- College of Energy and Power, Jiangsu University of Science and Technology, Zhenjiang, China
Magnesium hydride (MgH2) has attracted intense attention worldwide as solid state hydrogen storage materials due to its advantages of high hydrogen capacity, good reversibility, and low cost. However, high thermodynamic stability and slow kinetics of MgH2 has limited its practical application. We reviewed the recent development in improving the sorption kinetics of MgH2 and discovered that transition metals and their alloys have been extensively researched to enhance the de/hydrogenation performance of MgH2. In addition, to maintain the cycling property during the de/hydrogenation process, carbon materials (graphene, carbon nanotubes, and other materials) have been proved to possess excellent effect. In this work, we introduce various categories of transition metals and their alloys to MgH2, focusing on their catalytic effect on improving the hydrogen de/absorption performance of MgH2. Besides, carbon materials together with transition metals and their alloys are also summarized in this study, which show better hydrogen storage performance. Finally, the existing problems and challenges of MgH2 as practical hydrogen storage materials are analyzed and possible solutions are also proposed.
Introduction
Since the industrial revolution, human society is developing rapidly with continuous improvement in technology and rising demand for energy consumption (Pudukudy et al., 2014; He et al., 2016). Unfortunately, fossil fuels, which play dominate role in promoting the development of world, are not renewable and going to be running out in near future. Besides, the severe environmental problems caused by the excessive exploitation and use of fossil fuels, such as the greenhouse effect, ozone layer depletion, acid rains, and pollution, are damaging and threating the ecological balance of the earth. To mitigate the degradation of the earth, various measures have been taken by scientists to explore renewable and clean alternatives to fossil fuels.
Hydrogen, with its safe, high energy density (142 KJ/kg), environment friendliness, convenient and renewability, is proved to be the most promising sustainable and clean energy to replace fossil energy (Cao et al., 2016; Wan et al., 2020). As an energy carrier, hydrogen is abundant on earth and can be produced from any primary energy fuel: coal, oil, nuclear, natural gas, all sorts of renewable energies, and from grid electricity. Hydrogen also has a huge calorific value of energy, which is three times higher than that of petrol (43 MJ/kg) after combustion. Moreover, the dominating combustion product of hydrogen is clean and non-toxic water. Due to above advantages, hydrogen has received extensive attention from researchers worldwide and has made a rapid progress in recent decades (Winter, 2009; Sadhasivam et al., 2017; Peter, 2018). In order to realize the practical application of hydrogen energy, three challenges need to be conquered presciently, which are hydrogen preparation, storage and application. Among which, hydrogen storage has become the bottleneck technology in the wide spread of hydrogen energy (Felderhoff et al., 2007; Yang J. et al., 2010; Pukazhselvan et al., 2012; Kim et al., 2018).
Hydrogen can be stored as cryogenic liquid, high compression gas or solid-state materials (Yu et al., 2017; Abe et al., 2019). Compared with high cost cryogenic liquid storage and dangerous high compression gas tanks, hydrogen stored in solid-state materials shows easy manipulability temperature, low working pressure (Khafidz et al., 2016; Rusman and Dahari, 2016; Razavi et al., 2019). In the past decades, oceans of materials for hydrogen storage have been investigated, including physical adsorbents (carbon and MOF), complex hydrides (LiBH4, LiNH4, NaAlH4), alloys hydrides (Mg2NiH4, TiFeH2, NaMgH3), and metal hydrides (MgH2) (Shao et al., 2015; Zhai et al., 2016; Xiao et al., 2017; Chen et al., 2019; Goto et al., 2019; He et al., 2019, 2020; Liu H. et al., 2019, 2020; Song et al., 2019; Jansa et al., 2020; Yao et al., 2020).
Among different solid-state hydrogen storage materials, magnesium hydride (MgH2) has been much discussed and holds tremendous hope for storing hydrogen (Bogdanović and Spliethoff, 1990; Norberg et al., 2011; Zhang X. L. et al., 2020). As the sixth abundant metal element in the earth's crust, magnesium is widely distributed in nature. More importantly, MgH2 has a high gravimetric capacity of 7.6 wt% (volumetric capacity of 110 g/L) and excellent reversibility. However, the practical application of MgH2 has been hindered by the high desorption temperature and poor hydrogen absorption/desorption kinetics caused by high thermal stability (ΔH = 76 kJ/mol) and kinetic barrier (Ea =160 kJ/mol) (Webb, 2015; Peng et al., 2017; Zhou et al., 2019a; Jain et al., 2020).
To overcome above challenges, alloying (Bououdina and Guo, 2002; Liao et al., 2004; Kumar et al., 2013; Xu et al., 2018; Ali et al., 2019), nanostructuring (Chen et al., 2012, 2018; Yu et al., 2014; Sterl et al., 2018), nanoconfinement (Nielsen et al., 2009; Gosalawit-Utke et al., 2011; Jeon et al., 2011; Konarova et al., 2013; He et al., 2015), and doping with catalysts (Su et al., 2016; Sun et al., 2016; Zhang et al., 2018; Pluengphon et al., 2019; Wang et al., 2019) have been adopted to enhance the hydrogen storage properties of MgH2. According to recent studies, the transition metals (Ti, Fe, Co, Ni, Mn, Nb, V, Zr, etc.) and their alloys (Shang, 2004; Yavari et al., 2005; Xie et al., 2009; Pighin et al., 2012; Zahiri et al., 2012; Wang et al., 2016) doped in MgH2 showed superior modification impacts on the hydrogen storage properties while carbon materials (graphene, carbon nanotubes, and other materials) were proved to enhance the cycling property of MgH2. In this work, we systematically review transition metals, their alloys and carbon materials as catalysts to improve the hydrogen storage properties of MgH2. In addition, the remaining problems and possible solutions are proposed and discussed.
Transition Metals and Their Alloys
On the whole, doping transition metals and their alloys into magnesium hydride has been considered as one of the most feasible methods to accelerate the sorption kinetics of MgH2. During recent years, numerous transition metals and their alloys have been developed and researched. In this paper, these catalysts are reviewed and classified, presented as monometallic catalysts, binary alloys, ternary and multicomponent alloys and the composites of alloys and carbon materials. Their catalytic effects on hydrogen storage properties of MgH2 were summarized in Table 1.
Monometallic Catalysts
Nickel (Ni)
Monometallic catalysts, especially transition metals (Ershova et al., 2008; Gasan et al., 2012; El-Eskandarany et al., 2016; Tanniru et al., 2020), have shown great catalytic impact on improving the hydrogen storage properties of MgH2. Among all the transition metals studied in recent years, nickel has been the mostly adopted catalysts for MgH2. As early as 2005, Hanada et al. (2005) mixed purchased MgH2 powder with metal Ni by ball milling to get the MgH2+nano-Ni composite. Through the thermal desorption mass spectra (TDMS), they found that the hydrogen desorption peak of the Ni doped composite decreased to 260°C, which was much lower than that of pure MgH2 (370°C). Although the superior catalytic effect of Ni nanoparticles was confirmed, other factors such as particle size and catalyst amount were also widely researched lately. Xie et al. (2009) studied the hydrogen storage kinetics of the MgH2 nanoparticles doped with different concentration of Ni nanoparticles. The DSC curves depicted that the MgH2+10 wt% nano-Ni composite could desorb 6.1 wt% hydrogen within 10 min at 250°C. The desorption rate of MgH2+nano-Ni composite increased obviously with the increasing amount of catalyst. However, the activation energy of desorption could not be further lowered when the amount of Ni exceeded a certain value by using Kissinger equation. It was concluded that the catalytic effect of Ni could further be increased by reducing the particle size of catalyst and maintaining the hydrogen storage capacity at the same time. Yang W. N. et al. (2010) investigated the size effect of Ni particles on the hydrogen desorption of MgH2. The results showed that the MgH2 mixed with only 2 at% of fine Ni particles rapidly desorbed hydrogen from 200°C and almost 6.5 wt% hydrogen could be released when the temperature rose to 340°C. Nevertheless, DSC curves showed that the peak temperature of the MgH2 + 2Ni90 mixture is around 280°C, which was only about10°C lower than those of the MgH2 + 2Ni200 and the MgH2 + 2Ni100 composites. They finally concluded that the site density of the catalyst over the MgH2 particles but not the particle size was the key factor to improve the hydrogen adsorption kinetics of MgH2 after comparing with other references.
Titanium (Ti)
In comparison with nickel, titanium has also been demonstrated as a good catalyst for MgH2. In 1999, Liang et al. (1999a) studied the catalytic mechanism of titanium mainly through XRD results. During the synthesis process of the MgH2+5at%Ti composite via mechanical milling, a very stable TiH2 phase was formed by reaction of MgH2 with Ti. Interestingly, TiH2 could be obtained after desorption, which suggests that no decomposition of TiH2 phase occurred under the mild desorption condition of 300°C. The desorption curves showed that MgH2−5at%Ti composites could desorb hydrogen completely within 1,000 s at 250°C while the ball-milled MgH2 released no hydrogen under the same conditions. A lot of work has been done by researchers to further study the de/hydrogenation kinetics and microstructure of MgH2-Ti composites. Wang et al. (2015) also prepared the MgH2-Ti composite by ball milling, and found that the initial dehydrogenation temperature of the composite to be 257°C, which was 51°C lower than that of pure MgH2. The hydrogen capacity could reach 6.18 wt% at the same time. Compared to the sluggish desorption kinetics of pure MgH2, the Ea for the MgH2-Ti sample was 103.9 kJ mol−1, about 35.8% lower than that of pure MgH2 (161.3 kJ mol−1). By analyzing the mechanism it was depicted that elemental Ti reacted with MgH2 and formed active TiH1.971 during ball-milling, which acted as active species throughout the desorption process. Shao et al. (2011) mainly researched nanostructured Ti-catalyzed MgH2 for hydrogen storage. Through EDX measurements, it was discovered that Ti covered the MgH2 surface. The DTA curve showed that decomposition of Ti-catalyzed MgH2 sample started from below 300°C, which was about 130°C lower than that of the commercial MgH2 sample. Hydrogen absorption kinetics of MgH2-Ti sample were also investigated and the dehydrogenated sample could absorb 6 mass% hydrogen in <1 h at 257°C, while the commercial MgH2 needed an absorption time of about 3 h to reach a capacity of 6 mass% even at 350°C.
