- 1Dipartimento per la Innovazione nei Sistemi Biologici, Agroalimentari e Forestali (DIBAF), Università della Tuscia, Viterbo, Italy
- 2Istituto per i Sistemi Biologici del CNR, Monterotondo, Italy
Cationic species with noble gas (Ng)-hydrogen bonds play a major role in the gas-phase ion chemistry of the group 18 elements. These species first emerged more than 90 years ago, when the simplest HeH+ and HeH were detected from ionized He/H2 mixtures. Over the years, the family has considerably expanded and currently includes various bonding motifs that are investigated with intense experimental and theoretical interest. Quite recently, the results of these studies acquired new and fascinating implications. The diatomic ArH+ and HeH+ were, in fact, detected in various galactic and extragalactic regions, and this stimulates intriguing questions concerning the actual role in the outer space of the Ng-H cations observed in the laboratory. The aim of this review is to briefly summarize the most relevant information currently available on the structure, stability, and routes of formation of these fascinating systems.
About 130 years after the discovery of argon (Rayleigh, 1895), the chemistry of the noble gases currently appears as a fascinating “saga” (Grandinetti, 2018), where combative scientists never tire of using different chemical and physical weapons to challenge and defeat the proverbial inertness of the elements. Exemplary in this regard is the unceasing interest focused on gaseous ionic species. The story began in 1925, when Hogness and Lunn (1925) first detected the simplest HeH+ and HeH from ionized He/H2 mixtures. With the upsurge of interest in gas-phase ion-molecule reactions (Stevenson, 1957; Giese et al., 1961), it soon emerged that, under ionizing conditions, all the noble gas atoms (Ng) were “forgetting” to be inert, and capable of combining with a huge variety of atoms and molecules. The subsequent studies actually confirmed an exciting ion chemistry of both fundamental and applied interest (Grandinetti, 2011). None could, however, surmise the amazing implications that emerged quite recently, when ArH+ and HeH+ were detected in various galactic and extragalactic regions (Barlow et al., 2013; Schilke et al., 2014; Müller et al., 2015; Güsten et al., 2019). This unexpected projection from the ion sources to outer space rejuvenates particular interest in cationic noble-gas hydrides NgmHn+ (m, n ≥ 1), a well-established family of noble gas ions. The pertinent literature is already vast, and the contributions chosen here wish to illustrate issues of current interest that are also of relevance for the naturally-occurring chemistry. The systems are described so as to give an overview, useful also as a guide for future studies.
NgH+
The chemistry of the gaseous NgmHn+ plays around four major bonding motifs, namely the NgH+, the linear centro-symmetric Ng-H-Ng+, and their Ng-solvated complexes (NgHNg+)(Ng)n, the (H)(Ng)n, and the (H)(Ng)n (n ≥ 1). Their formations also benefit from ion sources operated at ultra-cold temperatures (Jašik et al., 2013), or from ionized helium nanodroplets doped with suitable precursors (Fárník and Toennies, 2005). The connectivities of some exemplary species are shown in Figure 1, and some quantitative data are reported in Table 1.
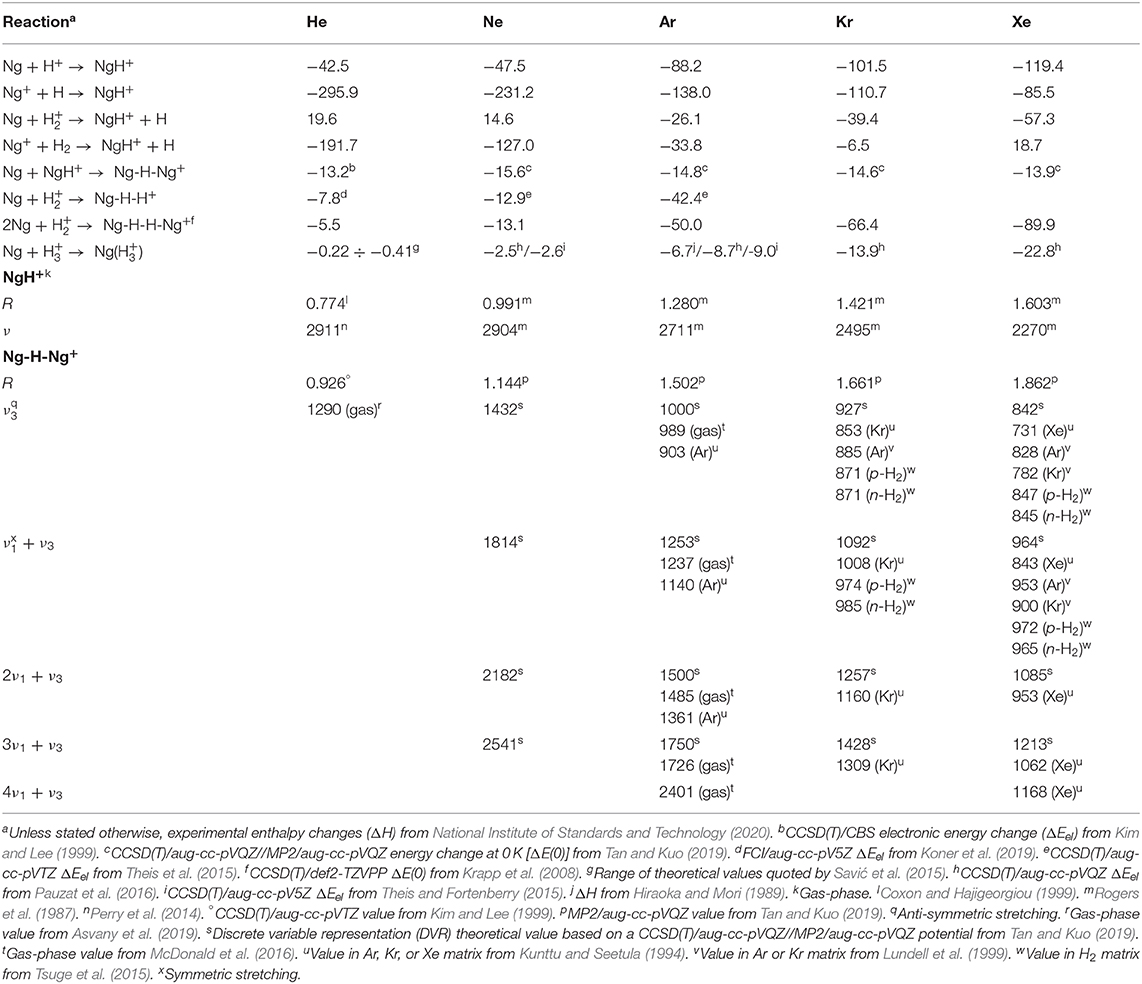
Table 1. Energetics (kcal mol−1) of reactions involving the gaseous NgmHn+, and bond distances (R, Å) and vibrational frequencies (ν, cm−1) of the NgH+ and Ng-H-Ng+.
