- 1College of Chemical Engineering, Beijing University of Chemical Technology, Beijing, China
- 2School of Chemistry and Chemical Engineering, Shandong Provincial Key Laboratory of Chemical Energy Storage and Novel Cell Technology, Liaocheng University, Liaocheng, China
Zinc-based flow batteries have gained widespread attention and are considered to be one of the most promising large-scale energy storage devices for increasing the utilization of intermittently sustainable energy. However, the formation of zinc dendrites at anodes has seriously depressed their cycling life, security, coulombic efficiency, and charging capacity. Inhibition of zinc dendrites is thus the bottleneck to further improving the performance of zinc-based flow batteries, but it remains a major challenge. Considering recent developments, this mini review analyzes the formation mechanism and growth process of zinc dendrites and presents and summarizes the strategies for preventing zinc dendrites by regulating the interfaces between anodes and electrolytes. Four typical strategies, namely electrolyte modification, anode engineering, electric field regulation, and ion transfer control, are comprehensively highlighted. Finally, remaining challenges and promising directions are outlined and anticipated for zinc dendrites in zinc-based flow batteries.
Introduction
Energy and environment are the foundation of human survival and development (Zhang et al., 2019a). To meet increasing requirements, people are exploring sustainable and clean energy (Turner, 1999). However, sustainable and clean energy, represented by wind, solar, and tidal, are affected by climate and cannot directly generate continuous and stable electrical power (Yang et al., 2011; Lou et al., 2020). Large-scale energy storage devices seem to be the best choice for collecting the fluctuating energy and outputting high-quality power (Dunn et al., 2011; Leadbetter and Swan, 2012).
Flow batteries have received widespread attention due to their high safety and low cost (Liu et al., 2019a; Zhang et al., 2019a; Ye et al., 2020a,b). Their power and capacity can be designed independently. The power is determined by the number and size of the stacks, while the capacity is limited by the volume and concentration of the electrolyte outside stacks. Their capacity can be easily be increased by increasing the number of redox couples in the electrolyte without adding other equipment. Therefore, flow batteries are very suitable for large-scale energy storage.
Zinc-based flow batteries (ZFBs) have the advantages of low cost, high safety, flexible structure, and high energy efficiency and have been extensively studied (Arenas et al., 2018). Various ZFBs have been proposed, such as the zinc-bromine flow battery (Jeon et al., 2014; Suresh et al., 2014), zinc-iodine flow battery (Xie et al., 2019), zinc-nickel flow battery (Cheng et al., 2014b, 2019c; Huang et al., 2018), zinc-air flow battery (Cheng et al., 2018, 2019b), zinc-iron flow battery (Yuan et al., 2018a; Chang et al., 2019), and zinc-manganese flow battery (Liu et al., 2020). Some of these flow batteries, like the zinc-bromine flow battery, zinc-nickel flow battery, zinc-air flow battery, and zinc-iron battery, are already in the demonstration stage and are close to commercial application (Arenas et al., 2018).
The structure and mechanism of ZFBs are shown in Figure 1A. The electrochemical reaction at the anode side is zinc deposition and stripping. This is a little different in aqueous acid/neutral and alkaline solutions (Khor et al., 2018).
(1) Acid or neutral solution
Zn2+ + 2e− ↔ Zn Eo = −0.763 V vs. SHE
(2) Alkaline solution
vs. SHE
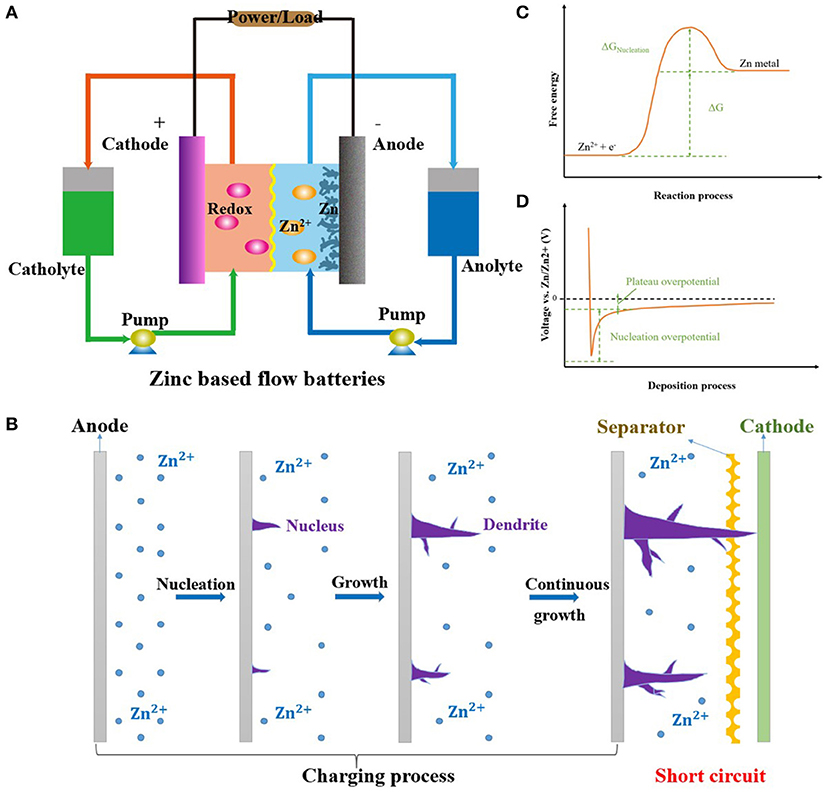
Figure 1. (A) Principle and structure of typical ZFBs. (B) Schematic of the nucleation and growth processes of zinc dendrites. (C) The energy barrier for the zinc nucleation and growth processes. (D) Overpotential of zinc nucleation and growth processes. Reproduced from Pei et al. (2017) with permission. Copyright 2017 American Chemical Society.
However, zinc dendrites are formed during the charging process and eventually pierce the separator, resulting in short circuit and battery failure (Figure 1B). Moreover, zinc dendrites can easily fall from anodes, resulting in a decrease in efficiency and capacity (Cheng et al., 2014a). Therefore, inhibiting zinc dendrite formation is very important for the further development of ZFBs. Recently, researchers have done a lot of work to solve zinc dendrite formation through modifications to the electrolyte, anodes, electric field, and zinc ion transfer. In this review, we will introduce the formation and growth mechanism of zinc dendrites, summarize typical methods for solving zinc dendrite formation, and outline promising future directions.
Formation Mechanism of Zinc Dendrites
Zinc deposition begins with nucleation and continues with growth (Yufit et al., 2019; Zheng et al., 2019). The energy barrier for zinc nucleation is much higher than for zinc growth on the nucleus, as shown in Figure 1C (Zeng et al., 2019; Zhang et al., 2020). As a result, the overpotential of zinc nucleation is also larger than that of zinc growth on the nucleus (Figure 1D; Zhang et al., 2019b, 2020). This indicates that once a zinc nucleus forms, zinc ions prefer to deposit on the nucleus rather than to produce a new nucleus. Moreover, small nuclei have high surface energy and thermodynamically tend to aggregate into larger particles (Pei et al., 2017; Cheng et al., 2019a). Therefore, it is very difficult to obtain uniform zinc nuclei on the anode.