Iron (Fe)
As the most common metal element in life, Fe has been widely concerned and studied in recent years. Bassetti et al. (2005) mixed different concentration values of Fe with MgH2 by ball milling to explore its catalytic effect. The Mg2FeH6 phase could be detected when the ball to powder ratio rose to 20:1. They also concluded that the optimum catalyst concentration was around 10 wt% and lower values seemed to be insufficient to avoid the presence of poorly catalyzed regions. DSC curves revealed that about 5 wt% of hydrogen could be released in 600 s at 300°C. Besides the desorption property, the cycling performance and the nano-sized Fe were further studied. Montone et al. (2012) explored the cycling properties of MgH2-Fe nanocomposite in 47 cycles at 300°C. Cycling results demonstrated that the maximum storage capacity and the rate of sorption remained stable after the first 10 cycles. They also discovered that the major effect of cycling on particle morphology was the progressive extraction of Mg from the MgO shell surrounding the powder particles. In our previous study (Zhang et al., 2019b), Fe nanosheets obtained by wet-chemical ball milling were introduced in MgH2 for the first time. The MgH2+5 wt% nano-Fe composite began to release and absorb hydrogen at 182.1 and 75°C, respectively. Moreover, the dehydrogenated composite could absorb 6 wt% H2 within just 10 min at 200°C. Cycling experiment depicted that the hydrogen capacity of MgH2+5 wt% nano-Fe composite could still maintain at about 5 wt% after 50 cycles. During the first cycle, it could been seen from microstructure pictures that the Fe nanosheets on the surface of MgH2 turned to be numerous ultrafine nanoparticles, which could provide more active sites in the following cycling. Further calculation results revealed that the addition of Fe could greatly weaken the Mg-H interaction strength, facilitating the dehydrogenation of MgH2 (Figure 1).
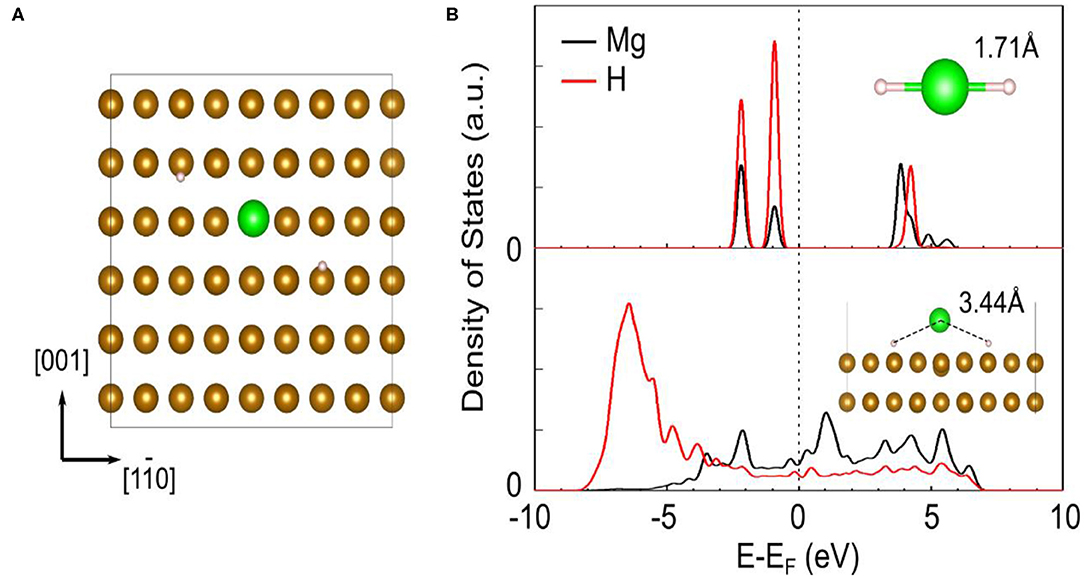
Figure 1. The adsorption geometry of MgH2 on Fe (110) surface in top view (A). The brown, green, and white ball represent Fe, Mg, and H, respectively. The projected density of states (PDOS) of Mg and H of MgH2 before (upper) and after (lower) adsorption on Fe (110) (B). The Mg-H distances are shown as inset. The vertical dash line represents the Fermi energy. Reproduced from Zhang et al. (2019b) with permission from Elsevier.
Other Monometallic Catalysts
Besides Ni, Ti, and Fe, other monometallic catalysts have also been developed to improve the hydrogen storage properties of MgH2. Cui et al. (2014) researched a series of core-shell structured Mg-TM (TM: Ti, Nb, V, Co, Mo, or Ni) nanocomposites by a wet-chemical method. The dehydrogenation performance was ranked as Mg-Ti > Mg-Nb > Mg-Ni > Mg-V > Mg-Co > Mg-Mo (Figure 2). All these composites could release hydrogen at a low temperature of 225°C, which was much lower than that of prepared MgH2. Gasan et al. (2012) studied the impacts of 5 wt% of additives (V and Nb) on the hydrogen desorption temperature of MgH2. XRD results demonstrated that the addition of V powders had a significant impact on the transformation of Mg into the MgO for the amount of MgO in MgH2-V system was higher than other systems, relevant samples were studied by others and this phenomenon needs to be further researched. Also, SEM images verified that the mean particle size of composites was decreased by mechanical milling to micro scale. DSC tests showed that the addition of 5 wt% additives reduced hydrogen desorption temperatures of MgH2 by about 40–50°C. Liang et al. (1999b) presented the hydrogen storage properties of MgH2+V composite prepared by ball milling. The MgH2+5 at% V composite could desorb hydrogen at 200°C and reabsorb hydrogen rapidly even at room temperature, the activation energy of hydrogen desorption was decreased to 62 kJ mol−1.
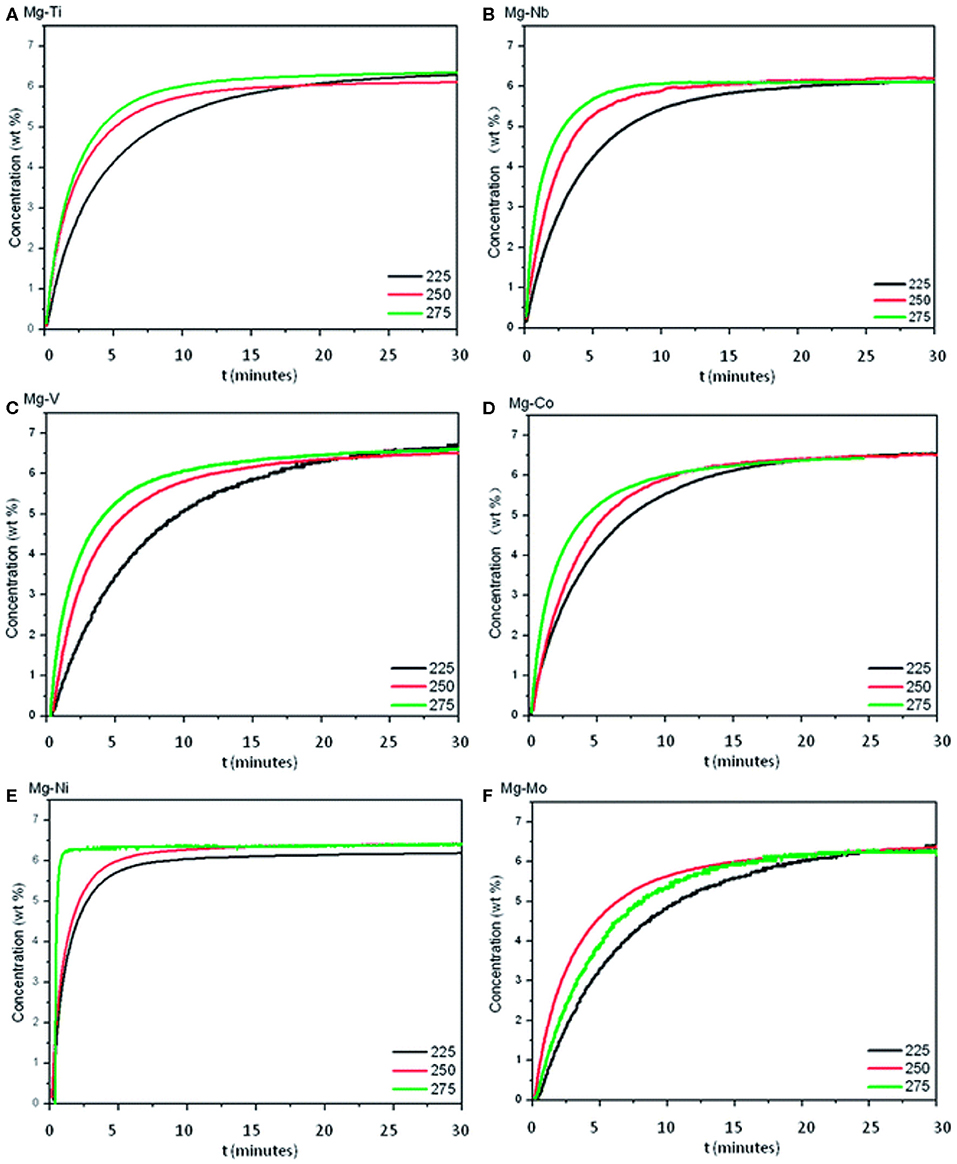
Figure 2. Isothermal dehydrogenation curves of Mg–TM samples at 225, 250, and 275°C. (A) Mg-Ti; (B) Mg-Nb; (C) Mg-V; (D) Mg-Co; (E) Mg-Ni; (F) Mg-Mo. Reproduced from Cui et al. (2014) with permission from Royal Society of Chemistry.
Binary Alloys
Zr-Based Binary Alloys
Recently, many papers reported an interesting strategy for improving hydrogen storage performance of MgH2 by using intermetallic compounds of transition metals as catalyst. The corresponding results showed that the absorption/desorption properties of modified MgH2 systems should be evidently enhanced by the synergetic effects of both phases. Following above idea, we successfully synthesized ZrMn2 nanoparticles ranging from 100 to 300 nm by a facile wet chemical method to explore their catalytic effect on enhancing the hydrogen storage properties of MgH2 (Zhang et al., 2019a). The MgH2+10 wt% nano-ZrMn2 composite began to release hydrogen from 181.9°C and 6.7 wt% hydrogen could be discharged in 5 min at 300°C. Isothermal absorption measurements represented that the dehydrogenated sample could absorb 5.3 wt% hydrogen within 10 min at 100°C under 3 MPa hydrogen pressure. Based on the XRD, TEM and calculation results, the ZrMn2 nanoparticles were distributed well on the surface of MgH2 and helped weaken Mg-H bond strength, which resulted in the enhanced hydrogen storage performance of MgH2 (Figure 3). With the above research experience, we also studied the catalytic effect of ZrCo nanosheets (Zhang et al., 2019c). The modified MgH2 composite could desorb almost 6.3 wt% H2 within 5 min at 300°C and took up 4.4 wt% H2 under 3 Mpa hydrogen pressure in 10 min even at 120°C for doping 10 wt% ZrCo nanosheets. The homogenously distributed ZrCo served as “hydrogen pump” to promote the de/composition of H2 molecules, which was the key to decrease the de/hydrogenation temperature of MgH2. Cycling performance (10 cycles) revealed that there was an apparent reduction in hydrogen storage capacity. In comparison to our studies, the MgH2-ZrNi5 nanocomposite system possessed more excellent hydrogen absorption/desorption performance without serious degradation after 600 complete cycles (El-Eskandarany et al., 2017). The prepared MgH2-10 wt% ZrNi5 sample required 1 and 10 min to absorb and discharge 5.3 wt% H2 at 275°C, respectively. Based on the FE-SEM and XRD results, nano-scaled ZrNi5 grains were uniformly distributed into the MgH2 matrix and ZrNi5 particles could create a network of micro channel that extending into the body of metal hydride to provide hydrogen diffusers during the de/hydrogenation processes.