The diatomic NgH+ (Ng = He-Xe), still elusive in any other environment, are quite stable in the gas phase. Their simplest ionic routes of formation given by the equations
are, invariably, exothermic (National Institute of Standards and Technology, 2020), with the only exception of He and Ne reacting with ground-state H (see Table 1). The galactic and extragalactic ArH+ is, in particular, ascribed to reactions (3) and (4) (Barlow et al., 2013; Theis et al., 2015), whereas the HeH+ observed in the planetary nebulae (Güsten et al., 2019) mainly arises from reaction (2) (Roberge and Dalgarno, 1982; Cecchi-Pestellini and Dalgarno, 1993; Fortenberry, 2019). In keeping with any type of bonding analysis (Borocci et al., 2015), the short bond distances, and high vibrational frequencies of the NgH+ point to typical covalent species. The experimental values (Rogers et al., 1987, and references cited therein; Coxon and Hajigeorgiou, 1999; Perry et al., 2014; Gruet and Pirali, 2019) (see Table 1) range between 0.774 and 1.603 Å, and 2,911 and 2,270 cm−1, respectively, and follow the expected periodic increase/decrease of R/ν when going from HeH+ to XeH+.
Ng-H-Ng+ and (NgHNg+)(Ng)n (n ≥ 1)
The addition of another Ng to NgH+ produces the linear centro-symmetric Ng-H-Ng+. Their thermochemistry is experimentally unknown, but theoretical calculations (Kim and Lee, 1999; Tan and Kuo, 2019) predict Ng additions that are exothermic by ca. 13–17 kcal mol−1. The alternative conceivable Ng-Ng-H+ are instead only marginally stable (Bop et al., 2017a,b), and do not play a role in the chemistry and the dynamics of the gaseous H+(Ngm) (Ritschel et al., 2004, 2005, 2007). The structural assignment of the Ng-H-Ng+ is based first on matrix infrared (IR) spectroscopy. Definitive evidence in this regard was obtained, in particular, by Kunttu and his coworkers (Kunttu et al., 1992; Kunttu and Seetula, 1994), who identified the symmetric (ν1) and anti-symmetric (ν3) stretching that appear in the IR spectra of the Ng-H-Ng+ (Ng = Ar, Kr, Xe) as a progression of (nν1 + ν3), with n up to 4 for Xe-H-Xe+. The ν1 and (ν1 + ν3) absorptions of Kr-H-Kr+ and Xe-H-Xe+ were subsequently measured in different matrices (Lundell et al., 1999; Tsuge et al., 2015), and found to be sensitive to the environment. As shown in Table 1, the comparison with very recent theoretical estimates (Tan and Kuo, 2019) clearly unravels that the “cold” bands are red-shifted by up to 100 cm−1 with respect to those of the naked Ng-H-Ng+. Consistently, the theoretical IR absorptions of Ar-H-Ar+ are instead quite close to the corresponding gas-phase values obtained by Duncan and his coworkers (McDonald et al., 2016). They produced the entire family of the smallest (Ar-H-Ar+)Arn (n = 1–5), and measured the IR photodissociation spectrum (loss of one Ar atom) of each mass-selected complex. In general, the (Ng-H-Ng+)Ngn consist (Giju et al., 2002; Ritschel et al., 2005, 2007; Császár et al., 2019) of Ng atoms weakly bound to a covalent centro-symmetric Ng2H +, the (Ng-H-Ng+)Ng5 growing by the step-by-step addition of five Ng in the plane perpendicular to the Ng-H-Ng+ axis (see Figure 1). The IR patterns of the gaseous (Ar-H-Ar+)Arn were assigned (McDonald et al., 2016) as the ν3 and (nν1 + ν3) progression, with n arriving up to 4 for (Ar-H-Ar+)Ar. As shown in Table 1, the ν3 (989 cm−1), (ν1 + ν3) (1,237 cm−1), and (2ν1 + ν3) (1,485 cm−1) absorptions of the latter species are, invariably, blue-shifted with respect to the corresponding values measured in argon matrix. Consistently, when going from (Ar-H-Ar+)Ar to (Ar-H-Ar+)Ar5, the ν3 and (ν1 + ν3) resulted progressively red-shifted up to 965 and 1,207 cm−1, respectively. A strictly similar trend was quite recently noticed by Asvany et al. (2019), who measured the ν3 of the gaseous (He-H-He+)Hen (n = 1–4) ranging between 1,290 (n = 1) and 1,273 cm−1 (n = 4). As for the