., Bhat, I. B., Zhang, S., et al. (2017). A two-step dry process for Cs2SnI6 perovskite thin film. Mater. Res. Lett. 5, 540–546. doi: 10.1080/21663831.2017.1346525
Han, X., Liang, J., Yang, J.-H., Soni, K., Fang, Q., Wang, W., et al. (2019). Lead-free double perovskite Cs2SnX6: facile solution synthesis and excellent stability. Small 15:1901650. doi: 10.1002/smll.201901650
Hoefler, S. F., Trimmel, G., and Rath, T. (2017). Progress on lead-free metal halide perovskites for photovoltaic applications: a review. Monatsh. Chem. Chem. 2017, 148, 795–826. doi: 10.1007/s00706-017-1933-9
Huang, L.-,y., and Lambrecht, W. R. L. (2013). Electronic band structure, phonons, and exciton binding energies of halide perovskites CsSnCl3, CsSnBr3, and CsSnI3. Phys. Rev. B 88:165203. doi: 10.1103/PhysRevB.88.165203
Igbari, F., Wang, Z.-K., and Liao, L.-S. (2019). Progress of lead-free halide double perovskites. Adv. Energy Mater. 9:1803150. doi: 10.1002/aenm.201803150
Jeon, N. J., Na, H., Jung, E. H., Yang, T.-Y., Lee, Y. G., Kim, G., et al. (2018). A fluorene-terminated hole-transporting material for highly efficient and stable perovskite solar cells. Nat. Energy 3, 682–689. doi: 10.1038/s41560-018-0200-6
Ju, M.-G., Chen, M., Zhou, Y., Garces, H. F., Dai, J., Ma, L., et al. (2017). Earth-abundant nontoxic titanium(IV)-based vacancy-ordered double perovskite halides with tunable 1.0 to 1.8 eV bandgaps for photovoltaic applications. ACS Energy Lett. 3, 297–304. doi: 10.1021/acsenergylett.7b01167
Kaltzoglou, A., Perganti, D., Antoniadou, M., Kontos, A. G., and Falaras, P. (2016). Stress tests on dye-sensitized solar cells with the Cs2SnI6 defect perovskite as hole-transporting material. Energy Procedia 102, 49–55. doi: 10.1016/j.egypro.2016.11.317
Ke, J. C.-R., Lewis, D. J., Walton, A. S., Spencer, B. F., O'Brien, P., Thomas, A. G., et al. (2018). Ambient-air-stable inorganic Cs2SnI6 double perovskite thin films via aerosol-assisted chemical vapour deposition. J. Mater. Chem. A 6, 11205–11214. doi: 10.1039/C8TA03133A
Kulbak, M., Gupta, S., Kedem, N., Levine, I., Bendikov, T., Hodes, G., et al. (2016). Cesium enhances long-term stability of lead bromide perovskite-based solar cells. J. Phys. Chem. Lett. 7, 167–172. doi: 10.1021/acs.jpclett.5b02597
Kumar, G. R., Kim, H.-J., Karupannan, S., and Prabhakar, K. (2017). Interplay between Iodide and tin vacancies in CsSnI3 perovskite solar cells. J. Phys. Chem. C 121, 16447–16453. doi: 10.1021/acs.jpcc.7b06278
Kumar, M. H., Dharani, S., Leong, W. L., Boix, P. P., Prabhakar, R. R., Baikie, T., et al. (2014). Lead-free halide perovskite solar cells with high photocurrents realized through vacancy modulation. Adv. Mater. 26, 7122–7127. doi: 10.1002/adma.201401991
Lee, B., Krenselewski, A., Baik, S. I., Seidman, D. N., and Chang, R. P. H. (2017). Solution processing of air-stable molecular semiconducting iodosalts, Cs2SnI6−xBrx, for potential solar cell applications. Sustain. Energy Fuels 1, 710–724. doi: 10.1039/C7SE00100B
Li, M. H., Yum, J. H., Moon, S. J., and Chen, P. (2016). Inorganic p-type semiconductors: their applications and progress in dye-sensitized solar cells and perovskite solar cells. Energies. 9:331. doi: 10.3390/en9050331
Liang, L., and Gao, P. (2018). Lead-free hybrid perovskite absorbers for viable application: can we eat the cake and have it too? Adv. Sci. 5:1700331. doi: 10.1002/advs.201700331
Maughan, A. E., Ganose, A. M., Almaker, M. A., Scanlon, D. O., and Neilson, J. R. (2018). Tolerance factor and cooperative tilting effects in vacancy-ordered double perovskite halides. Chem. Mater. 30, 3909–3919. doi: 10.1021/acs.chemmater.8b01549