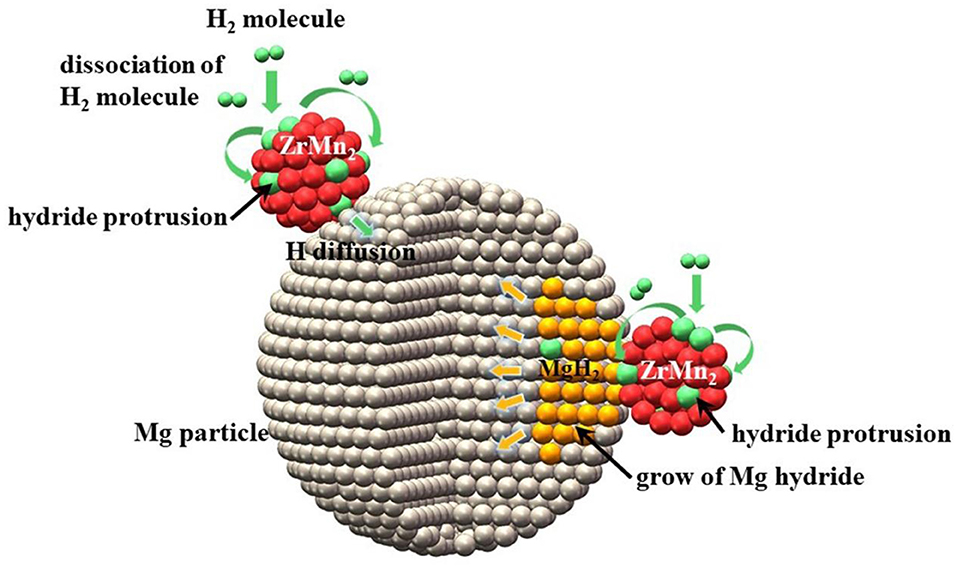
Figure 3. Schematic summary of hydrogenation mechanism of Mg particle catalyzed by ZrMn2 nanoportals. Reproduced from Zhang et al. (2019a) with permission from Royal Society of Chemistry.
Ti-Based Binary Alloys
El-Eskandarany et al. (2019a) employed TiMn2 compound for improving the de/hydrogenation kinetics of MgH2 powders. The 200 h ball milled MgH2-TiMn2 nanocomposites had a nearly spherical shape with particle size ranging between 100 and 320 nm. DSC analysis presented that this composite could absorb/desorb 5.1 wt% hydrogen within 100 and 400 s at 225°C, respectively. For cycling performance, no obvious degradation in storage capacity was found during the long cyclic-life-time (600 h). FE-SEM micrographs highlighted that TiMn2 particles could prevent a serious growth of Mg/MgH2 grains, which led to reduced hydrogen uptake/release kinetics. Neto et al. (2017) doped TiFe compound into MgH2 and concluded that a fine dispersion could be achieved by increasing milling time or using higher energy ball mill. To attain the best hydrogen kinetics, the sample prepared in the planetary mill for 36 h was the optimum selection and the MgH2+ 40 wt%TiFe sample milled for 36 h could release about 3 wt% hydrogen within the first hour. Other Ti-based binary alloys such as TiAl and TiNb have also shown excellent enhancement for catalyzing MgH2 (Zhou et al., 2013). Thermo gravimetric analysis and pressure composition temperature (PCT) isothermal tests showed that the MgH2-TiAl and MgH2-TiNb samples began to desorb hydrogen below 250°C, and the addition of TiAl or TiNb could make the dehydrogenated sample take up hydrogen even at room temperature. In terms of the dehydrogenation reaction, the Mg-H bond would be destabilized by doping with TM elements, which could be confirmed in the theoretical model. The TiAl catalyst illustrated the most effective impact on reducing the activation energy to 65 kJ/mol−1 among the Ti-based catalysts. Nevertheless, the Ti intermetallic catalyst did not change the thermodynamic equilibrium pressure of MgH2.
La-Based Binary Alloys
Rare earth elements, especially lanthanides, are considered as one of the most promising catalysts because of their high activity. Many researchers have used La-based binary alloys as catalyst dopants in MgH2 to explore the resulting catalytic effects. In 2000, Liang et al. (2000) studied MgH2-LaNi5 composite and found that Mg, LaH3, Mg2NiH4 were formed during the milling process. The first desorption of mechanically milled MgH2-30 wt% LaNi5 could release about 4 wt% hydrogen within 150 s at 300°C and the dehydrogenated sample could absorb 3.7 wt% hydrogen in 2,000 s at room temperature. In order to understand the cycling properties of the composite, SEM images manifested that no apparent change in particle size was observed after 20 absorption and desorption cycles. To systematically study the MgH2-LaNi5 composite, MgH2 with different amount of LaNi5 were synthesized by Fu et al. (2008). XRD patterns illustrated that the extended milling time of 40 h caused an additional decrease of peak intensity for the materials containing 5 and 15 wt% LaNi5, which could be ascribed to the brittleness of LaNi5. Further kinetics results showed that the influence of LaNi5 on absorption kinetics was more pronounced at lower temperatures. Additionally, other La-based binary alloys could also improve the hydrogen storage properties of MgH2. El-Eskandarany et al. (2019b) researched the ball-milled MgH2+ nano-LaNi3 composite and found a single amorphous phase after 100 h ball-milling. The milled MgH2-7 wt% LaNi3 sample could discharge 5.6 wt% H2 within 37 min at 225°C. For absorption, the dehydrogenated sample could absorb 3.8 wt% H2 within 40 min at 125°C. In addition, the MgH2-LaNi3 sample possessed an extraordinary long cycle-life-time (2,000 h) at 225°C without obvious degradation on its hydrogen storage capacity.
Other Binary Alloys
Santos et al. (2014) doped a vacuum grade commercial alloy FeNb into MgH2 to study its catalytic effect. The MgH2-FeNb nanocomposites depicted broaden XRD peaks, which indicated small crystallite size and presence of micro strain. Compared with Fe and Nb, the FeNb exhibited lower activity due to the diverse chemical interfacial energies associated to the nano-interfaces of Mg (MgH2)/Fe (or Nb) and Mg (MgH2)/FeNb alloy. Recently, we synthesized FeCo nanosheets and confirmed superior catalytic effect on MgH2 (Yang et al., 2019). For hydrogen storage performance, DSC curves indicated that the MgH2-FeCo composite started to desorb hydrogen from 200°C, which was 150°C lower than that of pure MgH2. The dehydrogenated sample could rapidly uptake H2 from room temperature and almost 6.7 wt% H2 could be absorbed within 1 min at 300°C. Moreover, the MgH2-FeCo composite showed excellent cycling performance over 10 cycles. Further TEM and XRD results demonstrated that the FeCo nanoparticles were evenly distributed on the surface of MgH2 and functioned as “hydrogen spillover,” which referred that the hydrogen molecules dissociated on the surfaces of FeCo nanosheets and in turn facilitated easy transfer of hydrogen atoms to the surface of Mg particles to generate MgH2 during the hydrogenation process. On the other hand, FeCo also effortlessly took up hydrogen atoms from MgH2 to form hydrogen molecule, thus remarkably improved the hydrogen storage properties of MgH2.
Ternary and Multicomponent Alloys
Ti-Based Ternary Alloys
Based on the great improvement of the binary alloy, ternary alloys which replace part of the binary alloy with another transition metal have also been concerned in recent years (Hu et al., 2004; Shahi et al., 2013; El-Eskandarany, 2016; Lu et al., 2018). For instance, Zhou et al. (2013) doped TiVMn alloy into MgH2 to study its hydrogen storage performance. On the contrast with other Ti-based binary alloys catalysts, the dehydrogenation kinetics of the MgH2-TiVMn composite was much better (Figure 4). Moreover, PCT curves also depicted that the addition of TiVMn exhibited the best catalytic effect, which could release more hydrogen under the same condition. The dehydrogenation activation energy was calculated to be 85.2 kJ/mol·H2 by OFW model, which was much lower than that of pure MgH2. Khodaparast and Rajabi (2015) prepared the MgH2+5 at% Ti-Mn-Cr sample by milling the Ti-Mn-Cr alloy produced by melt spinning method with pure MgH2. When Ti-Mn-Cr was doped into MgH2, the dehydrogenation temperature of the composite reduced from 399 to 345°C, much lower than that of prepared MgH2 under the same conditions. Mahmoudi et al. (2011) prepared MgH2-5 at% TiCr1.2Fe0.6 composites at the nanoscale. In comparison to pure MgH2, the initial desorption temperature of the MgH2-5 at% TiCr1.2Fe0.6 sample decreased to 241°C and almost 5 wt% hydrogen could be obtained at 300°C. Further, XRD and TEM studies stated that the interface of the TiCr1.2Fe0.6 alloy with magnesium also acted as active sites for nucleation of the hydride phase, thereby decreasing the nucleation barrier and enhancing the dehydrogenation property.