larger (Ng-H-Ng+)Ngn (n > 5), the available experimental results (Kojima et al., 1992; Bartl et al., 2013; Gatchell et al., 2018, 2019; Lundberg et al., 2020) unravel intriguing differences between the heaviest (Ng-H-Ng+)Ngn (Ng = Ne, Ar, Kr), and the He congeners. For the former species, the mass-spectrometric abundancies (Gatchell et al., 2018, 2019) indicate the “magic” role of three structures, namely the (Ng-H-Ng+)Ng5, the (Ng-H-Ng+)Ng11, and the (Ng-H-Ng+)Ng17. Based also on theoretical calculations, these species are identified as the most stable intermediates along a icosahedral growth. Starting from the (Ng-H-Ng+)Ng5, five Ng atoms progressively bind to one of the two equivalent ends of the Ng-H-Ng+ core, and the ensuing ring is eventually “capped” by a further Ng. A second specular capped ring of six Ng atoms is then obtained around the other Ng of the core, and the cluster eventually looks like a “roller” made up of an axel with three wheels, the two outside ones having hubcaps (Ritschel et al., 2005). On the other hand, consistent with previous results (Kojima et al., 1992; Bartl et al., 2013), recent experiments (Lundberg et al., 2020) showed that, in the mass spectra of protonated helium clusters, the only observed magic structure associated with the icosahedral motif is the (Ng-H-Ng+)Ng11. The (Ng-H-Ng+)Ng5 and (Ng-H-Ng+)Ng17 are missing, but there is evidence for peculiarly stable (Ng-H-Ng+)Ng4 and (Ng-H-Ng+)Ng9, the latter being the strongest abundance anomaly. Recent theoretical calculations (Császár et al., 2019) actually suggest the stability of the (Ng-H-Ng+)Ng4, and the (Ng-H-Ng+)Ng11, but do not provide evidence for peculiarly stable (Ng-H-Ng+)Ng9. Clearly, there is still room for further investigation.
The Ng-H distances of the Ng-H-Ng+ are experimentally unknown, and Table 1 quotes accurate available theoretical predictions (Kim and Lee, 1999; Tan and Kuo, 2019). Likewise, the NgH+, the values increase from He-H-He+ to Xe-H-Xe+, but are comparatively longer than those of the diatomic ions. In fact, in the Ng-H-Ng+, the proton is “shared” between two equivalent Ng atoms. In any case, the bonding analysis (Borocci et al., 2011) is again suggestive of covalent bonds.
Mixed ions Ng-H-Ng'+ are still elusive in the gas phase, and the only experimentally-detected species is the Xe-H-Kr+ identified in solid hydrogen by a ν3 absorption at 1,284 cm−1 (Tsuge et al., 2015). Compared with the ν3 of Kr-H-Kr+ and Xe-H-Xe+ measured in the same environment (see Table 1), this value is blue-shifted, and this is in line with previous theoretical predictions (Lundell, 1995) showing that in the Xe-H-Kr+, the proton is more tightly bound to the Xe atom and the ion is best described as a (Xe-H+–Kr) complex with a ν3 nearer to, but smaller than, the stretching frequency of XeH+ (2270 cm−1). Indeed, an asymmetric structure is a common feature of the mixed Ng-H-Ng'+ (Fridgen and Parnis, 1998a,b; Lundell et al., 1999). The (formal) H+ is more tightly bound to the heaviest Ng, and the resonance structure (Ng-H+–Ng') becomes progressively prevailing by increasing the difference between the proton affinity (PA) of Ng and Ng' (Ng > Ng'). Simplest illustrative examples are the Ne-H-He+, Ar-H-He+, and Ar-H-Ne+ reported by Koner et al. (2012). The Ne-H (1.108 Å) and He-H (0.959 Å) distances of Ne-H-He+ are shorter and longer, respectively, than the bond distances of Ne-H-Ne+ (1.139 Å) and He-H-He+ (0.926 Å), and the loss of Ne from Ne-H-He+ resulted more endothermic than that of He (18.2 vs. 12.0 kcal mol−1). In addition, the ν3 absorption of Ne-H-He+, 1,644 cm−1, falls in between the value predicted for He-H-He+ (1,717 cm−1) and Ne-H-Ne+ (1,437 cm−1). In the Ar-H-He+ and Ar-H-Ne+, the structural asymmetries are so pronounced to support their description as complexes of ArH+ weakly bound to He and Ne (by ca. 2.0 and 4.1 kcal mol−1, respectively).