During the growth process, zinc ions migrate to a nucleus under the driving forces of electric fields and concentration gradients (Wang et al., 2015; Lacitignola et al., 2017). The distributions of the electric field and zinc ions at the interface between anodes and electrolytes play an important role in zinc deposition (Cheng et al., 2013b, 2014a). A uniform electric field is favorable for both the nucleation and growth of zinc deposits. Unfortunately, the electric field is much stronger in the areas adjacent to current collectors than at edges and corners far away from current collectors (Cheng et al., 2013b). After zinc ions at the interface are consumed, zinc ions that exist in the electrolyte far from the interface cannot migrate to the interface in time, resulting in severe concentration polarization (Wang et al., 2015). Simultaneously, zinc ions preferentially migrate to the protruding tips of anodes and subsequently grow on previously deposited zinc seeds, which accelerates the formation of zinc dendrites (Lu et al., 2018). Additionally, hydrogen evolution at an anode also makes mass transfer more difficult (Ito et al., 2011a; Dundalek et al., 2017). This phenomenon is more serious in the case of the rapid deposition of zinc ions at large anodes (Cheng et al., 2015, 2019c).
Strategies to Prevent Zinc Dendrite Formation
Recently, various methods have been proposed to inhibit zinc dendrite formation, including electrolyte modification (Wen et al., 2012; Banik and Akolkar, 2013; Kim et al., 2019), anode engineering (Lin, 2018; Suresh et al., 2019; Yin et al., 2020), electric field regulation (Cheng et al., 2014a; Nikiforidis et al., 2014; Yuan et al., 2018b), and ion transfer control (Ito et al., 2011b; Song et al., 2014; Wang et al., 2014). In this section, we will introduce the typical solutions for preventing zinc dendrite formation in ZFBs from the above four aspects, as shown in Figure 2.
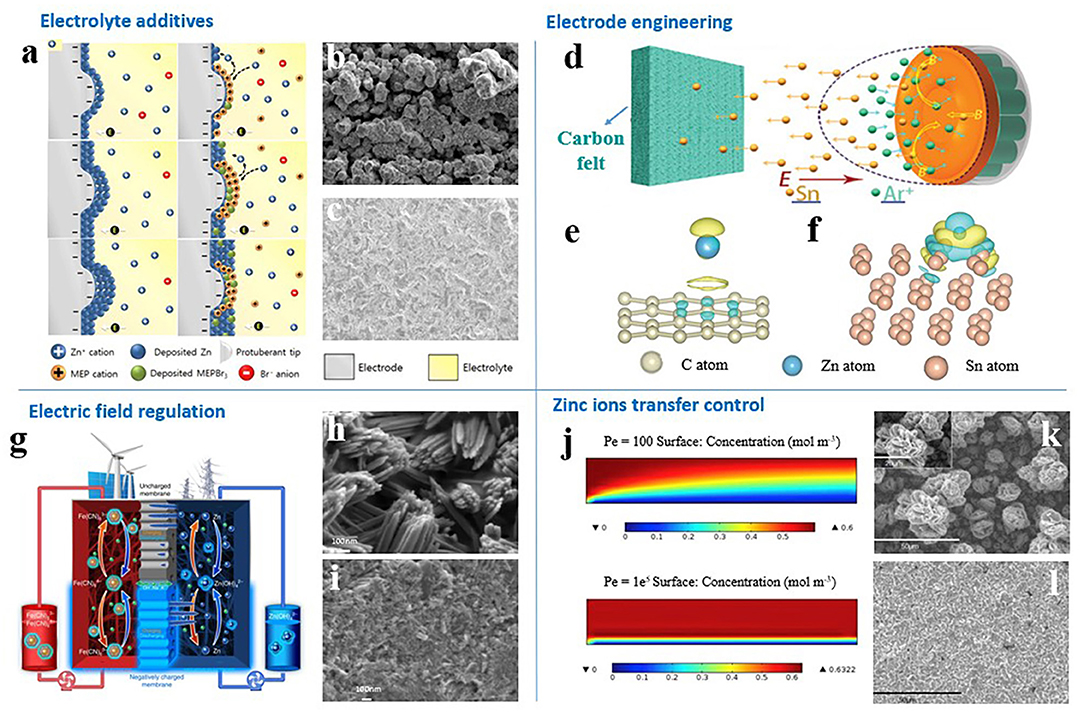
Figure 2. (a) Illustration of the prevention of zinc dendrite formation by cationic 1-Ethyl-1-methyl-pyrrolidinium bromide (MEP·Br) through an electrostatic shielding effect. (b) Without and (c) with 1.2 M MEP·Br in 2.0 M zinc-bromide electrolyte solution. X100 magnification of deposited zinc. (a–c) Reproduced from Kim et al. (2019) with permission. Copyright 2019 Elsevier. (d) Schematic illustration for the fabrication process of Sn-coated carbon felt. Interfacial charge-density of (e) carbon and (f) Sn based on DFT calculation. (d–f) Reproduced from Yin et al. (2020) with permission. Copyright 2020 Wiley-VCH. (g–i) Schematic of zinc deposition when employing an uncharged (top) and a negatively charged (bottom) membrane in a zinc–iron flow battery, and corresponding zinc morphologies at the end of charging. Reproduced from Yuan et al. (2018b) with permission. Copyright 2018 Nature Publishing Group. (j) Electrolyte concentration distribution at Pe = 100 and Pe = 1e5. Morphologies of deposited zinc in (k) quiescent alkaline electrolyte and (l) flowing alkaline electrolyte at a current density of 100 mA cm−2. (j–l) Reproduced from Wang et al. (2014) with permission. Copyright 2014 Elsevier.
Electrolyte Modification
Organic molecules, polymers, and metal ions are common additives for inhibiting zinc dendrites. Organic molecules and polymers can selectively adsorb onto the protruding parts of anodes and act as a barrier to the access of zinc ions (Mitha et al., 2018; Chladil et al., 2019). Therefore, they prevent zinc deposition on protruding parts and accelerate zinc nucleation and growth on dents by steric effects and/or electrostatic shielding. Compared with polymers, organic molecules have shorter chain lengths, and smaller end steric hindrances. Organic molecules are more likely to cover protruding parts and delay the deposition of zinc ions. Generally, the higher the polarity of the organic additives, the stronger the adsorption on anodes. However, excessively strong adsorption will result in severe electrochemical polarization for zinc deposition. Metal ions can affect the nucleation of zinc and thereby influence the growing process, and so, a uniform and compact zinc deposits layer can be obtained.