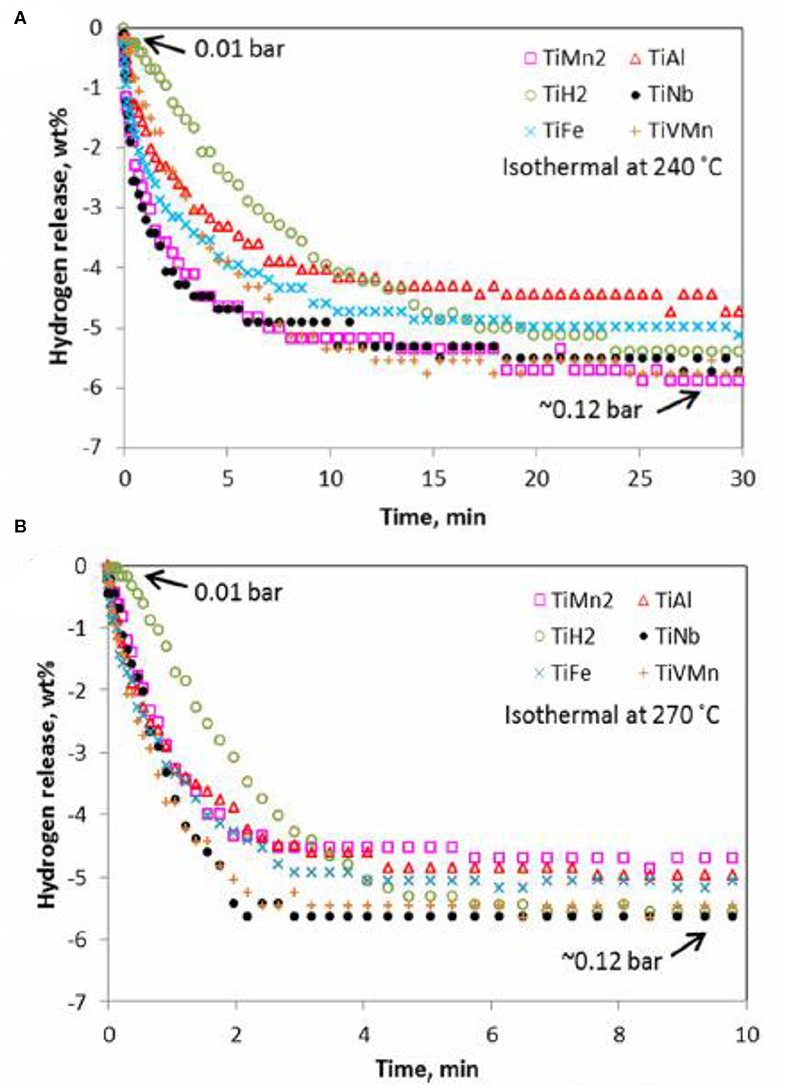
Figure 4. PCT dehydrogenation kinetics for different Ti-based alloy catalyzed magnesium hydrides at 240 °C (A) and 270 °C (B). Reproduced from Zhou et al. (2013) with permission from ACS Publications.
Non-Ti-Based Ternary Alloys
Besides Ti-based alloys, other alloys formed by various single transition metals also illustrated their remarkable effect on improving hydrogen storage performance of MgH2. Agarwal et al. (2009) studied the catalytic effect of ZrCrNi alloy on hydrogenation properties of MgH2. The ZrCrNi alloy was prepared by melting the three pure metals in an arc furnace and then milling with MgH2 for 5 h in a SPEX 8,000 mixer-miller to receive the MgH2-10 wt% ZrCrNi sample. They also performed 20 cycles of de/hydrogenation to explore the stabilization of kinetics and the achievement of hydrogen capacity. In aspect of de/hydrogenation performance, the composite could quickly desorb and absorb about 90% of its maximum hydrogen capacity within 7 min at 300°C after the 20th cycle. XRD and SEM patterns demonstrated that there was no other phases formed during milling and cycling. Also, the alloy was homogeneously dispersed in the MgH2/Mg matrix. To improve hydrogen desorption properties of MgH2, Motavalli and Rajabi (2014) prepared the MgH2-5 at% Ni3FeMn sample by mechanical milling, where the Ni3FeMn catalyst was in two states: as-cast and melt-spun ribbon. DTA curves clarified that 30 h mechanically alloyed catalysts in both states could significantly decrease the desorption temperature. MgH2-Ni3FeMn melt-spun composite could discharge H2 in lower temperature due to the ability to improve particle size refinement of MgH2 and a more pronounced homogeneous distribution of the alloyed elements. The MgH2-5 at% Ni3FeMn melt-spun ribbon composite could release 3.39 wt% hydrogen within 1,000 s at 340°C. Zhou et al. (2019b) doped purchased VTiCr into MgH2 and demonstrated a reversible capacity of 4 wt% H2 between 150 and 350°C for the MgH2-VTiCr composite. Besides, the dehydrogenated sample could absorb hydrogen in a low hydrogen pressure of 0.04–0.4 bar. The VTiCr catalyst was uniformly dispersed on the surface of MgH2 matrix. VTiCr was deemed as a strong catalyst that provided not only excellent catalytic effect but also offer effective cyclic stability in the sense that the reaction kinetics still remained stable after the 10 cycles.
Multicomponent Alloys
As mentioned above, single transition metals and their binary and ternary alloys have shown great catalytic effects on MgH2-based systems (Haghparast and Rajabi, 2015; El-Eskandarany et al., 2018). Further, studies about multicomponent alloys were also stated, Yu et al. (2010) found that the addition of Ti0.4Cr0.15Mn0.15V0.3 alloy could apparently improve the de/absorption properties of MgH2. The MgH2-Ti0.4Cr0.15Mn0.15V0.3 composite began to release hydrogen at 255°C and reached its peak at 294°C, which was much lower than that of unanalyzed MgH2. Besides, the dehydrogenated sample could absorb 3.1 wt% H2 in 500 min even at 29°C. The cycling results manifested that the dehydrogenation rate increased slowly in the first 20 cycles and then remained stable after 20 cycles. SEM and TEM techniques showed that the Ti0.4Cr0.15Mn0.15V0.3 alloy hydride nanoparticles were well-distributed on the surface of MgH2. Meena et al. (2018) found that MgH2 could desorb H2 even at 180°C with the addition of 50 wt% NiMn9.3Al4.0Co14.1Fe3.6 alloy. Compared to as-milled MgH2 sample, the Ea of this composite was lower by about 46.56 kJ/mol. Haghparast and Rajabi (2015) studied the de/hydrogenation kinetics of MgH2-TiCrMn0.4Fe0.4V0.2 composite and found that the dehydrogenation temperature of modified MgH2 decreased to 378°C, which was lower than that of as-received MgH2 (421°C). V45Zr20Ni20Cu10Al3Pd2 powders were doped into MgH2 by El-Eskandarany et al. (2018) and found that the desorption temperature of MgH2-10 wt% V45Zr20Ni20Cu10Al3Pd2 powders was 308.9°C, which was 116°C lower than that of pure MgH2.This prepared nanocomposite possessed superior de/hydrogenation kinetics at relatively low temperature (180°C), absorbing and desorbing 5.5 wt% H2 within 200 s.
Alloys and Carbon Materials
Alloys and Graphene
All above catalytic materials have shown remarkable improvement on the hydrogen storage performance of MgH2, however, stable cycling performance is still the bottleneck for realizing the practical application of MgH2. Carbon materials such as graphene and carbon nanotubes, were widely researched and lots of studies have proven that carbon materials are helpful in preserving stable cycling properties (Xia et al., 2015). Hudson et al. (2016) reported that graphene together with Fe nanoclusters could enhance the hydrogen sorption kinetics of MgH2. From the TPD and DSC curves, the peak temperature of desorption for MgH2+5wt% Fe@G was 281.7°C, lower than that of exhibited peak ball-milled MgH2. In addition, the activation energy of MgH2+5wt% Fe@G composite was reduced to 119.1 kJ/mol (24% lower than that of ball-milled MgH2). Furthermore, TEM confirmed that the grain size of MgH2 increased only 15 nm after 6 cycles, displaying a low grain growth rate during cycling due to the addition of graphene. Density functional theory calculations demonstrated that the defect in graphene and the presence of iron clusters at the defect site of graphene played important role in desorbing hydrogen. Ji et al. (2020) prepared FeNi nanoparticles dispersed on reduced graphene oxide nanosheets (FeNi/rGO) and then found that this catalyst played a vital role in improving the hydrogen storage performance of MgH2. The MgH2-FeNi/rGO sample started to release hydrogen at 230°C and the dehydrogenated sample could absorb 5.4 wt% within 20 min at 125°C. Further investigations proved that FeNi nanoparticles were well distributed on the MgH2 surface in the nanoscale range (Figure 5). More importantly, cycling tests exhibited that 6.9 wt% hydrogen capacity was maintained even after 50 cycles. Singh et al. (2017) investigated the catalytic effect of FeCoNi@GS on hydrogen sorption of MgH2. The onset desorption temperature of this sample was around 255, 25°C lower than that of FeCoNi catalyzed MgH2. The FeCoNi@GS remained stable even after 24 cycles with FeCoNi particles uniformly distributed on the surface of GS.
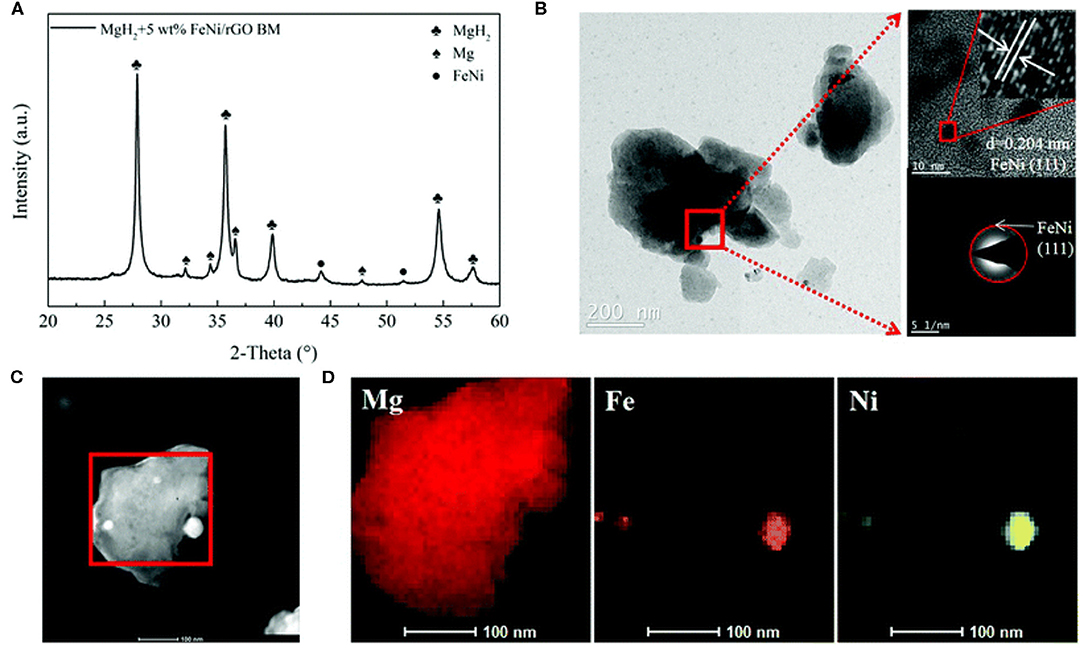
Figure 5. Structural characterization of ball-milled MgH2 + 5 wt% FeNi/rGO: (A) XRD pattern, (B) TEM photograph with the HRTEM image and SAED pattern, and (C,D) corresponding EDS spectra with elemental mapping of Mg, Fe, and Ni. Reproduced from Ji et al. (2020) with permission from Royal Society of Chemistry.