Are there prospects to detect the Ng-H-Ng+ and Ng-H-Ng'+ in outer space (Fortenberry, 2017; Stephan and Fortenberry, 2017)? Assuming that the extreme conditions of some dense regions of the interstellar medium and planetary atmospheres may be sufficient to produce not only ArH+ but also NeH+, Fortenberry (2017) suggested the conceivable formation of Ar-H-Ar+, Ne-H-Ne+, and Ar-H-Ne+ from the exothermic reaction of NgH+ with the H(Ng) (Ng = Ne, Ar) (vide infra) or with Ne or Ar atoms absorbed on polycyclic aromatic hydrocarbons (PAHs) (Rodríguez-Cantano et al., 2017). While the former process is probably hampered by the high barriers arising from the positive charges of the colliding partners, the PAHs route seems more plausible, especially in view of the predicted exothermic character of model reactions between NeH+ and C10H8-Ng or C32H14-Ng (Ng = Ne, Ar) (Fortenberry, 2017). Other suggestions for conceivable formation routes come from studies performed in cold matrices (Feldman et al., 2016; Saenko and Feldman, 2016), showing that ionized molecules such as H2O and CH3OH (quite abundant in the outer space) might protonate Ng atoms so to eventually form the Ng-H-Ng+.
(H)(Ng)n (n ≥ 1)
The NgH, particularly the lightest HeH, NeH, and ArH, have received sustained experimental and theoretical interest, also in connection with their role in reactions (3) and (4). Illustrative in this regard are, for example, the extensive studies on the reaction between H and He performed so far by Herman, Zülicke, and their coworkers (Schneider et al., 1976; Havemann et al., 1978, and references cited therein) with crossed-beam experiments and quasi-classical trajectory calculations. The NgH possess linear Ng-H-H+ connectivities, and their potential wells (see Table 1) are deep enough (Theis et al., 2015; Koner et al., 2019) to sustain numerous vibrational and rovibrational states. The latter were accurately estimated even recently (Theis et al., 2015; Papp et al., 2017, 2018; Szidarovszky and Yamanouchi, 2017), also in the intriguing prospect of actual detection of natural NgH. Some general warnings in this regard come, however, from the information already available, in particular on the gas-phase ion chemistry of Ar/H2 mixtures (Bedford and Smith, 1990; Hvistendahl et al., 1990; Koyanagi et al., 2000). The ArH was actually detected (Bedford and Smith, 1990) from the ligand-switching between Ar and H2, exothermic by ca. 17 kcal mol−1. However, dynamics calculations on the reaction between Ar and H (Liu et al., 2011; Hu et al., 2013) point to a direct reactive mechanism, and the experiments also showed (Bedford and Smith, 1990) that, at least down to 80 K, Ar+ reacts with H2 to directly from ArH+, with no evidence for ArH. But even assuming stabilization at the lowest temperatures, ArH could exothermically react with H2 (by ca. 8 kcal mol−1) to form ArH. The latter is stable enough to conceivably exist at the lowest temperatures of the interstellar medium (vide infra).
The NgH are the simplest members of the H(Ng)n family (n ≥ 1). The H(Ng)2 were, in particular, theoretically investigated by Krapp et al. (2008), who obtained evidence for linear symmetric complexes (Ng-H-H-Ng)+, thermochemically bound with respect to H and two Ng (see Table 1). However, this stability must contend with the rearrangement into Ng—NgH that resulted exothermic for Ng = Xe, and very fast for Ng = He and Ne. Therefore, the experiments were oriented to search the argon and krypton congeners; however, no Ar2H or Kr2H were detected from ionized Ng/H2 mixtures (Krapp et al., 2008). Somewhat unexpectedly, the lightest H(He)2 was instead observed by electron ionization of H2-doped helium droplets soon afterward (Jaksch et al., 2009). Besides the expected dissociation into HeH and He, featuring a low kinetic energy release (KER) of 15 ± 4 meV, the mass-analyzed kinetic energy experiments unraveled a not-surmised dissociation into HeH+ and HeH (or He + H), occurring with a higher probability, and a KER four times larger than that of the loss of He. This behavior was ascribed to a metastable, electronically excited H(He)2, whose excess energy arising from the ionization event allows the rupture of the stronger H-H+ bond, the weaker He-H+ remaining intact. Larger H(He)n (n ≤ 30) were subsequently detected from ionized helium droplets doped with H2 (Bartl et al., 2013), but their structure is only little explored.
(H)(Ng)n (n ≥ 1)
The H(Ng)n generally consist of a H covalent ionic core weakly bound to one or more Ng atoms. According to the calculations (Beyer et al., 1999; Kaczorowska et al., 2000), the first three Ng add to the vertices of the equilateral H, and the fourth and the fifth ones complete the bi-pyramidal structure shown in Figure 1. The vertex-coordination of H(Ar) is also supported by spectroscopic measurements (Bogey et al., 1987, 1988; Bailleux et al., 1998; McCarthy and Thaddeus, 2010). As shown in Table 1, the addition of one Ng to H is, invariably, exothermic, the stability increasing when going from H(He) to H(Xe) (Savić et al., 2015; Pauzat et al., 2016). Interestingly, as shown by the calculations (Beyer et al., 1999; Mousis et al., 2008), this trend mirrors a structure of the H(Ng) that gradually changes from nearly pure H(He) and H(Ne) to a description close to XeH+(H2). This parallels a PA of H2, 100.9 kcal mol−1, that is lower than the PA of Xe (119.4 kcal mol−1). In essence, the periodic increase of the stabilities of the H(Ng) reflects not only the increase of the polarizability of Ng (with consequent increase of the ensuing charge transfer to H), but also the onset of covalency in the Kr-H and especially the Xe-H bonds. The calculations indicate also (Pauzat and Ellinger, 2005; Pauzat et al., 2009; Chakraborty et al., 2010) that, when going to the larger H(Ng)n (n ≥ 2), the energy change of the reaction H(Ng)n → H(Ng)n−1 + Ng tends to decrease by increasing n, appreciable jumps in particular being predicted between n = 1 and n = 2, and between n = 3 and n = 4. These trends are well-consistent with the experimental binding energies of H(Ar)n (n = 1-7), measured (Hiraoka and Mori, 1989) as 6.7 (n = 1), 4.6 (n = 2), 4.3 (n = 3), 2.5 (n = 4), 2.3 (n = 5), 2.2 (n = 6), and 1.6 kcal mol−1 (n = 7), and clearly mirror the growing mode of the cluster.