Organic molecules include non-ionic dimethyl sulfoxide (Hosseini et al., 2019), thiourea (Goh et al., 2014; Sun et al., 2017), diethyl ether (Xu et al., 2019), polyacrylic acid (Shimizu et al., 2019); cationic quaternary ammonium (Rossi et al., 2020), benzyl trimethyl ammonium hydroxide (Liu et al., 2019b), trimethyl octadecyl ammonium chloride (Shimizu et al., 2019), hexadecyl trimethyl ammonium bromide (Chladil et al., 2019), anionic sodium dodecyl sulfate (Miyazaki et al., 2016; Hosseini et al., 2018; Shimizu et al., 2019), and EMI-PF6 and EMI-TFSA (Song et al., 2016). Polymers include polyethyleneimine (Banik and Akolkar, 2015; Hashemi et al., 2017), Triton X-100 (Kan et al., 1998), polyvinyl alcohol (Ortiz-Aparicio et al., 2013), polyethylene glycol (Lee et al., 2006a; Ballesteros et al., 2007; Banik and Akolkar, 2013), polyacrylamide (Zhang et al., 2019b), Tween 20 (Chladil et al., 2019), and Pluronic F-127 (Hosseini et al., 2018). Metal ions comprise Pb2+ (Justinijanović et al., 1973; Wen et al., 2012), Sn2+ (Yuan et al., 2007; Kim and Shin, 2015; Yao et al., 2019), Bi3+ (Wang et al., 2001), In3+ (Leung et al., 2011), and La3+ (Yang et al., 2004).
For example, cationic 1-Ethyl-1-methyl-pyrrolidinium bromide was employed as an additive in electrolytes of zinc-bromine flow batteries to prevent zinc-dendrite development through forming an electrostatic shield in and around the zinc dendrite during the charging process (Figures 2a–c; Kim et al., 2019). The zinc deposits were uniform and compact, but the charging overpotential increased by 47 mV, and discharging overpotential increased by 98 mV. The cycling life of zinc-bromine flow batteries was improved by sacrificing voltage efficiency.
Tin ions promote the formation of crystal seeds and substantially improve the charge retention of the zinc-nickel flow battery. Interestingly, only a slightly negative shift in the initial potential of zinc nucleation was observed, and the rate performance and polarization properties of zinc anodes were no significantly reduced (Yao et al., 2019).
The synergy between various additives should also be noted. The synergistic effect of lead ions and TBAB can inhibit the growth of zinc dendrites, thereby obtaining smooth and dense zinc deposits in alkaline zincate electrolytes. This is beneficial for improving the cycling life of zinc-nickel flow batteries (Wen et al., 2012).
Anode Engineering
The physicochemical properties and structure of anodes have an important effect on zinc deposition (Wei et al., 2016; Parker et al., 2017; Jiang et al., 2018). As zinc randomly deposits onto and strips from the anode, cracks are inevitable after repeated charge-discharge cycles when using pure zinc foils/sheets as anodes (Cheng et al., 2019b). To avoid rapid failure, conductive materials are usually used as a host for zinc deposition/dissolution, such as carbon (Jiang et al., 2018; Lin, 2018; Shen et al., 2018; Suresh et al., 2019; Zeng et al., 2019; Zhang et al., 2019b), nickel (Cheng et al., 2013a), copper (Zhang et al., 2019b), lead (Zhang et al., 2008), tin (Yin et al., 2020), chromium (Zhang et al., 2008), indium (Lee et al., 2006b; Nikiforidis and Daoud, 2015), and their compounds (Kang et al., 2018). Additionally, a traditional flat electrode has a low specific surface area and limits the charging current and capacity (Cheng et al., 2013b). Further design or modifications of anodes is essential to obtain uniform and compact zinc deposits and improve the performance of ZFBs (Chamoun et al., 2015; Li et al., 2015; Yan et al., 2015).
Nickel and carbon materials are widely used as anodes due to their good corrosion resistance and high electric conductivity (Li et al., 2015, 2018; Wang et al., 2016, 2017b; Xia et al., 2019). Under a large charging current, a rapid zinc deposition process occurs, which leads to severe zinc dendrite development on flat anodes because of their lower specific surface area (Xie et al., 2019). Cheng et al. for the first time, introduced three-dimensional porous nickel foam into zinc-nickel flow batteries (Cheng et al., 2013b). Its high specific surface area reduces the actual current density. Its three-dimensional porous structure greatly reduces the internal resistance of the interface between electrodes and electrolytes. Thus, zinc dendrite was prevented, and improved power density, energy efficiency, and cycling life were reported. This indicates that three-dimensional porous electrodes are more suitable for zinc deposition and dissolution under a high charging current.
Recently, Yin et al. chose the low-cost metal Sn as the morphology-inducing material for zinc deposition (Yin et al., 2020). Magnetron sputtering technology was used to enable Sn to be firmly deposited on carbon felt without binders (Figures 2d–f). Sn possesses stronger adsorption ability to zinc atoms than does carbon, which effectively strengthened the affiliation between the Sn nanoparticles and zinc deposits. Sn-modified carbon felt thus affords more robust zinc nucleation sites and induces compact and uniform zinc deposition. The Cycling life and coulombic efficiency of zinc-bromine flow batteries were significantly improved.
Electric Field Regulation
The electric field drives zinc nucleation on anodes and the transfer of zinc ions to the interface between anodes and electrolytes. The electric field can be controlled by the charging current (Cheng et al., 2014a; Desai et al., 2014; Nikiforidis et al., 2014; Song et al., 2014), a charged separator (Yuan et al., 2018b), and a pulsed charging model (Wang et al., 2015, 2017a; Zelger et al., 2016; Garcia et al., 2017; Pichler et al., 2017; Yang et al., 2019).
A charged separator provides an effective way to solve zinc dendrite development in ZFBs. As shown in Figures 2g–i, Yuan et al. designed a porous membrane with negative charges on the pore wall and surface (Yuan et al., 2018b). The negatively charged zincate ions and the negatively charged porous membrane repel each other. Therefore, zinc ions can be deposited easily along the direction of the separator to the 3D carbon felt frame. A ZFB using a negatively charged membrane has no short circuit in about 240 cycles at current densities of 80 to 160 mA/cm2 and exhibits stable performance.
Ito et al. studied the effect of charging currents on zinc morphology in flowing alkaline electrolytes (Ito et al., 2012). The ratio of the effective current density to the limiting current density (current density ratio) is directly related to the zincate concentration on the interface and determines the morphology of zinc deposits. When the current density ratio is <0.4, the zinc morphology is mossy and porous. When the current density ratio is between 0.4 and 0.9, it has a mixture of a mossy and crystal structure. Only when the current density ratio is higher than 0.9 will the zinc deposits become crystalline and dense.