Persson, K. (2016). Materials Data on CsInI4 (SG:14) by Materials Project. Berkeley, CA, 7. doi: 10.17188/1277593
Pisanu, A., Speltini, A., Quadrelli, P., Drera, G., Sangaletti, L., and Malavasi, L. (2019) Enhanced air-stability of Sn-based hybrid perovskites induced by dimethylammonium (DMA): synthesis, characterization, aging hydrogen photogeneration of the MA1−−xDMAxSnBr3 system. J. Mater. Chem. C7, 7020–7026. doi: 10.1039/C9TC01743G
Powalla, M., Paetel, S., Ahlswede, E., Wuerz, R., Wasserdorf, C. D., and Friedlmeier, T. M. (2018). Thin film solar cells exceeding 22% solar cell efficiency: an overview on CdTe−, Cu(In,Ga)Se2−, and perovskite-based materials. Appl. Phys. Rev. 5:041602. doi: 10.1063/1.5061809
Qiu, X., Cao, B., Yuan, S., Chen, X., Qiu, Z., Jiang, Y., et al. (2017). From unstable CsSnI3 to air-stable Cs2SnI6: A lead-free perovskite solar cell light absorber with bandgap of 1.48eV and high absorption coefficient. Solar Energy Mater. Solar Cells 159, 227–234. doi: 10.1016/j.solmat.2016.09.022
Sani, F., Shafie, S., Lim, H., and Musa, A. (2018). Advancement on lead-free organic-inorganic halide perovskite solar cells: a review. Materials 11:E1008. doi: 10.3390/ma11061008
Savory, C. N., Walsh, A., and Scanlon, D. O. (2016). Can Pb-free halide double perovskites support high-efficiency solar cells? ACS Energy Lett. 1, 949–955. doi: 10.1021/acsenergylett.6b00471
Song, T.-B., Yokoyama, T., Longsdon, J., Wasielewski, M. R., Aramaki, S., and Kanatzidis, M. G. (2018). Piperazine suppresses self-doping in CsSnI3 perovskite solar cells. ACS Appl. Energy Mater. 1, 4221–4226. doi: 10.1021/acsaem.8b00866
Stoumpos, C. C., Malliakas, C. D., and Kanatzidis, M. G. (2013). Semiconducting tin and lead iodide perovskites with organic cations: phase transitions, high mobilities, and near-Infrared photoluminescent properties. Inorg. Chem. 52, 9019–9038. doi: 10.1021/ic401215x
Stoumpos, C. C., Mao, L., Malliakas, C. D., and Kanatzidis, M. G. (2016). Structure–band gap relationships in hexagonal polytypes and low-dimensional structures of hybrid tin iodide perovskites. Inorg. Chem. 56, 56–73. doi: 10.1021/acs.inorgchem.6b02764
Veronese, A., Patrini, M., Bajoni, D., Ciarrocchi, C., Quadrelli, P., and Malavasi, L. (2020). Highly tunable emission by halide engineering in lead-free perovskite-derivative nanocrystals: The Cs2SnX6 (X = Cl, Br, Br/I, I) system. Front. Chem. 8:35. doi: 10.3389/fchem.2020.00035
Wang, A., Yan, X., Zhang, M., Sun, S., Yang, M., Shen, W., et al. (2016). Controlled synthesis of lead-free and stable perovskite derivative Cs2SnI6 nanocrystals via a facile hot-injection process. Chem. Mater. 28, 8132–8140. doi: 10.1021/acs.chemmater.6b01329
Wang, X.-D., Huang, Y.-H., Liao, J.-F., Jiang, J., Zhou, L., Zhang, X.-Y., et al. (2019). In situ construction of a Cs2SnI6 perovskite nanocrystal/SnS2 nanosheet heterojunction with boosted interfacial charge transfer. J. Am. Chem. Soc. 141, 13434–13441. doi: 10.1021/jacs.9b04482
Wang, Y., Tu, J., Li, T., Tao, C., Deng, X., and Li, Z. (2019). Convenient preparation of CsSnI3 quantum dots, excellent stability, and the highest performance of lead-free inorganic perovskite solar cells so far. J. Mater. Chem. A 7, 7683–7690. doi: 10.1039/C8TA10901J
Wan-Jian, Y., Ji-Hui, Y., Kang, J., Yan, Y., and Wei, S.-H. (2015). Halide perovskite materials for solar cells: a theoretical review. J. Mater. Chem. A 3, 8926–8942. doi: 10.1039/C4TA05033A
Wijesekara, A., Varagnolo, S., Dabera, G. D. M. R., Marshall, K. P., Pereira, H. J., and Hatton, R. A. (2018). Assessing the suitability of copper thiocyanate as a hole-transport layer in inverted CsSnI3 perovskite photovoltaics. Sci. Rep. 8:15722. doi: 10.1038/s41598-018-33987-7