The vibrational and rotational patterns of the H(Ar) and H(Ne) were more recently refined by theoretical calculations (Theis and Fortenberry, 2015), performed also to best guide the conceivable detection of these ions in the outer space. The natural role of the H(Ng)n was first suggested by Pauzat, Ellinger, and their coworkers, who proposed that the deficit of noble gases observed in planetary objects could be due to the sequestration by H during the early stages of the solar nebula (Pauzat and Ellinger, 2005, 2007; Mousis et al., 2008; Pauzat et al., 2009, 2013). According to their recent quantum-dynamics calculations (Pauzat et al., 2016), especially for the heaviest Kr and Xe, the rate constants of the radiative association are indeed large enough to support the sequestration by H. The helium complexes H(He)n are also of potential astrochemical interest. Theoretical calculations (Chakraborty et al., 2010) confirm the planar structure of the H(He)3, the three He bound to the vertices of H featuring nearly constant complexation energies of ca. 1 kcal mol−1. On the experimental side, Gerlich and his coworkers (Savić et al., 2015) recently produced H(He)n (n up to 9) in an ion trap cooled down to 3.7 K. Particularly for the simplest H(He), laser-induced dissociation experiments unraveled almost 100 lines between 2,700 and 2,765 cm−1, whose detailed assignment was, however, hampered by the lack of a sufficiently accurate potential energy surface. Species with higher n are also detected in different ion sources (Kojima et al., 1992; Bartl et al., 2013), the experiments suggesting, in particular, the peculiar stability of the H(He)12, and the “magic” role of n = 9 and 10. Further structural assay awaits more detailed theoretical investigations.
Finally, it is worth mentioning a group of complexes of the NgH+ with simple molecules such as N2, CO, SiO, CS, BF, N2, and H2O investigated by Ghanty and his coworkers (Jayasekharan and Ghanty, 2008, 2012; Ghosh et al., 2013, 2014; Sirohiwal et al., 2013; Sekhar et al., 2015). The HNgNH were as well-reported (Gao and Sheng, 2015). The most stable complex between NgH+ and a ligand L is the Ng-H-L+, the proton being typically more tightly bound to L (the PA of most molecules is, in fact, higher than that of the Ng atoms). In any case, the thermochemically less stable H-Ng-L+, best described by the limiting resonance structure (HNg+)L, is bound with respect to NgH+ + L, and kinetically protected toward the fast decomposition into Ng + LH+. Whether these conditions are sufficient for their formation in the outer space is another intriguing question related to the fascinating gas-phase chemistry of cationic noble-gas hydrides.
Author Contributions
The author made a direct intellectual contribution to the work.
Funding
This work was supported by the Departments of Excellence-2018 Program (Dipartimenti di Eccellenza) of the Italian Ministry of Education, University and Research, DIBAF—Department of University of Tuscia, Project Landscape 4.0—food, wellbeing and environment.
Conflict of Interest
The author declares that the research was conducted in the absence of any commercial or financial relationships that could be construed as a potential conflict of interest.