The charging module can be designed and operated to inhibit zinc dendrite (Wang et al., 2015; Pichler et al., 2017, 2018; Yang et al., 2019). The Taguchi method was utilized to optimize the values of current density, duty cycle, and pulse frequency. As the nucleation is mainly determined by overpotentials and zinc ion distribution on anodes, large overpotentials can produce more zinc seeds. Interestingly, pulsed current or voltage provides more time for zinc transfer to reactive interfaces. This will be prone to form compact and uniform zinc deposits and prevent zinc dendrite development in zinc-air flow batteries (Yang et al., 2019).
Zinc Ion Transfer Control
Zinc ion transfer plays an important role in the growth of zinc on the nucleus. A uniform distribution of zinc ions will result in the same rate of zinc growth on anodes (Nikiforidis et al., 2014; Song et al., 2014). However, the concentration gradient of zinc ions may be different along the interfaces due to non-uniform zinc seeding. Accurate regulation of zinc ion transfer is needed. Currently, controlling the flow rate of electrolyte and adding extra magnetic field are two typical methods for achieving this (Shi et al., 2013; Wang et al., 2017a, 2018a,b).
Flowing electrolyte can change the mass transfer of zinc ions from diffusion control in static electrolytes to convection control (Wang et al., 2014). As shown in Figures 2j–l, the larger the flow velocity is, the greater the zinc concentration gradient is in the interfaces. The concentration gradient is the main driving force for zinc ion transfer. A large concentration gradient can ensure the timely delivery of reactants for the nucleation and growth process of zinc deposition. Therefore, zinc dendrites appear under quiescent electrolyte, while uniform and compact zinc deposits are obtained in flowing electrolyte (Wang et al., 2014).
Ito et al. also found that when the flow rate of the electrolyte is higher than 15 cm/s, the growth of zinc dendrites is deformed in the direction of the electrolyte flow, thereby avoiding short circuit of the battery. A zinc-nickel system with a 100 Wh battery was scaled up to evaluate the influence of zinc ion transfer on zinc morphology and battery performance. This system had a long cycling life of more than 200 cycles (Ito et al., 2011b).
A magnetic field can affect the movement of zinc ions (Wang et al., 2018a). The magnet is placed on the anode side to design an additional driving force for zinc ion transfer. The magnetic field accelerates zinc ion transfer and suppresses the dendritic growth of zinc deposits. As a result, the cycling life of batteries is improved.
Summary and Outlook
Zinc anodes are usually used in aqueous electrolytes, enabling zinc-based batteries with high safety and low cost. Flowing electrolyte can enhance mass transfer and reduce concentration polarization. ZFBs have therefore been investigated widely and show prospects for practical application. The issue of zinc dendrite formation has been extensively studied since its emergence. Some effective strategies for inhibiting zinc dendrite development in ZFBs have been proposed, including electrolyte modification, anode engineering, electric field regulation, and ion transfer control. Although great progress has been made in the field of zinc dendrites, many methods are used in isolation, with strict working conditions, and costly implementation. Here, remaining challenges and promising directions for the inhibition of zinc dendrite formation in ZFBs are outlined and anticipated.
i) The forms of zinc ions existing in aqueous solution are very complex. Zinc ions can combine with different amounts of water and other anions, which has a significant impact on the nucleation and growth processes of zinc deposition.
ii) Zinc morphology depends strongly on specific operating conditions, such as the charging model, current density, flow rate, zinc ion concentration, and temperature. Most current studies only investigate one or two variables, idly fixing other parameters. It is necessary to systematically investigate the relationship between zinc morphologies and operating conditions. The theoretical basis of our understanding of zinc deposition needs to be enriched.
iii) The capacity and thickness of zinc deposits in ZFBs is much larger than that of lithium deposits in lithium batteries. The zinc deposits in ZFBs are expected to have a specific capacity of more than 100 mAh/cm−2 and to be thicker than 170 μm. The thicker the zinc deposit is, the more difficult it is to control its morphology. Therefore, great efforts are required to concentrate on the inhibition of zinc dendrites under large capacity or thick deposits.
iv) In static zinc batteries, brighteners, pretreatment of zinc anodes, and new electrolytes have made significant progress toward achieving a uniform zinc electroplating/electrostripping process, which may enable flow battery researchers to look into more possibilities in further work.
In short, we look forward to a better solution to the zinc dendrite problem with a view to achieving a long cycling life and high safety and eventually improving the competitiveness of ZFBs.
Author Contributions
All authors listed have made a substantial, direct and intellectual contribution to the work, and approved it for publication.
Funding
This work was supported by the National Key Research and Development Program of China (2019YFA0210300), Distinguished Scientist Program at BUCT; Fundamental Research Funds for the Central Universities (buctrc201524), and BUCT Fund for Disciplines Construction and Development (XK1502).
Conflict of Interest
The authors declare that the research was conducted in the absence of any commercial or financial relationships that could be construed as a potential conflict of interest.
References