Alloys and Carbon Nanotubes
Carbon nanotubes (CNTs), were widely researched in every field for its small particle size and great microstructure (Luo et al., 2007; Gao et al., 2019; Liu M. et al., 2019). Lillo-Ródenas et al. (2008) demonstrated that the de/hydrogenation performance of MgH2 could be strengthened by the addition of different carbon materials. Comparing with other materials, the mixtures involving CNTs and MWCNTs showed the best results to achieve low temperature operation and high hydrogen storage capacity. Ismail et al. (2014) evidenced an apparent catalytic effect of co-doping MgH2 with FeCl3 and carbon nanotubes on hydrogen storage performance. The CNT-added MgH2-FeCl3 composite started to release hydrogen at 230, 45°C lower than that of MgH2-FeCl3. Moreover, the MgH2-FeCl3/CNT sample could desorb more hydrogen than that of MgH2-FeCl3 under the same isothermal condition. SEM images confirmed that the CNT was not destroyed after the short milling process and indicated that the sample with CNT appeared to have less agglomeration. It was believed that the presence of the unique structure of the CNTs played a critical role in the improvement of hydrogen storage properties in the MgH2-FeCl3/CNTs composite. In our recent investigation (Zhang L. et al., 2020), CNTs combined with Zr0.4Ti0.6Co nanosheets was adopt to strengthen the hydrogen storage properties of MgH2. With the addition of Zr0.4Ti0.6Co sheets, the sorption kinetics were evidently improved while hydrogen capacity was slowly decreasing. Meanwhile, the MgH2-Zr0.4Ti0.6Co/CNTs exhibited no reduction in cycling performance even after 10 cycles after doping CNTs (Figure 6). Deeper structure investigation revealed that particle size of MgH2-Zr0.4Ti0.6Co/CNTs was almost unchanged, contributing to the stable cycling performance.
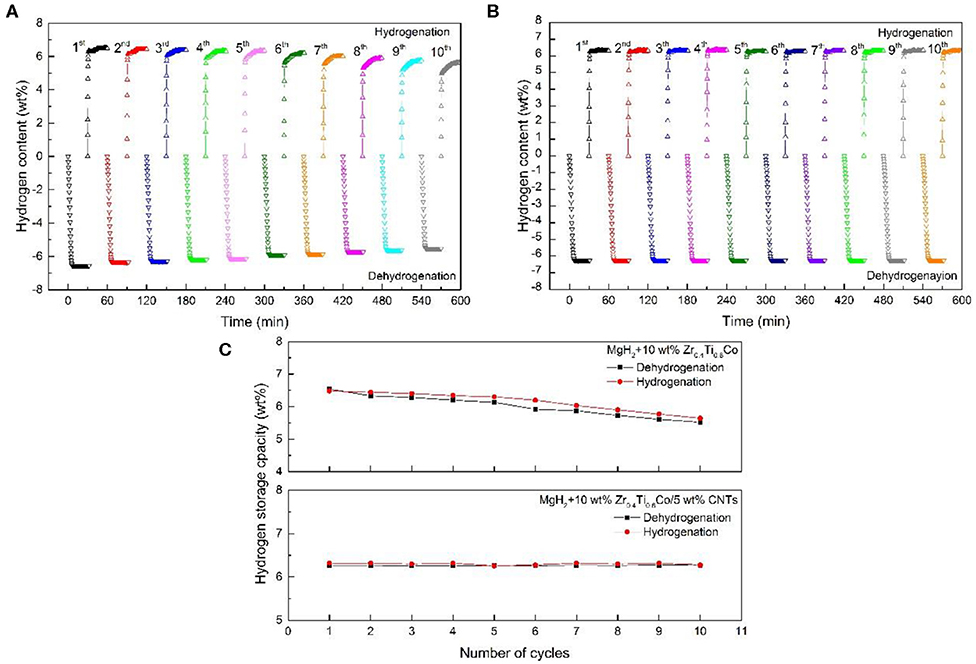
Figure 6. Non-isothermal dehydrogenation/hydrogenation curves of the MgH2+10 wt% Zr0.4Ti0.6Co (A), MgH2+10 wt% Zr0.4Ti0.6Co/5 wt% CNTs (B) composites and as a function of cycling and the resulting line chart (C). Reproduced from Zhang L. et al. (2020) with permission from Elsevier.
Alloys and Other Carbon Materials
Apart from carbon materials mentioned above, other carbon materials also have distinguished effect on ameliorating the de/hydrogenation kinetics of MgH2 (Xia et al., 2018). An et al. (2014) reported that the one-dimensional porous Ni@C nanorods modified MgH2 performed an excellent hydrogen storage properties. The addition of Ni@C decreased the onset temperature of MgH2 to 175°C. Cycling results illustrated no significant loss of hydrogen storage capacity and the MgH2-5 wt% Ni@C composite had favorable cycle stability. Chen et al. (2018) reported the mesoporous carbon CMK-3 performed well in enhancing the hydrogen storage properties of MgH2. The onset desorption temperature of MgH2-10 wt% Ni/CMK-3 was 170°C lower than that of pure MgH2 (above 350°C) and the sample could discharge 6 wt% H2 even at 295°C. The more fascinating fact was that 3.9 wt% hydrogen was absorbed at 55°C for MgH2-Ni/CMK-3 composite. The sample maintained nearly 90.8% of the original de/hydrogenation capacity when cycled for 10 times, indicating that MgH2-Ni/CMK-3 had a good cycle stability. Wang et al. (2018) combined graphene oxide-based porous carbon (GC) and TiCl3 to improve the reversible kinetics of MgH2. The MgH2/GC-TiCl3 composite could reversibly deliver about 7.6 wt% hydrogen at 300°C within 9 min and the average dehydrogenation rate was several times faster than that of the single catalytic MgH2 system. Concerning cycling property, the capacity of the MgH2/GC-TiCl3 sample was also stable with slower kinetics, owing to the nanoconfinement effect of the ball-milled GC. In a word, graphite and carbon with their derivatives could mainly improve the cycling performance, which results in remarkably enhanced the hydrogen storage properties of MgH2.
Conclusions and Perspectives
To realize the practical application of hydrogen energy, numerous effects still need to be carried out in the coming future. For hydrogen storage materials, magnesium hydride is generally believed as a promising material due to its natural abundance, excellent reversibility, light weight and efficient cost. Among the methods investigated, the transition metals have demonstrated excellent catalytic effect on improving the hydrogen storage properties of MgH2. Further studies about alloys based on transition metals are demonstrated to be more effective than the single metal counterparts. In our recent studies, Zr-based alloys and Fe-based alloys were successfully prepared and confirmed to striking improve the de/hydrogenation performance of MgH2. Although the transition metals and their alloys have shown superior enhancement on the de/absorption performance of MgH2, maintaining good cyclic performance is still a challenge for MgH2-based systems. A large number of experiments indicated that carbon materials show excellent effect on maintaining good hydrogen absorption and desorption performance. Our group also demonstrated that carbon nanotubes and reduced graphene oxide together with transition metal alloys can improve the de/hydrogenation kinetics of MgH2 while maintain stable cycling properties at the same time. From above review on literature and our own work, we propose the following strategy to further enhance the hydrogen storage properties of MgH2: (1) regulate the components of transition metal alloys to its best catalytic effect, (2) make the particle size of the alloys as small as possible, (3) combine alloys and carbon materials to synthetically improve the hydrogen storage properties of MgH2. In summary, nanoscale transition metal alloys together with carbon materials would be a promising catalyst for realizing the practical application of MgH2.
Author Contributions
LZ, SS, and JX contributed conception and design of the study. ZS wrote the first draft of the manuscript. XL, FN, and NY wrote sections of the manuscript. All authors contributed to manuscript revision, read and approved the submitted version.
Conflict of Interest
The authors declare that the research was conducted in the absence of any commercial or financial relationships that could be construed as a potential conflict of interest.
Acknowledgments
The authors would like to acknowledge financial support from the National Natural Science Foundation of China (Grant No. 51801078), the National Science Foundation of Jiangsu Province (Grant No. BK20180986) and the Postgraduate Research and Practice Innovation Program of Jiangsu Province (SJCX19_0614).