Wu, C., Zhang, Q., Liu, Y., Luo, W., Guo, X., Huang, Z., et al. (2017). The dawn of lead-free perovskite solar cell: highly stable double perovskite Cs2AgBiBr6 film. Adv. Sci. 5:1700759. doi: 10.1002/advs.201700759
Wu, Y., Xie, F., Chen, H., Yang, H., Su, H., Cai, M., et al. (2017). Thermally stable MAPbI3 perovskite solar cells with efficiency of 19.19% and area over 1cm2 achieved by additive engineering. Adv. Mater. 29:1701073. doi: 10.1002/adma.201701073
Xiao, Z., Lei, H., Zhang, X., Zhou, Y., Hosono, H., and Kamiya, T. (2015). Ligand-hole in [SnI6] unit and origin of band gap in photovoltaic perovskite variant Cs2SnI6. Bull. Chem. Soc. Jpn. 88, 1250–1255. doi: 10.1246/bcsj.20150110
Xiao, Z., Song, Z., and Yan, Y. (2019). From lead halide perovskites to lead-free metal halide perovskites and perovskite derivatives. Adv. Mater. 31:1803792. doi: 10.1002/adma.201803792
Xiao, Z., and Yan, Y. (2017). Progress in theoretical study of metal halide perovskite solar cell materials. Adv. Energy Mater. 7:1701136. doi: 10.1002/aenm.201701136
Yang, W. S., Park, B. W., Jung, E. H., Jeon, N. J., Kim, N. C., Lee, D. U., et al. (2017). Iodide management in formamidinium-lead-halide–based perovskite layers for efficient solar cells. Science 356, 1376–1379. doi: 10.1126/science.aan2301
Yin, H., Xian, Y., Zhang, Y., Li, W., and Fan, J. (2019). Structurally-stabilizing and friendly-environmental triggers: double-metallic lead-free perovskites. Solar RRL 3:1900148. doi: 10.1002/solr.201900148
Yuan, G., Huang, S., Qin, S., Wu, X., Ding, H., and Lu, A. (2019). Structural, optical and thermal properties of Cs2SnI6−xBrx mixed perovskite solid solution. Eur. J. Inorg. Chem. 20, 2524–2529. doi: 10.1002/ejic.201900120
Zhao, X.-G., Yang, D., Sun, Y., Li, T., Zhang, L., Yu, L., et al. (2017b). Cu–In halide perovskite solar absorbers. J. Am. Chem. Soc. 139, 6718–6725. doi: 10.1021/jacs.7b02120
Zhao, X.-G., Yang, J.-H., Fu, Y., Yang, D., Xu, Q., Yu, L., et al. (2017a). Design of lead-free inorganic halide perovskites for solar cells via cation-transmutation. J. Am. Chem. Soc. 139, 2630–2638. doi: 10.1021/jacs.6b09645
Zhu, W., Yao, T., Shen, Y., Xu, W., Gong, B., Wang, Y., et al. (2019). In situ investigation of water interaction with lead-free all inorganic perovskite (Cs2SnIxCl6−x). J. Phys. Chem. C 123, 14, 9575–9581. doi: 10.1021/acs.jpcc.9b00720
Keywords: perovskite photovoltaics, cesium iodostannate(IV), solid solution, Raman spectroscopy, optical characteristics
Citation: Umedov ST, Grigorieva AV, Lepnev LS, Knotko AV, Nakabayashi K, Ohkoshi S and Shevelkov AV (2020) Indium Doping of Lead-Free Perovskite Cs2SnI6. Front. Chem. 8:564. doi: 10.3389/fchem.2020.00564
Received: 15 February 2020; Accepted: 02 June 2020;
Published: 04 August 2020.
Edited by:
W. H. Eugen Schwarz, University of Siegen, GermanyReviewed by:
Thiti Bovornratanaraks, Chulalongkorn University, ThailandLorenzo Malavasi, University of Pavia, Italy
Copyright © 2020 Umedov, Grigorieva, Lepnev, Knotko, Nakabayashi, Ohkoshi and Shevelkov. This is an open-access article distributed under the terms of the Creative Commons Attribution License (CC BY). The use, distribution or reproduction in other forums is permitted, provided the original author(s) and the copyright owner(s) are credited and that the original publication in this journal is cited, in accordance with accepted academic practice. No use, distribution or reproduction is permitted which does not comply with these terms.
*Correspondence: Anastasia V. Grigorieva, YW5hc3Rhc2lhJiN4MDAwNDA7aW5vcmcuY2hlbS5tc3UucnU=