Arenas, L. F., Loh, A., Trudgeon, D. P., Li, X., Ponce de León, C., and Walsh, F. C. (2018). The characteristics and performance of hybrid redox flow batteries with zinc negative electrodes for energy storage. Renew. Sust. Energ. Rev. 90, 992–1016. doi: 10.1016/j.rser.2018.03.016
Ballesteros, J. C., Díaz-Arista, P., Meas, Y., Ortega, R., and Trejo, G. (2007). Zinc electrodeposition in the presence of polyethylene glycol 20000. Electrochim. Acta 52, 3686–3696. doi: 10.1016/j.electacta.2006.10.042
Banik, S. J., and Akolkar, R. (2013). Suppressing dendrite growth during zinc electrodeposition by PEG-200 additive. J. Electrochem. Soc. 160, D519–D523. doi: 10.1149/2.040311jes
Banik, S. J., and Akolkar, R. (2015). Suppressing dendritic growth during alkaline zinc electrodeposition using polyethylenimine additive. Electrochim. Acta 179, 475–481. doi: 10.1016/j.electacta.2014.12.100
Chamoun, M., Hertzberg, B. J., Gupta, T., Davies, D., Bhadra, S., Van Tassell, B., et al. (2015). Hyper-dendritic nanoporous zinc foam anodes. NPG Asia Mater. 7:8. doi: 10.1038/am.2015.32
Chang, S., Ye, J., Zhou, W., Wu, C., Ding, M., Long, Y., et al. (2019). A low-cost SPEEK-K type membrane for neutral aqueous zinc-iron redox flow battery. Surf. Coat. Technol. 358, 190–194. doi: 10.1016/j.surfcoat.2018.11.028
Cheng, Y., Ke, X., Chen, Y., Huang, X., Shi, Z., and Guo, Z. (2019a). Lithiophobic-lithiophilic composite architecture through co-deposition technology toward high-performance lithium metal batteries. Nano Energy 63:103854. doi: 10.1016/j.nanoen.2019.103854
Cheng, Y., Lai, Q., Li, X., Xi, X., Zheng, Q., Ding, C., et al. (2014a). Zinc-nickel single flow batteries with improved cycling stability by eliminating zinc accumulation on the negative electrode. Electrochim. Acta 145, 109–115. doi: 10.1016/j.electacta.2014.08.090
Cheng, Y., Li, D., Shi, L., and Xiang, Z. (2018). Efficient unitary oxygen electrode for air-based flow batteries. Nano Energy 47, 361–367. doi: 10.1016/j.nanoen.2018.03.013
Cheng, Y., Wang, Y., Wang, Q., Liao, Z., Zhang, N., Guo, Y., et al. (2019b). Hierarchically porous metal-free carbon with record high mass activity for oxygen reduction and Zn-air batteries. J. Mater. Chem. A 7, 9831–9836. doi: 10.1039/C9TA02220A
Cheng, Y., Xi, X., Li, D., Li, X., Lai, Q., and Zhang, H. (2015). Performance and potential problems of high power density zinc-nickel single flow batteries. RSC Adv. 5, 1772–1776. doi: 10.1039/C4RA12812E
Cheng, Y., Zhang, H., Lai, Q., Li, X., and Shi, D. (2013a). Performance gains in single flow zinc-nickel batteries through novel cell configuration. Electrochim. Acta 105, 618–621. doi: 10.1016/j.electacta.2013.05.024
Cheng, Y., Zhang, H., Lai, Q., Li, X., Shi, D., and Zhang, L. (2013b). A high power density single flow zinc–nickel battery with three-dimensional porous negative electrode. J. Power Sources 241, 196–202. doi: 10.1016/j.jpowsour.2013.04.121
Cheng, Y., Zhang, H., Lai, Q., Li, X., Zheng, Q., Xi, X., et al. (2014b). Effect of temperature on the performances and in situ polarization analysis of zinc-nickel single flow batteries. J. Power Sources 249, 435–439. doi: 10.1016/j.jpowsour.2013.10.115
Cheng, Y., Zhang, N., Wang, Q., Guo, Y., Tao, S., Liao, Z., et al. (2019c). A long-life hybrid zinc flow battery achieved by dual redox couples at cathode. Nano Energy 63:103822. doi: 10.1016/j.nanoen.2019.06.018
Chladil, L., Cech, O., Smejkal, J., and Vanýsek, P. (2019). Study of zinc deposited in the presence of organic additives for zinc-based secondary batteries. J Energy Storage 21, 295–300. doi: 10.1016/j.est.2018.12.001
Desai, D., Wei, X., Steingart, D. A., and Banerjee, S. (2014). Electrodeposition of preferentially oriented zinc for flow-assisted alkaline batteries. J. Power Sources 256, 145–152. doi: 10.1016/j.jpowsour.2014.01.026
Dundalek, J., Snajdr, I., Libansky, O., Vrana, J., Pocedic, J., Mazur, P., et al. (2017). Zinc electrodeposition from flowing alkaline zincate solutions: role of hydrogen evolution reaction. J. Power Sources 372, 221–226. doi: 10.1016/j.jpowsour.2017.10.077
Dunn, B., Kamath, H., and Tarascon, J.-M. (2011). Electrical energy storage for the grid: a battery of choices. Science 334, 928–935. doi: 10.1126/science.1212741
Garcia, G., Ventosa, E., and Schuhmann, W. (2017). Complete prevention of dendrite formation in Zn metal anodes by means of pulsed charging protocols. ACS Appl. Mater. Interfaces 9, 18691–18698. doi: 10.1021/acsami.7b01705
Goh, F. W. T., Liu, Z. L., Hor, T. S. A., Zhang, J., Ge, X. M., Zong, Y., et al. (2014). A near-neutral chloride electrolyte for electrically rechargeable zinc-air batteries. J. Electrochem. Soc. 161, A2080–A2086. doi: 10.1149/2.0311414jes
Hashemi, A. B., Kasiri, G., and La Mantia, F. (2017). The effect of polyethyleneimine as an electrolyte additive on zinc electrodeposition mechanism in aqueous zinc-ion batteries. Electrochim. Acta 258, 703–708. doi: 10.1016/j.electacta.2017.11.116
Hosseini, S., Abbasi, A., Uginet, L.-O., Haustraete, N., Praserthdam, S., Yonezawa, T., et al. (2019). The influence of dimethyl sulfoxide as electrolyte additive on anodic dissolution of alkaline zinc-air flow battery. Sci. Rep. 9:14958. doi: 10.1038/s41598-019-51412-5
Hosseini, S., Lao-Atiman, W., Han, S. J., Arpornwichanop, A., Yonezawa, T., and Kheawhom, S. (2018). Discharge performance of zinc-air flow batteries under the effects of sodium dodecyl sulfate and pluronic F-127. Sci. Rep. 8:13. doi: 10.1038/s41598-018-32806-3
Huang, H., Guo, Y., and Cheng, Y. (2018). Ultrastable alpha phase nickel hydroxide as energy storage materials for alkaline secondary batteries. Appl. Surf. Sci. 435, 635–640. doi: 10.1016/j.apsusc.2017.11.156
Ito, Y., Nyce, M., Plivelich, R., Klein, M., and Banerjee, S. (2011a). Gas evolution in a flow-assisted zinc–nickel oxide battery. J. Power Sources 196, 6583–6587. doi: 10.1016/j.jpowsour.2011.03.025
Ito, Y., Nyce, M., Plivelich, R., Klein, M., Steingart, D., and Banerjee, S. (2011b). Zinc morphology in zinc–nickel flow assisted batteries and impact on performance. J. Power Sources 196, 2340–2345. doi: 10.1016/j.jpowsour.2010.09.065