References
Asvany, O., Schlemmer, S., Szidarovszky, T., and Császár, A. G. (2019). Infrared signatures of the HHe and DHe (n = 3–6) complexes. J. Phys. Chem. Lett. 10, 5325–5330. doi: 10.1021/acs.jpclett.9b01911
Bailleux, S., Bogey, M., Bolvin, H., Civiš, S., Cordonnier, M., Krupnov, A. F., et al. (1998). Sub-Millimeter-Wave spectroscopy of the Ar·H and Ar·D ionic complexes. J. Mol. Spectrosc. 190, 130–139. doi: 10.1006/jmsp.1998.7564
Barlow, M. J., Swinyard, B. M., Owen, P. J., Cernicharo, J., Gomez, H. L., Ivison, R. J., et al. (2013). Detection of a noble gas molecular ion, 36ArH+, in the Crab nebula. Science 342, 1343–1345. doi: 10.1126/science.1243582
Bartl, P., Leidlmair, C., Denifl, S., Scheier, P., and Echt, O. (2013). Cationic complexes of hydrogen with helium. ChemPhysChem 14, 227–232. doi: 10.1002/cphc.201200664
Bedford, D. K., and Smith, D. (1990). Variable-temperature selected ion flow tube studies of the reactions of Ar+., Ar. and ArH (n = 1-3) with H2, HD and D2 at 300 K and 80 K. Int. J. Mass Spectrom. Ion Processes 98, 179–190. doi: 10.1016/0168-1176(90)85017-V
Beyer, M., Savchenko, E. V., Niedner-Scatteburg, G., and Bondybey, V. E. (1999). Trihydrogen cation solvated by rare gas atoms: RgnH. J. Chem. Phys. 110, 11950–11957. doi: 10.1063/1.479134
Bogey, M., Bolvin, H., Demuynck, C., and Destombes, J. L. (1987). High-resolution rotational spectroscopy of weakly bound ionic clusters: ArH, ArD. Phys. Rev. Lett. 58, 988–991. doi: 10.1103/PhysRevLett.58.988
Bogey, M., Bolvin, H., Demuynck, C., Destombes, J. L., and Van Eijck, B. P. (1988). Tunneling motion in ArH and isotopomers from the analysis of their rotational spectra. J. Chem. Phys. 88, 4120–4126. doi: 10.1063/1.453819
Bop, C. T., Hammami, K., and Faye, N. A. B. (2017b). Collisional rates based on the first potential energy surface of the NeH+-He system. Mon. Not. R. Astron. Soc. 470, 2911–2917. doi: 10.1093/mnras/stx1369
Bop, C. T., Hammami, K., Niane, A., Faye, N. A. B., and Jaïdane, N. (2017a). Rotational excitation of 36ArH+ by He at low temperature. Mon. Not. R. Astron. Soc. 465, 1137–1143. doi: 10.1093/mnras/stw2809
Borocci, S., Giordani, M., and Grandinetti, F. (2011). Cationic noble gas hydrides-2: a theoretical investigation on HNgHNgH+ (Ng = Ar, Kr, Xe). Comput. Theor. Chem. 964, 318–323. doi: 10.1016/j.comptc.2011.01.018
Borocci, S., Giordani, M., and Grandinetti, F. (2015). Bonding motifs of noble-gas compounds as described by the local electron energy density. J. Phys. Chem. A 119, 6528–6541. doi: 10.1021/acs.jpca.5b03043
Cecchi-Pestellini, C., and Dalgarno, A. (1993). Emission of HeH+ in nebulae. Astrophys. J. 413, 611–618.
Chakraborty, A., Giri, S., and Chattaraj, P. K. (2010). Trapping of noble gases (He-Kr) by the aromatic H and Li species: a conceptual DFT approach. New J. Chem. 34, 1936–1945. doi: 10.1039/C0NJ00040J
Coxon, J. A., and Hajigeorgiou, P. G. (1999). Experimental Born-Hoppenheimer potential for the X1∑+ ground state of HeH+: comparison with the Ab Initio potential. J. Mol. Spectrosc. 193, 306–318. doi: 10.1006/jmsp.1998.7740
Császár, A. G., Szidarovszky, T., Asvany, O., and Schlemmer, S. (2019). Fingerprints of microscopic superfluidity in HHe clusters. Mol. Phys. 117, 1559–1583. doi: 10.1080/00268976.2019.1585984
Fárník, M., and Toennies, J. P. (2005). Ion-molecule reactions in 4He droplets: flying nano-cryo-reactors. J. Chem. Phys. 122, 014307. doi: 10.1063/1.1815272
Feldman, V. I., Ryazantsev, S. V., Saenko, E. V., Kameneva, S. V., and Shiryaeva, E. S. (2016). Matrix isolation model studies on the radiation-induced transformations of small molecules of astrochemical and atmospheric interest. Radiat. Phys. Chem. 124, 7–13. doi: 10.1016/j.radphyschem.2015.12.005
Fortenberry, R. C. (2017). Rovibrational characterization and interstellar implications of the proton-bound, noble gas complexes: ArHAr+, NeHNe+, and ArHNe+. ACS Earth Space Chem. 1, 60–69. doi: 10.1021/acsearthspacechem.7b00003
Fortenberry, R. C. (2019). The oldest molecular ancestor finally brought into the light. Chem 5, 1028–1030. doi: 10.1016/j.chempr.2019.04.016
Fridgen, T. D., and Parnis, J. M. (1998a). Electron bombardment matrix isolation of Rg/Rg'/methanol mixtures (Rg = Ar, Kr, Xe): fourier-transform infrared characterization of the proton-bound dimers Kr2H+, Xe2H+, (ArHKr)+ and (ArHXe)+ in Ar matrixes and (KrHXe)+ and Xe2H+ in Kr matrixes. J. Chem. Phys. 109, 2155–2161. doi: 10.1063/1.476728
Fridgen, T. D., and Parnis, J. M. (1998b). Density functional theory study of the proton-bound rare-gas dimers Rg2H+ and (RgHRg')+ (Rg = Ar, Kr, Xe): interpretation of experimental matrix isolation infrared data. J. Chem. Phys. 109, 2162–2168. doi: 10.1063/1.476729
Gao, K., and Sheng, L. (2015). Theoretical investigation of HNgNH ions (Ng = He, Ne, Ar, Kr, and Xe). J. Chem. Phys. 142:144301. doi: 10.1063/1.4916648
Gatchell, M., Martini, P., Kranabetter, L., Rasul, B., and Scheier, P. (2018). Magic sizes of cationic and protonated argon clusters. Phys. Rev. A 98:022519. doi: 10.1103/PhysRevA.98.022519
Gatchell, M., Martini, P., Schiller, A., and Scheier, P. (2019). Protonated clusters of neon and krypton. J. Am. Soc. Mass Spectrom. 30, 2632–2636. doi: 10.1021/jasms.8b06284
Ghosh, A., Manna, D., and Ghanty, T. K. (2013). Theoretical prediction of rare gas inserted hydronium ions: HRgOH. J. Chem. Phys. 138:194308. doi: 10.1063/1.4804623
Ghosh, A., Manna, D., and Ghanty, T. K. (2014). Theoretical prediction of noble gas inserted thioformyl cations: HNgCS+ (Ng = He, Ne, Ar, Kr, and Xe). J. Phys. Chem. A 119, 2233–2243. doi: 10.1021/jp5042266
Giese, C. F., and Maier, W. B. II. (1961). Ion-molecule reactions studied with mass analysis of primary ion beam. J. Chem. Phys. 35, 1913–1914. doi: 10.1063/1.1732184
Giju, K. T., Roszak, S., and Leszczynski, J. (2002). A theoretical study of protonated argon clusters: ArnH+ (n = 1-7). J. Chem. Phys. 117, 4803–4809. doi: 10.1063/1.1485956
Grandinetti, F. (2011). Gas-phase ion chemistry of the noble gases: recent advances and future perspectives. Eur. J. Mass Spectrom. 17, 423–463. doi: 10.1255/ejms.1151
Grandinetti, F. (2018). Noble Gas Chemistry, Structure, Bonding, and Gas-Phase Chemistry. Weinheim: Wiley, VCH.