References
Abe, J. O., Popoola, A. P. I., Ajenifuja, E., and Popoola, O. M. (2019). Hydrogen energy, economy and storage: review and recommendation. Int. J. Hydrogen Energy 44, 15072–15086. doi: 10.1016/j.ijhydene.2019.04.068
Agarwal, S., Aurora, A., Jain, A., Jain, I. P., and Montone, A. (2009). Catalytic effect of ZrCrNi alloy on hydriding properties of MgH2. Int. J. Hydrogen Energy 34, 9157–9162. doi: 10.1016/j.ijhydene.2009.09.034
Ali, N. A., Idris, N. H., Din, M. F. M., Yahya, M. S., and Ismail, M. (2019). Nanoflakes MgNiO2 synthesised via a simple hydrothermal method and its catalytic roles on the hydrogen sorption performance of MgH2. J. Alloy. Compd. 796, 279–286. doi: 10.1016/j.jallcom.2019.05.048
An, C., Liu, G., Li, L., Wang, Y., Chen, C., Wang, Y., et al. (2014). In situ synthesized one-dimensional porous Ni@C nanorods as catalysts for hydrogen storage properties of MgH2. Nanoscale 6, 3223–3230. doi: 10.1039/c3nr05607d
Bassetti, A., Bonetti, E., Pasquini, L., Montone, A., Grbovic, J., and Vittori Antisari, M. (2005). Hydrogen desorption from ball milled MgH2 catalyzed with Fe. Eur. Phys. J. B. 43, 19–27. doi: 10.1140/epjb/e2005-00023-9
Bogdanović, B. R. A., and Spliethoff, B. (1990). Active MgH2ð*Mg systems for reversible chemical energy storage. Angew. Chem. Int. Edit. 29, 223–234. doi: 10.1002/anie.199002233
Bououdina, M., and Guo, Z. X. (2002). Comparative study of mechanical alloying of (Mg+Al) and (Mg+Al+Ni) mixtures for hydrogen storage. J. Alloy. Compd. 336, 222–231. doi: 10.1016/S0925-8388(01)01856-4
Cao, Z., Ouyang, L., Wang, H., Liu, J., Sun, L., Felderhoff, M., et al. (2016). Development of ZrFeV alloys for hybrid hydrogen storage system. Int. J. Hydrogen Energy. 41, 11242–11253. doi: 10.1016/j.ijhydene.2016.04.083
Chen, G., Zhang, Y., Chen, J., Guo, X., Zhu, Y., and Li, L. (2018). Enhancing hydrogen storage performances of MgH2 by Ni nano-particles over mesoporous carbon CMK-3. Nanotechnology 29:265705. doi: 10.1088/1361-6528/aabcf3
Chen, G., Zhang, Y., Cheng, H., Zhu, Y., Li, L., and Lin, H. (2019). Effects of two-dimension MXene Ti3C2 on hydrogen storage performances of MgH2-LiAlH4 composite. Chem. Phys. 522, 178–187. doi: 10.1016/j.chemphys.2019.03.001
Chen, M., Yang, X. B., Cui, J., Tang, J. J., Gan, L. Y., Zhu, M., et al. (2012). Stability of transition metals on Mg(0001) surfaces and their effects on hydrogen adsorption. Int. J. Hydrogen Energy 37, 309–317. doi: 10.1016/j.ijhydene.2011.09.065
Cui, J., Liu, J., Wang, H., Ouyang, L., Sun, D., Zhu, M., et al. (2014). Mg–TM (TM: Ti, Nb, V, Co, Mo or Ni) core–shell like nanostructures: synthesis, hydrogen storage performance and catalytic mechanism. J. Mater. Chem. A 2, 9645–9655. doi: 10.1039/C4TA00221K
El-Eskandarany, M. S. (2016). Metallic glassy Zr70Ni20Pd10 powders for improving the hydrogenation/dehydrogenation behavior of MgH2. Sci. Rep. 6:26936. doi: 10.1038/srep26936
El-Eskandarany, M. S., Ahmed, S. A., and Shaban, E. (2018). Metallic glassy V45Zr20Ni20Cu10Al3Pd2 alloy powders for superior hydrogenation/dehydrogenation kinetics of MgH2. Mater. Today 5, 13718–13725. doi: 10.1016/j.matpr.2018.02.010
El-Eskandarany, M. S., Al-Ajmi, F., Banyan, M., and Al-Duweesh, A. (2019a). Synergetic effect of reactive ball milling and cold pressing on enhancing the hydrogen storage behavior of nanocomposite MgH2/10 wt% TiMn2 binary system. Int. J. Hydrogen Energy 44, 26428–26443. doi: 10.1016/j.ijhydene.2019.08.093
El-Eskandarany, M. S., Saeed, M., Al-Nasrallah, E., Al-Ajmi, F., and Banyan, M. (2019b). Effect of LaNi3 amorphous alloy nanopowders on the performance and hydrogen storage properties of MgH2. Energies 12:1005. doi: 10.3390/en12061005
El-Eskandarany, M. S., Shaban, E., Ali, N., Aldakheel, F., and Alkandary, A. (2016). In-situ catalyzation approach for enhancing the hydrogenation/dehydrogenation kinetics of MgH2 powders with Ni particles. Sci. Rep. 6:37335. doi: 10.1038/srep37335
El-Eskandarany, M. S., Shaban, E., Al-Matrouk, H., Behbehani, M., Alkandary, A., Aldakheel, F., et al. (2017). Structure, morphology and hydrogen storage kinetics of nanocomposite MgH2/10 wt% ZrNi5 powders. Mater. Today Energy 3, 60–71. doi: 10.1016/j.mtener.2016.12.002
Ershova, O. G., Dobrovolsky, V. D., Solonin, Y. M., Khyzhun, O. Y., and Koval, A. Y. (2008). Influence of Ti, Mn, Fe, and Ni addition upon thermal stability and decomposition temperature of the MgH2 phase of alloys synthesized by reactive mechanical alloying. J. Alloy. Compd. 464, 212–218. doi: 10.1016/j.jallcom.2007.10.064
Felderhoff, M., Weidenthaler, C., Helmolt, V. R., and Eberle, U. (2007). Hydrogen storage: the remaining scientific and technological challenges. Phys. Chem. 9, 2643–2653. doi: 10.1039/b701563c
Fu, Y., Groll, M., Mertz, R., and Kulenovic, R. (2008). Effect of LaNi5 and additional catalysts on hydrogen storage properties of Mg. J. Alloy. Compd. 460, 607–613. doi: 10.1016/j.jallcom.2007.06.008
Gao, S., Wang, X., Liu, H., He, T., Wang, Y., Li, S., et al. (2019). Effects of nano-composites (FeB, FeB/CNTs) on hydrogen storage properties of MgH2. J. Power Sources 438:227006. doi: 10.1016/j.jpowsour.2019.227006
Gasan, H., Celik, O. N., Aydinbeyli, N., and Yaman, Y. M. (2012). Effect of V, Nb, Ti and graphite additions on the hydrogen desorption temperature of magnesium hydride. Int. J. Hydrogen Energy 37, 1912–1918. doi: 10.1016/j.ijhydene.2011.05.086
Gosalawit-Utke, R., Nielsen, T. K., Saldan, I., Laipple, D., Cerenius, Y., Jensen, T. R., et al. (2011). Nanoconfined 2LiBH4-MgH2 prepared by direct melt infiltration into nanoporous materials. J. Phys. Chem. C 115, 10903–10910. doi: 10.1021/jp2021903
Goto, K., Hirata, T., Yamamoto, I., and Nakao, W. (2019). Suitability evaluation of LaNi5 as hydrogen-storage-alloy actuator by in-situ displacement measurement during hydrogen pressure change. Molecules 24:2420. doi: 10.3390/molecules24132420
Haghparast, M. R., and Rajabi, M. (2015). Hydrogen desorption properties of MgH2-5 at% Ti-Cr-Mn-Fe-V composite via combined vacuum arc remelting and mechanical alloying. Proc. Mater. Sci. 11, 605–610. doi: 10.1016/j.mspro.2015.11.091
Hanada, N., Ichikawa, T., and Fujii, H. (2005). Catalytic effect of Ni nano-particle and Nb oxide on H-desorption properties in MgH2 prepared by ball milling. J. Alloy. Compd. 404–406, 716–719. doi: 10.1016/j.jallcom.2004.12.166
He, D., Wang, Y., Wu, C., Li, Q., Ding, W., and Sun, C. (2015). Enhanced hydrogen desorption properties of magnesium hydride by coupling non-metal doping and nano-confinement. Appl. Phys. Lett. 107:243907. doi: 10.1063/1.4938245
He, Q., Zhu, D., Wu, X., Dong, D., Xu, M., and Tong, Z. (2019). Hydrogen desorption properties of LiBH4/xLiAlH4 (x = 0.5, 1, 2) composites. Molecules 24:1861. doi: 10.3390/molecules24101861
He, T., Pachfule, P., Wu, H., Xu, Q., and Chen, P. (2016). Hydrogen carriers. Nat. Rev. Mater. 1:12. doi: 10.1038/natrevmats.2016.59
He, T., Wang, X., Liu, H., Gao, S., Wang, Y., Li, S., et al. (2020). Enhanced hydrogen desorption/absorption properties of magnesium hydride with CeF3@Gn. Int. J. Hydrogen Energy 45, 4754–4764. doi: 10.1016/j.ijhydene.2019.12.111
Hu, Y. Q., Yan, C., Zhang, H. F., Ye, L., and Hu, Z. Q. (2004). Preparation and hydrogenation characteristics of Mg−30 wt.% Ti37.5V25Cr37.5 composite. J. Alloy. Compd. 375, 265–269. doi: 10.1016/j.jallcom.2003.11.046
Hudson, M. S. L., Takahashi, K., Ramesh, A., Awasthi, S., Ghosh, A. K., Ravindran, P., et al. (2016). Graphene decorated with Fe nanoclusters for improving the hydrogen sorption kinetics of MgH2-experimental and theoretical evidence. Catal. Sci. Technol. 6, 261–268. doi: 10.1039/C5CY01016K
Ismail, M., Juahir, N., and Mustafa, N. S. (2014). Improved hydrogen storage properties of MgH2 co-doped with FeCl3 and carbon nanotubes. J. Phys. Chem. C 118, 18878–18883. doi: 10.1021/jp5046436
Jain, I. P., Lal, C., and Jain, A. (2020). Hydrogen storage in Mg: a most promising material. Int. J. Hydrogen Energy 35, 5133–5144. doi: 10.1016/j.ijhydene.2009.08.088
Jansa, L. I., Kalantzopoulos, G. N., Nordholm, K., and Hauback, B. C. (2020). Destabilization of NaBH4 by transition metal fluorides. Molecules 25:780. doi: 10.3390/molecules25040780
Jeon, K. J., Moon, H. R., Ruminski, A. M., Jiang, B., Kisielowski, C., Bardhan, R., et al. (2011). Air-stable magnesium nanocomposites provide rapid and high-capacity hydrogen storage without using heavy-metal catalysts. Nat. Mater. 10, 286–290. doi: 10.1038/nmat2978
Ji, L., Zhang, L., Yang, X., Zhu, X., and Chen, L. (2020). The remarkably improved hydrogen storage performance of MgH2 by the synergetic effect of an FeNi/rGO nanocomposite. Dalton Trans. 49:4146. doi: 10.1039/D0DT00230E
Khafidz, N. Z. A. K., Yaakob, Z., Lim, K. L., and Timmiati, S. N. (2016). The kinetics of lightweight solid-state hydrogen storage materials: a review. Int. J. Hydrogen Energy 41, 13131–13151. doi: 10.1016/j.ijhydene.2016.05.169