Ito, Y., Wei, X., Desai, D., Steingart, D., and Banerjee, S. (2012). An indicator of zinc morphology transition in flowing alkaline electrolyte. J. Power Sources 211, 119–128. doi: 10.1016/j.jpowsour.2012.03.056
Jeon, J.-D., Yang, H. S., Shim, J., Kim, H. S., and Yang, J. H. (2014). Dual function of quaternary ammonium in Zn/Br redox flow battery: capturing the bromine and lowering the charge transfer resistance. Electrochim. Acta 127, 397–402. doi: 10.1016/j.electacta.2014.02.073
Jiang, H. R., Wu, M. C., Ren, Y. X., Shyy, W., and Zhao, T. S. (2018). Towards a uniform distribution of zinc in the negative electrode for zinc bromine flow batteries. Appl. Energy 213, 366–374. doi: 10.1016/j.apenergy.2018.01.061
Justinijanović, I. N., Jovićević, J. N., and Despić, A. R. (1973). The effect of foreign atoms on the properties of electrolytic zinc powders. J. Appl. Electrochem. 3, 193–200. doi: 10.1007/BF00619161
Kan, J., Xue, H., and Mu, S. (1998). Effect of inhibitors on Zn-dendrite formation for zinc-polyaniline secondary battery. J. Power Sources 74, 113–116. doi: 10.1016/S0378-7753(98)00040-8
Kang, L. T., Cui, M. W., Jiang, F. Y., Gao, Y. F., Luo, H. J., Liu, J. J., et al. (2018). Nanoporous CaCO3 coatings enabled uniform Zn stripping/plating for long-life zinc rechargeable aqueous batteries. Adv. Energy Mater. 8:8. doi: 10.1002/aenm.201801090
Khor, A., Leung, P., Mohamed, M. R., Flox, C., Xu, Q., An, L., et al. (2018). Review of zinc-based hybrid flow batteries: from fundamentals to applications. Mater. Today Energy 8, 80–108. doi: 10.1016/j.mtener.2017.12.012
Kim, H.-I., and Shin, H.-C. (2015). SnO additive for dendritic growth suppression of electrolytic zinc. J. Alloys Compd. 645, 7–10. doi: 10.1016/j.jallcom.2015.04.208
Kim, M., Yun, D., and Jeon, J. (2019). Effect of a bromine complex agent on electrochemical performances of zinc electrodeposition and electrodissolution in Zinc–Bromide flow battery. J. Power Sources 438:227020. doi: 10.1016/j.jpowsour.2019.227020
Lacitignola, D., Bozzini, B., Frittelli, M., and Sgura, I. (2017). Turing pattern formation on the sphere for a morphochemical reaction-diffusion model for electrodeposition. Commun. Nonlinear Sci. Numer. Simul. 48, 484–508. doi: 10.1016/j.cnsns.2017.01.008
Leadbetter, J., and Swan, L. G. (2012). Selection of battery technology to support grid-integrated renewable electricity. J. Power Sources 216, 376–386. doi: 10.1016/j.jpowsour.2012.05.081
Lee, C. W., Sathiyanarayanan, K., Eom, S. W., Kim, H. S., and Yun, M. S. (2006a). Novel electrochemical behavior of zinc anodes in zinc/air batteries in the presence of additives. J. Power Sources 159, 1474–1477. doi: 10.1016/j.jpowsour.2005.11.074
Lee, C. W., Sathiyanarayanan, K., Eom, S. W., and Yun, M. S. (2006b). Novel alloys to improve the electrochemical behavior of zinc anodes for zinc/air battery. J. Power Sources 160, 1436–1441. doi: 10.1016/j.jpowsour.2006.02.019
Leung, P. K., Ponce-de-Leon, C., Low, C. T. J., and Walsh, F. C. (2011). Zinc deposition and dissolution in methanesulfonic acid onto a carbon composite electrode as the negative electrode reactions in a hybrid redox flow battery. Electrochim. Acta 56, 6536–6546. doi: 10.1016/j.electacta.2011.04.111
Li, H. F., Xu, C. J., Han, C. P., Chen, Y. Y., Wei, C. G., Li, B. H., et al. (2015). Enhancement on cycle performance of Zn anodes by activated carbon modification for neutral rechargeable zinc ion batteries. J. Electrochem. Soc. 162, A1439–A1444. doi: 10.1149/2.0141508jes
Li, M., Meng, J. S., Li, Q., Huang, M., Liu, X., Owusu, K. A., et al. (2018). Finely crafted 3D electrodes for dendrite-free and high-performance flexible fiber-shaped Zn-Co batteries. Adv. Funct. Mater. 28:10. doi: 10.1002/adfm.201802016
Lin, H. (2018). Pyrolytic carbon felt electrode inhibits formation of zinc dendrites in zinc bromine flow batteries. Int. J. Electrochem. Sci. 13, 12049–12061. doi: 10.20964/2018.12.18
Liu, N. N., Mohanapriya, K., Pan, J., Hu, Y., Sun, Y. Z., and Liu, X. G. (2020). A facile preparation of lambda-MnO2 as cathode material for high-performance zinc-manganese redox flow battery. J. Electrochem. Soc. 167:8. doi: 10.1149/1945-7111/ab75c2
Liu, W., Lu, W., Zhang, H., and Li, X. (2019a). Aqueous flow batteries: research and development. Chem. Eur. J. 25, 1649–1664. doi: 10.1002/chem.201802798
Liu, X. Y., Bolton, O., and Akolkar, R. (2019b). Experimental and modeling studies of the hysteresis behavior and dendrite suppression efficacy of an electrolyte additive in zinc electrodeposition. J. Electrochem. Soc. 166, D583–D588. doi: 10.1149/2.0411913jes
Lou, X., Yuan, D., Yu, Y., Lei, Y., Ding, M., Sun, Q., et al. (2020). A cost-effective nafion composite membrane as an effective vanadium-ion barrier for vanadium redox flow batteries. Chem. Asi. J. 607:118177. doi: 10.1002/asia.202000140
Lu, W., Xie, C., Zhang, H., and Li, X. (2018). Inhibition of zinc dendrite growth in zinc-based batteries. ChemSusChem 11, 3996–4006. doi: 10.1002/cssc.201801657
Mitha, A., Yazdi, A. Z., Ahmed, M., and Chen, P. (2018). Surface adsorption of polyethylene glycol to suppress dendrite formation on zinc anodes in rechargeable aqueous batteries. ChemElectroChem 5, 2409–2418. doi: 10.1002/celc.201800572
Miyazaki, K., Nakata, A., Lee, Y. S., Fukutsuka, T., and Abe, T. (2016). Influence of surfactants as additives to electrolyte solutions on zinc electrodeposition and potential oscillation behavior. J. Appl. Electrochem. 46, 1067–1073. doi: 10.1007/s10800-016-0987-4
Nikiforidis, G., Cartwright, R., Hodgson, D., Hall, D., and Berlouis, L. (2014). Factors affecting the performance of the Zn-Ce redox flow battery. Electrochim. Acta 140, 139–144. doi: 10.1016/j.electacta.2014.04.150
Nikiforidis, G., and Daoud, W. A. (2015). Indium modified graphite electrodes on highly zinc containing methanesulfonate electrolyte for zinc-cerium redox flow battery. Electrochim. Acta 168, 394–402. doi: 10.1016/j.electacta.2015.03.118