Gruet, S., and Pirali, O. (2019). Far-infrared spectroscopy of heavy protonated noble gas species using synchrotron radiation. Mol. Phys. 117, 1719–1731. doi: 10.1080/00268976.2018.1564851
Güsten, R., Wiesemeyer, H., Neufeld, D., Menten, K. M., Graf, U. U., Jacobs, K., et al. (2019). Astrophysical detection of the helium hydride ion HeH+. Nature 568, 357–362. doi: 10.1038/s41586-019-1090-x
Havemann, U., Pacák, V., Herman, Z., Schneider, F., Zuhrt, C., and Zülicke, L. (1978). Dissociation in collisions of H with He in the eV region. crossed-beam experiments and quasi-classical trajectory calculations. Chem. Phys. 28, 147–54. doi: 10.1016/0301-0104(78)85045-9
Hiraoka, K., and Mori, T. (1989). Isotope effect and nature of bonding in the cluster ions H(Ar)n and D(Ar)n. J. Chem. Phys. 91, 4821–4826. doi: 10.1063/1.456720
Hogness, T. R., and Lunn, E. G. (1925). The ionization of hydrogen by electron impact as interpreted by positive ray analysis. Phys. Rev. 26, 44–55. doi: 10.1103/PhysRev.26.44
Hu, M., Xu, W., Liu, X., Tan, R., and Li, H. (2013). Time-dependent quantum wave packet study of the Ar + H → ArH+ + H reaction on a new ab initio potential energy surface for the ground electronic state (12A'). J. Chem. Phys. 138:174305. doi: 10.1063/1.4803116
Hvistendahl, G., Saastad, O. W., and Uggerud, E. (1990). Ion/molecule reactions in a mixture of Ar and H2: high pressure mass spectrometry and quantum chemical calculations. Int. J. Mass Spectrom. Ion Processes 98, 167–177. doi: 10.1016/0168-1176(90)85016-U
Jaksch, S., da Silva, F. F., Denifl, S., Echt, O., Märk, T. D., and Scheier, P. (2009). Experimental evidence for the existence of an electronically excited state of the proposed dihydrogen radical cation He-H-H-He+. Chem. Eur. J. 15, 4190–4194. doi: 10.1002/chem.200802545
Jašik, J., Žabka, J., Roithová, J., and Gerlich, D. (2013). Infrared spectroscopy of trapped molecular dications below 4 K. Int. J. Mass Spectrom. 354-355, 204–210. doi: 10.1016/j.ijms.2013.06.007
Jayasekharan, T., and Ghanty, T. K. (2008). Theoretical prediction of HRgCO+ ion (Rg = He, Ne, Ar, Kr, and Xe). J. Chem. Phys. 129:184302. doi: 10.1063/1.3008057
Jayasekharan, T., and Ghanty, T. K. (2012). Theoretical investigation of rare gas hydride cations: HRgN (Rg = He, Ar, Kr, and Xe). J. Chem. Phys. 136:164312. doi: 10.1063/1.4704819
Kaczorowska, M., Roszak, S., and Leszczynski, J. (2000). The structure and properties of HArn (n = 1-9) cations. J. Chem. Phys. 113, 3615–3620. doi: 10.1063/1.1287831
Kim, S. T., and Lee, J. S. (1999). Ab initio study of He2H+ and Ne2H+: accurate structure and energetics. J. Chem. Phys. 110, 4413–4418. doi: 10.1063/1.478324
Kojima, T. M., Kobayashi, N., and Kaneko, Y. (1992). Formation of helium cluster ions HHe (x ≤ 14) and H3He (x ≤ 13) in a very low temperature drift tube. Z. Phys. D –Atoms Mol. Clust. 23, 181–185. doi: 10.1007/BF01436742
Koner, D., Vats, A., Vashishta, M., and Panda, A. N. (2012). Ab initio electronic structure investigation of protonated mixed rare gas dimers [NeHHe]+, [ArHHe]+ and [ArHNe]+. Comput. Theor. Chem. 1000, 19–25. doi: 10.1016/j.comptc.2012.09.004
Koner, D., Veliz, J. C. S. V., van der Avoird, A., and Meuwly, M. (2019). Near dissociation states for H-He on MRCI and FCI potential energy surfaces. Phys. Chem. Chem. Phys. 21, 24976–24983. doi: 10.1039/C9CP05259C
Koyanagi, G. K., Lavrov, V. V., Baranov, V., Bandura, D., Tanner, S., McLaren, J. W., et al. (2000). A novel inductively coupled plasma/selected-ion flow tube mass spectrometer for the study of reactions of atomic and atomic oxide ions. Int. J. Mass Spectrom. 194, L1–L5. doi: 10.1016/S1387-3806(99)00233-X