Khodaparast, V., and Rajabi, M. (2015). Hydrogen desorption properties of MgH2-5 wt% Ti-Mn-Cr composite via combined melt spinning and mechanical alloying. Proc. Mater. Sci. 11, 611–615. doi: 10.1016/j.mspro.2015.11.092
Kim, J., Jun, A., Gwon, O., Yoo, S., Liu, M., Shin, J., et al. (2018). Hybrid-solid oxide electrolysis cell: a new strategy for efficient hydrogen production. Nano Energy 44, 121–126. doi: 10.1016/j.nanoen.2017.11.074
Konarova, M., Tanksale, A., Beltramini, J. N., Lu, G., and Qing. (2013). Effects of nano-confinement on the hydrogen desorption properties of MgH2. Nano Energy 2, 98–104. doi: 10.1016/j.nanoen.2012.07.024
Kumar, V., Pukazhselvan, D., Tyagi, A. K., and Singh, S. K. (2013). Effect of Ni concentration on the structural and hydrogen storage characteristics of Zr–Mn based laves phase system. Mater. Renew. Sust. Energy 2:12. doi: 10.1007/s40243-013-0012-3
Liang, G., Hout, J., Boil, S., Neste, V. A., and Schul, R. (1999b). Hydrogen storage properties of the mechanically milled MgH2-V nanocomposite. J. Alloy. Compd. 291, 295–299. doi: 10.1016/S0925-8388(99)00268-6
Liang, G., Hout, J., Boily, S., Neste, V. A., and Schulz, R. (1999a). Catalytic effect of transition metals on hydrogen sorption in nanocrystalline ball milled MgH2 -Tm (Tm=Ti, V, Mn, Fe and Ni) systems. J. Alloy. Compd. 292, 247–252. doi: 10.1016/S0925-8388(99)00442-9
Liang, G., Hout, J., Boily, S., Neste, V. A., and Schulz, R. (2000). Hydrogen storage in mechanically milled Mg-LaNi5 and MgH2-LaNi5 composites. J. Alloy. Compd. 297, 261–265. doi: 10.1016/S0925-8388(99)00592-7
Liao, B., Lei, Y. Q., Chen, L. X., Lu, G. L., Pan, H. G., and Wang, Q. D. (2004). Effect of the La/Mg ratio on the structure and electrochemical properties of LaxMg3-xNi9 (x=1.6–2.2) hydrogen storage electrode alloys for nickel–metal hydride batteries. J. Power Sources 129, 358–367. doi: 10.1016/j.jpowsour.2003.11.044
Lillo-Ródenas, M. A., Guo, Z. X., Aguey-Zinsou, K. F., Cazorla-Amorós, D., and Linares-Solano, A. (2008). Effects of different carbon materials on MgH2 decomposition. Carbon 46, 126–137. doi: 10.1016/j.carbon.2007.10.033
Liu, H., Ma, H., Zhang, L., Gao, S., Wang, X., Xu, L., et al. (2019a). Wet chemical synthesis of non-solvated rod-Like alpha'-AlH3 as a hydrogen storage material. Front. Chem. 7:892. doi: 10.3389/fchem.2019.00892
Liu, H., Zhang, L., Ma, H., Lu, C., Luo, H., Wang, X., et al. (2020). Aluminum hydride for solid-state hydrogen storage: structure, synthesis, thermodynamics, kinetics, and regeneration. J. Energy Chem. 52, 428–440.doi: 10.1016/j.jechem.2020.02.008
Liu, M., Xiao, X., Zhao, S., Saremi-Yarahmadi, S., Chen, M., Zheng, J., et al. (2019b). ZIF-67 derived Co@CNTs nanoparticles: remarkably improved hydrogen storage properties of MgH2 and synergetic catalysis mechanism. Int. J. Hydrogen Energy 44, 1059–1069. doi: 10.1016/j.ijhydene.2018.11.078
Lu, Y., Wang, H., Liu, J., Ouyang, L., and Zhu, M. (2018). Destabilizing the dehydriding thermodynamics of MgH2 by reversible intermetallics formation in Mg–Ag–Zn ternary alloys. J. Power Sources 396, 796–802. doi: 10.1016/j.jpowsour.2018.06.060
Luo, Y., Wang, P., Ma, L. P., and Cheng, H. M. (2007). Enhanced hydrogen storage properties of MgH2 co-catalyzed with NbF5 and single-walled carbon nanotubes. Scripta Mater. 56, 765–768. doi: 10.1016/j.scriptamat.2007.01.016
Mahmoudi, N., Kaflou, A., and Simchi, A. (2011). Hydrogen desorption properties of MgH2-TiCr1.2Fe0.6 nanocomposite prepared by high-energy mechanical alloying. J. Power Sources 196, 4604–4608. doi: 10.1016/j.jpowsour.2011.01.001
Meena, P., Singh, R., Sharma, V. K., and Jain, I. P. (2018). Role of NiMn9.3Al4.0Co14.1Fe3.6 alloy on dehydrogenation kinetics of MgH2. J. Magnes. Alloy. 6, 318–325. doi: 10.1016/j.jma.2018.05.007
Montone, A., Aurora, A., Gattia, D. M., and Antisari, M. V. (2012). Microstructural and kinetic evolution of Fe doped MgH2 during H2 cycling. Catalysts 2, 400–411. doi: 10.3390/catal2030400
Motavalli, A., and Rajabi, M. (2014). Catalytic effect of melt-spun Ni3FeMn alloy on hydrogen desorption properties of nanocrystalline MgH2 synthesized by mechanical alloying. Int. J. Hydrogen Energy 39, 17047–17053. doi: 10.1016/j.ijhydene.2014.08.061
Neto, R. M. L., Rafael, A. S., Floriano, R., Coutinho, G. C. S., Falcão, R. B., and Leiva, D. R. (2017). Synthesis by high-energy ball milling of MgH2-TiFe composites for hydrogen storage. Mater. Sci. Forum. 899, 13–18. doi: 10.4028/www.scientific.net/MSF.899.13
Nielsen, T. K., Manickam, K., and Hirscher, M. (2009). Confinement of MgH\r, 2\r nanoclusters within nanoporous aerogel scaffold materials. ACS Nano. 3, 3521–3528. doi: 10.1021/nn901072w
Norberg, N. S., Arthur, T. S., Fredrick, S. J., and Prieto, A. L. (2011). Size-dependent hydrogen storage properties of Mg nanocrystals prepared from solution. J. Am. Chem. Soc. 133, 10679–10681. doi: 10.1021/ja201791y
Peng, X., Wang, H., Hu, R., Ouyang, L., Liu, J., and Zhu, M. (2017). Electrochemical performances of MgH2 and MgH2-C films for lithium ion battery anode. J. Alloy. Compd. 711, 473–479. doi: 10.1016/j.jallcom.2017.03.180
Peter, S. C. (2018). Reduction of CO2 to chemicals and fuels: a solution to global warming and energy crisis. ACS Energy Lett. 3, 1557–1561. doi: 10.1021/acsenergylett.8b00878
Pighin, S. A., Capurso, G., Russo, S. L., and Peretti, H. A. (2012). Hydrogen sorption kinetics of magnesium hydride enhanced by the addition of Zr8Ni21 alloy. J. Alloy. Compd. 530, 111–115. doi: 10.1016/j.jallcom.2012.03.100
Pluengphon, P., Bovornratanaraks, T., Tsuppayakorn-aek, P., Pinsook, U., and Inceesungvorn, B. (2019). High-pressure phases induce H-vacancy diffusion kinetics in TM-doped MgH2: Ab initio study for hydrogen storage improvement. Int. J. Hydrogen Energy. 44, 21948–21954. doi: 10.1016/j.ijhydene.2019.06.066
Pudukudy, M., Yaakob, Z., Mohammad, M., Narayanan, B., and Sopian, K. (2014). Renewable hydrogen economy in Asia–Opportunities and challenges: an overview. Renew. Sust. Energ. Rev. 30, 743–757. doi: 10.1016/j.rser.2013.11.015
Pukazhselvan, D., Kumar, V., and Singh, S. K. (2012). High capacity hydrogen storage: basic aspects, new developments and milestones. Nano Energy.1, 566–589. doi: 10.1016/j.nanoen.2012.05.004
Razavi, F. S., Morassaei, M. S., Salehabadi, A., Salavati-Niasari, M., and Moayedi, H. (2019). Auto-combustion synthesis, structural analysis, and electrochemical solid-state hydrogen storage performance of strontium cobalt oxide nanostructures. Int. J. Hydrogen Energy 44, 31183–31191. doi: 10.1016/j.ijhydene.2019.10.012
Rusman, N. A. A., and Dahari, M. (2016). A review on the current progress of metal hydrides material for solid-state hydrogen storage applications. Int. J. Hydrogen Energy 41, 12108–12126. doi: 10.1016/j.ijhydene.2016.05.244
Sadhasivam, T., Kim, H., Jung, S., Roh, S., Park, J., and Jung, H. (2017). Dimensional effects of nanostructured Mg/MgH2 for hydrogen storage applications: a review. Renew. Sust. Energ. Rev. 72, 523–534. doi: 10.1016/j.rser.2017.01.107
Santos, S. F., Ishikawa, T. T., Botta, W. J., and Huot, J. (2014). MgH2+FeNb nanocomposites for hydrogen storage. Mater. Chem. Phys. 147, 557–562. doi: 10.1016/j.matchemphys.2014.05.031
Shahi, R. R., Tiwari, A. P., Shaz, M. A., and Srivastava, O. N. (2013). Studies on de/rehydrogenation characteristics of nanocrystalline MgH2 co-catalyzed with Ti, Fe and Ni. Int. J. Hydrogen Energy 38, 2778–2784. doi: 10.1016/j.ijhydene.2012.11.073
Shang, C. (2004). Mechanical alloying and electronic simulations of (MgH2+M) systems (M=Al, Ti, Fe, Ni, Cu and Nb) for hydrogen storage. Int. J. Hydrogen Energy 29, 73–80. doi: 10.1016/S0360-3199(03)00045-4
Shao, H., Felderhoff, M., Schuth, F., and Weidenthaler, C. (2011). Nanostructured Ti-catalyzed MgH2 for hydrogen storage. Nanotechnology 22:235401. doi: 10.1088/0957-4484/22/23/235401
Shao, J., Xiao, X., Fan, X., Huang, X., Zhai, B., Li, S., et al. (2015). Enhanced hydrogen storage capacity and reversibility of LiBH4 nanoconfined in the densified zeolite-templated carbon with high mechanical stability. Nano Energy 15, 244–255. doi: 10.1016/j.nanoen.2015.04.023
Singh, M. K., Bhatnagar, A., Pandey, S. K., Mishra, P. C., and Srivastava, O. N. (2017). Experimental and first principle studies on hydrogen desorption behavior of graphene nanofibre catalyzed MgH2. Int. J. Hydrogen Energy 42, 960–968. doi: 10.1016/j.ijhydene.2016.09.210
Song, J., Wang, J., Hu, X., Meng, D., and Wang, S. (2019). Activation and disproportionation of Zr2Fe alloy as hydrogen storage material. Molecules 24:1542. doi: 10.3390/molecules24081542
Sterl, F., Linnenbank, H., Steinle, T., Morz, F., Strohfeldt, N., and Giessen, H. (2018). Nanoscale hydrogenography on single magnesium nanoparticles. Nano Lett. 18, 4293–4302. doi: 10.1021/acs.nanolett.8b01277