Ortiz-Aparicio, J. L., Meas, Y., Trejo, G., Ortega, R., Chapman, T. W., and Chainet, E. (2013). Effects of organic additives on zinc electrodeposition from alkaline electrolytes. J. Appl. Electrochem. 43, 289–300. doi: 10.1007/s10800-012-0518-x
Parker, J. F., Chervin, C. N., Pala, I. R., Machler, M., Burz, M. F., Long, J. W., et al. (2017). Rechargeable nickel-3D zinc batteries: an energy-dense, safer alternative to lithium-ion. Science 356, 414–417. doi: 10.1126/science.aak9991
Pei, A., Zheng, G., Shi, F., Li, Y., and Cui, Y. (2017). Nanoscale Nucleation and Growth of Electrodeposited Lithium Metal. Nano Lett 17, 1132–1139. doi: 10.1021/acs.nanolett.6b04755
Pichler, B., Berner, B. S., Rauch, N., Zelger, C., Pauling, H.-J., Gollas, B., et al. (2018). The impact of operating conditions on component and electrode development for zinc-air flow batteries. J. Appl. Electrochem. 48, 1043–1056. doi: 10.1007/s10800-018-1233-z
Pichler, B., Weinberger, S., Rescec, L., Grimmer, I., Gebetsroither, F., Bitschnau, B., et al. (2017). Bifunctional electrode performance for zinc-air flow cells with pulse charging. Electrochim. Acta 251, 488–497. doi: 10.1016/j.electacta.2017.08.128
Rossi, F., Mele, C., Boniardi, M., and Bozzini, B. (2020). Electrodeposition of zinc from alkaline electrolytes containing quaternary ammonium salts and ionomers: impact of cathodic-anodic cycling conditions. Chemelectrochem 7, 1752–1764. doi: 10.1002/celc.202000165
Shen, C., Li, X., Li, N., Xie, K. Y., Wang, J. G., Liu, X. R., et al. (2018). Graphene-boosted, high-performance aqueous Zn-Ion battery. ACS Appl. Mater. Interfaces 10, 25446–25453. doi: 10.1021/acsami.8b07781
Shi, J., Xu, H., Lu, L., and Sun, X. (2013). Study of magnetic field to promote oxygen transfer and its application in zinc–air fuel cells. Electrochim. Acta 90, 44–52. doi: 10.1016/j.electacta.2012.11.088
Shimizu, M., Hirahara, K., and Arai, S. (2019). Morphology control of zinc electrodeposition by surfactant addition for alkaline-based rechargeable batteries. PCCP 21, 7045–7052. doi: 10.1039/C9CP00223E
Song, S., Pan, J., Wen, Y., Cheng, J., Pan, J., and Cao, G. (2014). Effects of electrolyte flow speed on the performance of Zn-Ni single flow batteries. Chem. J. Chin. Univ.-Chin. 35, 134–139. doi: 10.7503/cjcu20130340
Song, Y. X., Hu, J. H., Tang, J., Gu, W. M., He, L. L., and Ji, X. B. (2016). Real-time X-ray imaging reveals interfacial growth, suppression, and dissolution of zinc dendrites dependent on anions of ionic liquid additives for rechargeable battery applications. ACS Appl. Mater. Interfaces 8, 32031–32040. doi: 10.1021/acsami.6b11098
Sun, K. E. K., Hoang, T. K. A., Doan, T. N. L., Zhu, Y. Y. X., Tian, Y., and Chen, P. (2017). Suppression of dendrite formation and corrosion on zinc anode of secondary aqueous batteries. ACS Appl. Mater. Interfaces 9, 9681–9687. doi: 10.1021/acsami.6b16560
Suresh, S., Kesavan, T., Munaiah, Y., Arulraj, I., Dheenadayalan, S., and Ragupathy, P. (2014). Zinc-bromine hybrid flow battery: effect of zinc utilization and performance characteristics. RSC Adv. 4, 37947–37953. doi: 10.1039/C4RA05946H
Suresh, S., Ulaganathan, M., and Pitchai, R. (2019). Realizing highly efficient energy retention of Zn–Br2 redox flow battery using rGO supported 3D carbon network as a superior electrode. J. Power Sources 438:226998. doi: 10.1016/j.jpowsour.2019.226998
Turner, J. A. (1999). A realizable renewable energy future. Science 285, 687–689. doi: 10.1126/science.285.5428.687
Wang, J. M., Zhang, L., Zhang, C., and Zhang, J. Q. (2001). Effects of bismuth ion and tetrabutylammonium bromide on the dendritic growth of zinc in alkaline zincate solutions. J. Power Sources 102, 139–143. doi: 10.1016/S0378-7753(01)00789-3
Wang, K., Liu, X., Pei, P., Xiao, Y., and Wang, Y. (2018a). Guiding bubble motion of rechargeable zinc-air battery with electromagnetic force. Chem. Eng. J. 352, 182–187. doi: 10.1016/j.cej.2018.07.020
Wang, K., Pei, P., Ma, Z., Chen, H., Xu, H., Chen, D., et al. (2015). Dendrite growth in the recharging process of zinc-air batteries. J. Mater. Chem. A 3, 22648–22655. doi: 10.1039/C5TA06366C
Wang, K., Pei, P., Ma, Z., Xu, H., Li, P., and Wang, X. (2014). Morphology control of zinc regeneration for zinc-air fuel cell and battery. J. Power Sources 271, 65–75. doi: 10.1016/j.jpowsour.2014.07.182
Wang, K., Pei, P., and Wang, Y. (2017a). Magnetic field improving interfacial behavior of the two-electrode system. J. Electrochem. Soc. 164, A3440–A3444. doi: 10.1149/2.0031714jes
Wang, K., Pei, P., Wang, Y., Liao, C., WAng, W., and Huang, S. (2018b). Advanced rechargeable zinc-air battery with parameter optimization. Appl. Energy 225, 848–856. doi: 10.1016/j.apenergy.2018.05.071
Wang, L. P., Li, N. W., Wang, T. S., Yin, Y. X., Guo, Y. G., and Wang, C. R. (2017b). Conductive graphite fiber as a stable host for zinc metal anodes. Electrochim. Acta 244, 172–177. doi: 10.1016/j.electacta.2017.05.072
Wang, X. W., Wang, F. X., Wang, L. Y., Li, M. X., Wang, Y. F., Chen, B. W., et al. (2016). An aqueous rechargeable Zn//Co3O4 battery with high energy density and good cycling behavior. Adv. Mater. 28, 4904–4911. doi: 10.1002/adma.201505370
Wei, X., Desai, D., Yadav, G. G., Turney, D. E., Couzis, A., and Banerjee, S. (2016). Impact of anode substrates on electrodeposited zinc over cycling in zinc-anode rechargeable alkaline batteries. Electrochim. Acta 212, 603–613. doi: 10.1016/j.electacta.2016.07.041
Wen, Y., Wang, T., Cheng, J., Pan, J., Cao, G., and Yang, Y. (2012). Lead ion and tetrabutylammonium bromide as inhibitors of the growth of spongy zinc in single flow zinc/nickel batteries. Electrochim. Acta 59, 64–68. doi: 10.1016/j.electacta.2011.10.042
Xia, L., Zhang, Q., Wu, C., Liu, Y., Ding, M., Ye, J., et al. (2019). Graphene coated carbon felt as a high-performance electrode for all vanadium redox flow batteries. Surf. Coat. Technol. 358, 153–158. doi: 10.1016/j.surfcoat.2018.11.024