Su, W., Zhu, Y., Zhang, J., Liu, Y., Yang, Y., Mao, Q., et al. (2016). Effect of multi-wall carbon nanotubes supported nano-nickel and TiF3 addition on hydrogen storage properties of magnesium hydride. J.Alloy. Compd. 669, 8–18. doi: 10.1016/j.jallcom.2016.01.253
Sun, G., Li, Y., Zhao, X., Wu, J., Wang, L., and Mi, Y. (2016). First-principles investigation of the effects of Ni and Y co-doped on destabilized MgH2. RSC Adv. 6, 23110–23116. doi: 10.1039/C5RA23996F
Tanniru, M., Slattery, D. K., and Ebrahimi, F. (2020). A study of stability of MgH2 in Mg−8at%Al alloy powder. Int. J. Hydrogen Energy. 35, 3555–3564. doi: 10.1016/j.ijhydene.2010.01.109
Wan, C., Zhou, L., Sun, L., Xu, L. X., Cheng, D. G., Chen, F. Q., et al. (2020). Boosting visible-light-driven hydrogen evolution from formic acid over AgPd/2D g-C3N4 nanosheets Mott-Schottky photocatalyst. Chem. Eng. J. 396:125229. doi: 10.1016/j.cej.2020.125229
Wang, H., Lin, H. J., Cai, W. T., Ouyang, L. Z., and Zhu, M. (2016). Tuning kinetics and thermodynamics of hydrogen storage in light metal element based systems – A review of recent progress. J. Alloy. Compd. 658, 280–300. doi: 10.1016/j.jallcom.2015.10.090
Wang, K., Wu, G., Cao, H., Li, H., and Zhao, X. (2018). Improved reversible dehydrogenation properties of MgH2 by the synergetic effects of graphene oxide-based porous carbon and TiCl3. Int. J. Hydrogen Energy 43, 7440–7446. doi: 10.1016/j.ijhydene.2018.02.195
Wang, Y., Zhang, Q., Wang, Y., Jiao, L., and Yuan, H. (2015). Catalytic effects of different Ti-based materials on dehydrogenation performances of MgH2. J. Alloy. Compd. 645, S509–S512. doi: 10.1016/j.jallcom.2014.12.071
Wang, Z., Zhang, X., Ren, Z., Liu, Y., Hu, J., Li, H., et al. (2019). In situ formed ultrafine NbTi nanocrystals from a NbTiC solid-solution MXene for hydrogen storage in MgH2. J. Mater. Chem. A 7, 14244–14252. doi: 10.1039/C9TA03665B
Webb, C. J. (2015). A review of catalyst-enhanced magnesium hydride as a hydrogen storage material. J. Phys. Chem. Solids 84, 96–106. doi: 10.1016/j.jpcs.2014.06.014
Winter, C. J. (2009). Hydrogen energy — Abundant, efficient, clean: a debate over the energy-system-of-change. Int. J. Hydrogen Energy 34, S1–S52. doi: 10.1016/j.ijhydene.2009.05.063
Xia, G. L., Tan, Y. B., Chen, X. W., Sun, D. L., Guo, Z. P., Liu, H. K., Ouyang, L. Z., et al. (2015). Monodisperse magnesium hydride nanoparticles uniformly self-assembled on graphene. Adv. Mater. 27, 5981–5988. doi: 10.1002/adma.201502005
Xia, G. L., Zhang, L. J., Chen, X. W., Huang, Y. Q., Sun, D. L., Fang, F., et al. (2018). Carbon hollow nanobubbles on porous carbon nanofibers: an ideal host for high-performance sodium-sulfur batteries and hydrogen storage. Energy Storage Mat. 14, 314–323. doi: 10.1016/j.ensm.2018.05.008
Xiao, X., Qin, T., Jiang, Y., Jiang, F., Li, M., Fan, X., et al. (2017). Significantly enhanced hydrogen desorption properties of Mg(AlH4)2 nanoparticles synthesized using solvent free strategy. Prog. Natl.Acad. Sci. U.S.A. 27, 112–120. doi: 10.1016/j.pnsc.2017.01.006
Xie, L., Liu, Y., Zhang, X., Qu, J., Wang, Y., and Li, X. (2009). Catalytic effect of Ni nanoparticles on the desorption kinetics of MgH2 nanoparticles. J. Alloy. Compd. 482, 388–392. doi: 10.1016/j.jallcom.2009.04.028
Xu, S., Wang, F., Tang, W., Wang, Y., and Yu, R. (2018). Microstructure and hydrogen storage properties of Zr0.8Ti0.2Co1−−xFe (x=0, 0.1, 0.2, 0.3) alloys. Int. J. Hydrogen Energy 43, 839–847. doi: 10.1016/j.ijhydene.2017.11.151
Yang, J., Sudik, A., Wolverton, C., and Siegel, D. J. (2010). High capacity hydrogen storage materials: attributes for automotive applications and techniques for materials discovery. Chem. Soc. Rev. 39, 656–675. doi: 10.1039/B802882F
Yang, W. N., Shang, C. X., and Guo, Z. X. (2010). Site density effect of Ni particles on hydrogen desorption of MgH2. Int. J. Hydrogen Energy 35, 4534–4542. doi: 10.1016/j.ijhydene.2010.02.047
Yang, X., Ji, L., Yan, N., Sun, Z., Lu, X., Zhang, L., et al. (2019). Superior catalytic effects of FeCo nanosheets on MgH2 for hydrogen storage. Dalton Trans. 48, 12699–12706. doi: 10.1039/C9DT02084E
Yao, L., Lyu, X., Zhang, J., Liu, Y., Zhu, Y., Lin, H., et al. (2020). Remarkable synergistic effects of Mg2NiH4 and transition metal carbides (TiC, ZrC, WC) on enhancing the hydrogen storage properties of MgH2. Int. J. Hydrogen Energy 45, 6765–6779. doi: 10.1016/j.ijhydene.2019.12.139
Yavari, A., Lemoulec, A., Decastro, F., Deledda, S., Friedrichs, O., Botta, W., et al. (2005). Improvement in H-sorption kinetics of MgH powders by using Fe nanoparticles generated by reactive FeF addition. Scripta Mater. 52, 719–724. doi: 10.1016/j.scriptamat.2004.12.020
Yu, H., Bennici, S., and Auroux, A. (2014). Hydrogen storage and release: kinetic and thermodynamic studies of MgH2 activated by transition metal nanoparticles. Int. J. Hydrogen Energy. 39, 11633–11641. doi: 10.1016/j.ijhydene.2014.05.069
Yu, X., Tang, Z., Sun, D., Ouyang, L., and Zhu, M. (2017). Recent advances and remaining challenges of nanostructured materials for hydrogen storage applications. Prog. Mater. Sci. 88, 1–48. doi: 10.1016/j.pmatsci.2017.03.001
Yu, X. B., Yang, Z. X., Liu, H. K., Grant, D. M., and Walker, G. S. (2010). The effect of a Ti-V-based BCC alloy as a catalyst on the hydrogen storage properties of MgH2. Intern. J. Hydrogen Energy 35, 6338–6344. doi: 10.1016/j.ijhydene.2010.03.089
Zahiri, B., Danaie, M., Tan, X., Amirkhiz, B. S., Botton, G. A., and Mitlin, D. (2012). Stable hydrogen storage cycling in magnesium hydride, in the range of room temperature to 300 °C, achieved using a new bimetallic Cr-V nanoscale catalyst. J. Phys. Chem. C 116, 3188–3199. doi: 10.1021/jp211254k
Zhai, B., Xiao, X., Lin, W., Huang, X., Fan, X., Li, S., et al. (2016). Enhanced hydrogen desorption properties of LiBH4-Ca(BH4)2 by a synergetic effect of nanoconfinement and catalysis. Int. J. Hydrogen Energy 41, 17462–17470. doi: 10.1016/j.ijhydene.2016.06.170
Zhang, J., Shi, R., Zhu, Y., Liu, Y., Zhang, Y., Li, S., et al. (2018). Remarkable synergistic catalysis of Ni-doped ultrafine TiO2 on hydrogen sorption kinetics of MgH2. ACS Appl. Mater. Inter. 10, 24975–24980. doi: 10.1021/acsami.8b06865
Zhang, L., Cai, Z., Yao, Z., Ji, L., Sun, Z., Yan, N., et al. (2019a). A striking catalytic effect of facile synthesized ZrMn2 nanoparticles on the de/rehydrogenation properties of MgH2. J. Mater. Chem. A 7, 5626–5634. doi: 10.1039/C9TA00120D
Zhang, L., Cai, Z., Zhu, X., Yao, Z., Sun, Z., Ji, L., et al. (2019b). Two-dimensional ZrCo nanosheets as highly effective catalyst for hydrogen storage in MgH2. J. Alloy. Compd. 805, 295–302. doi: 10.1016/j.jallcom.2019.07.085
Zhang, L., Ji, L., Yao, Z., Yan, N., Sun, Z., Yang, X., et al. (2019c). Facile synthesized Fe nanosheets as superior active catalyst for hydrogen storage in MgH2. Int. J. Hydrogen Energy 44, 21955–21964. doi: 10.1016/j.ijhydene.2019.06.065
Zhang, L., Sun, Z., Cai, Z., Yan, N., Lu, X., Zhu, X., et al. (2020a). Enhanced hydrogen storage properties of MgH2 by the synergetic catalysis of Zr0.4Ti0.6Co nanosheets and carbon nanotubes. Appl. Surf. Sci. 504:144465. doi: 10.1016/j.apsusc.2019.144465
Zhang, X. L., Liu, Y. F., Zhang, X., Hu, J. J., Gao, M. X., and Pan, H. G. (2020b). Empowering hydrogen storage performance of MgH2 by nanoengineering and nanocatalysis. Mater.Today Nano 9:100064. doi: 10.1016/j.mtnano.2019.100064
Zhou, C., Bowman, R. C., Fang, Z. Z., Lu, J., Xu, L., Sun, P., et al. (2019a). Amorphous TiCu-based additives for improving hydrogen storage properties of magnesium hydride. ACS Appl. Mater. Interfaces 11, 38868–38879. doi: 10.1021/acsami.9b16076
Zhou, C., Fang, Z. Z., Ren, C., Li, J., and Lu, J. (2013). Effect of Ti intermetallic catalysts on hydrogen storage properties of magnesium hydride. J. Phys. Chem. C 117, 12973–12980. doi: 10.1021/jp402770p
Keywords: hydrogen storage, MgH2, transition metals, carbon materials, cycling performance
Citation: Sun Z, Lu X, Nyahuma FM, Yan N, Xiao J, Su S and Zhang L (2020) Enhancing Hydrogen Storage Properties of MgH2 by Transition Metals and Carbon Materials: A Brief Review. Front. Chem. 8:552. doi: 10.3389/fchem.2020.00552
Received: 14 April 2020; Accepted: 28 May 2020;
Published: 02 July 2020.
Edited by:
Guanglin Xia, Fudan University, ChinaCopyright © 2020 Sun, Lu, Nyahuma, Yan, Xiao, Su and Zhang. This is an open-access article distributed under the terms of the Creative Commons Attribution License (CC BY). The use, distribution or reproduction in other forums is permitted, provided the original author(s) and the copyright owner(s) are credited and that the original publication in this journal is cited, in accordance with accepted academic practice. No use, distribution or reproduction is permitted which does not comply with these terms.
*Correspondence: Liuting Zhang, zhanglt89@just.edu.cn