Xie, C., Liu, Y., Lu, W., Zhang, H., and Li, X. (2019). Highly stable zinc–iodine single flow batteries with super high energy density for stationary energy storage. Energy Environ. Sci. 12, 1834–1839. doi: 10.1039/C8EE02825G
Xu, W. N., Zhao, K. N., Huo, W. C., Wang, Y. Z., Yao, G., Gu, X., et al. (2019). Diethyl ether as self-healing electrolyte additive enabled long-life rechargeable aqueous zinc ion batteries. Nano Energy 62, 275–281. doi: 10.1016/j.nanoen.2019.05.042
Yan, Z., Wang, E. D., Jiang, L. H., and Sun, G. Q. (2015). Superior cycling stability and high rate capability of three-dimensional Zn/Cu foam electrodes for zinc-based alkaline batteries. RSC Adv. 5, 83781–83787. doi: 10.1039/C5RA16264E
Yang, H. B., Meng, X. L., Yang, E. D., Wang, X. D., and Zhou, Z. X. (2004). Effect of La addition on the electrochemical properties of secondary zinc electrodes. J. Electrochem. Soc. 151, A389–A393. doi: 10.1149/1.1646407
Yang, T.-F., Lu, J.-H., Yan, W.-M., and Ghalambaz, M. (2019). Optimization of pulse current on energy storage of zinc-air flow batteries. J. Power Sources 442:227253. doi: 10.1016/j.jpowsour.2019.227253
Yang, Z., Zhang, J., Kintner-Meyer, M. C. W., Lu, X., Choi, D., Lemmon, J. P., et al. (2011). Electrochemical energy storage for green grid. Chem. Rev. 111, 3577–3613. doi: 10.1021/cr100290v
Yao, S., Chen, Y., Cheng, J., Shen, Y., and Ding, D. (2019). Effect of stannum ion on the enhancement of the charge retention of single-flow zinc–nickel battery. J. Electrochem. Soc. 166, A1813–A1818. doi: 10.1149/2.0311910jes
Ye, J., Wu, C., Qin, W., Zhong, F., and Ding, M. (2020a). Advanced sulfonated poly(Ether Ether Ketone)/Graphene-Oxide/Titanium dioxide nanoparticle composited membrane with superior cyclability for vanadium redox flow battery. J. Nanosci. Nanotechnol. 20, 4714–4721. doi: 10.1166/jnn.2020.18503
Ye, J., Zhao, X., Ma, Y., Su, J., Xiang, C., Zhao, K., et al. (2020b). Hybrid membranes dispersed with superhydrophilic TiO2 nanotubes toward ultra-stable and high-performance vanadium redox flow batteries. Adv. Energy Mater. 10:1904041. doi: 10.1002/aenm.201904041
Yin, Y., Wang, S., Zhang, Q., Song, Y., Chang, N., Pan, Y., et al. (2020). Dendrite-free zinc deposition induced by tin-modified multifunctional 3D host for stable zinc-based flow battery. Adv Mater 32:e1906803. doi: 10.1002/adma.201906803
Yuan, Y. F., Tu, J. P., Wu, H. M., Wang, S. F., Zhang, W. K., and Huang, H. (2007). Effects of stannous ions on the electrochemical performance of the alkaline zinc electrode. J. Appl. Electrochem. 37, 249–253. doi: 10.1007/s10800-006-9249-1
Yuan, Z., Duan, Y., Liu, T., Zhang, H., and Li, X. (2018a). Toward a low-cost alkaline zinc-iron flow battery with a polybenzimidazole custom membrane for stationary energy storage. Science 3, 40–49. doi: 10.1016/j.isci.2018.04.006
Yuan, Z., Liu, X., Xu, W., Duan, Y., Zhang, H., and Li, X. (2018b). Negatively charged nanoporous membrane for a dendrite-free alkaline zinc-based flow battery with long cycle life. Nat Commun 9:3731. doi: 10.1038/s41467-018-06209-x
Yufit, V., Tariq, F., Eastwood, D. S., Biton, M., Wu, B., Lee, P. D., et al. (2019). Operando visualization and multi-scale tomography studies of dendrite formation and dissolution in zinc batteries. Joule 3, 485–502. doi: 10.1016/j.joule.2018.11.002
Zelger, C., Laumen, J., Laskos, A., and Gollas, B. (2016). Rota-hull cell study on pulse current zinc electrodeposition from alkaline electrolytes. Electrochim. Acta 213, 208–216. doi: 10.1016/j.electacta.2016.07.108
Zeng, Y., Zhang, X., Qin, R., Liu, X., Fang, P., Zheng, D., et al. (2019). Dendrite-free zinc deposition induced by multifunctional CNT frameworks for stable flexible Zn-Ion batteries. Adv. Mater. 31:1903675. doi: 10.1002/adma.201903675
Zhang, H., Lu, W., and Li, X. (2019a). Progress and perspectives of flow battery technologies. Electrochem. Energy Rev. 2, 492–506. doi: 10.1007/s41918-019-00047-1
Zhang, L., Cheng, J., Yang, Y. S., Wen, Y. H., Wang, X. D., and Cao, G. P. (2008). Study of zinc electrodes for single flow zinc/nickel battery application. J. Power Sources 179, 381–387. doi: 10.1016/j.jpowsour.2007.12.088
Zhang, Q., Luan, J., Fu, L., Wu, S., Tang, Y., Ji, X., et al. (2019b). The Three-Dimensional Dendrite-Free Zinc Anode On A Copper Mesh With A Zinc-Oriented Polyacrylamide Electrolyte Additive. Angew. Chem. Int. Ed. 58, 15841–15847. doi: 10.1002/anie.201907830
Zhang, Q., Luan, J., Tang, Y., Ji, X., and Wang, H. Y. (2020). Interfacial design of dendrite-free zinc anodes for aqueous zinc-ion batteries. Angew. Chem. Int. Ed. Engl. 59, 2–14. doi: 10.1002/anie.202000162
Keywords: flow battery, zinc deposition, zinc dendrites, interfaces engineering, energy storage and conversion, rechargeable battery
Citation: Guo L, Guo H, Huang H, Tao S and Cheng Y (2020) Inhibition of Zinc Dendrites in Zinc-Based Flow Batteries. Front. Chem. 8:557. doi: 10.3389/fchem.2020.00557
Received: 30 April 2020; Accepted: 02 June 2020;
Published: 24 July 2020.
Edited by:
Chuankun Jia, Changsha University of Science and Technology, ChinaReviewed by:
Ao Tang, Institute of Metals Research (CAS), ChinaHui Chen, Yancheng Institute of Technology, China
Copyright © 2020 Guo, Guo, Huang, Tao and Cheng. This is an open-access article distributed under the terms of the Creative Commons Attribution License (CC BY). The use, distribution or reproduction in other forums is permitted, provided the original author(s) and the copyright owner(s) are credited and that the original publication in this journal is cited, in accordance with accepted academic practice. No use, distribution or reproduction is permitted which does not comply with these terms.
*Correspondence: Haili Huang, aGxodWFuZyYjeDAwMDQwO21haWwuYnVjdC5lZHUuY24=; Yuanhui Cheng, Y2hlbmd5aCYjeDAwMDQwO21haWwuYnVjdC5lZHUuY24=