Krapp, A., Frenking, G., and Uggerud, E. (2008). Nonpolar dihydrogen bonds - on a gliding scale from weak dihydrogen interaction to covalent H-H in symmetric radical cations [HnE-H-H-EHn]+. Chem. Eur. J. 14, 4028–2038. doi: 10.1002/chem.200701613
Kunttu, H., Seetula, J., Rasanen, M., and Apkarian, V. A. (1992). Photogeneration of ions via delocalized charge transfer states. I. Xe2H+ and Xe2D+ in solid Xe. J. Chem. Phys. 96, 5630–5635. doi: 10.1063/1.462687
Kunttu, H. M., and Seetula, J. A. (1994). Photogeneration of ionic species in Ar, Kr and Xe matrices doped with HCl, HBr and HI. Chem. Phys. 189, 273–292. doi: 10.1016/0301-0104(94)00273-8
Liu, X., Liu, H., and Zhang, Q. (2011). An ab initio potential energy surface and dynamics of the Ar + H → ArH(+) + H reaction. Chem. Phys. Lett. 507, 24–28. doi: 10.1016/j.cplett.2011.03.021
Lundberg, L., Bartl, P., Leidlmar, C., Scheier, P., and Gatchell, M. (2020). Protonated and cationic helium clusters. Molecules 25:1066. doi: 10.3390/molecules25051066
Lundell, J. (1995). Density functional approach on ground state RgH+ and RgHRg+ (Rg = Ar, Kr, Xe) ions. J. Mol. Struct. 355, 291–297. doi: 10.1016/0022-2860(95)08916-J
Lundell, J., Pettersson, M., and Räsänen, M. (1999). The proton-bound rare gas compounds (RgHRg')+ (Rg = Ar, Kr, Xe) - a computational approach. Phys. Chem. Chem. Phys. 1, 4151–4155. doi: 10.1039/A904242C
McCarthy, M. C., and Thaddeus, P. (2010). High-resolution rotational spectroscopy of NNOH+, DCS+, Ar···D, Ar···DCO+, and Ar···HN. J. Mol. Spectrosc. 263, 71–77. doi: 10.1016/j.jms.2010.06.006
McDonald, D. C. II., Mauney, D. T., Leicht, D., Marks, J. H., Tan, J. A., Kuo, J.-L., et al. (2016). Communication: trapping a proton in argon: spectroscopy and theory of the proton-bound argon dimer and its solvation. J. Chem. Phys. 145:231101. doi: 10.1063/1.4972581
Mousis, O., Pauzat, F., Ellinger, Y., and Ceccarelli, C. (2008). Sequestration of noble gases by H in protoplanetary disks and outer solar system composition. Astrophys. J. 673, 637–646. doi: 10.1086/523925
Müller, H. S. P., Muller, S., Schilke, P., Bergin, E. A., Black, J. H., Gerin, M., et al. (2015). Detection of extragalactic argonium, ArH+, toward PKS 1830-211. Astron. Astrophys. 582, L4. doi: 10.1051/0004-6361/201527254
National Institute of Standards and Technology (2020) NIST Chemistry WebBook, NIST Standard Reference Database Number. eds P. J. Linstrom and W. G. Mallard (Gaithersburg MD: National Institute of Standards and Technology).
Papp, D., Császár, A. G., Yamanouchi, K., and Szidarovszky, T. (2018). Rovibrational resonances in H2He+. J. Chem. Theory Comput. 14, 1523–1533. doi: 10.1021/acs.jctc.7b01136
Papp, D., Szidarovszky, T., and Császár, A. G. (2017). A general variational approach for computing rovibrational resonances of polyatomic molecules. application to the weakly bound H2He+ and H2·CO systems. J. Chem. Phys. 147:094106. doi: 10.1063/1.5000680
Pauzat, F., Bacchus-Montabonel, M.-C., Ellinger, Y., and Mousis, O. (2016). Trapping of noble gases by radiative association with H in the protosolar nebula. Astrophys. J. Lett. 821:L33. doi: 10.3847/2041-8205/821/2/L33
Pauzat, F., and Ellinger, Y. (2005). H as a trap for noble gases: 1- the case of argon. Planet. Space Sci. 53, 1389–1399. doi: 10.1016/j.pss.2005.07.005
Pauzat, F., and Ellinger, Y. (2007). H as a trap for noble gases-2: structure and energetics of XH complexes from X = neon to xenon. J. Chem. Phys. 127:014308. doi: 10.1063/1.2746033
Pauzat, F., Ellinger, Y., Mousis, O., Dib, M. A., and Ozgurel, O. (2013). Gas-phase sequestration of noble gases in the protosolar nebula: possible consequences on the outer solar system composition. Astrophys. J. 777:29. doi: 10.1088/0004-637X/777/1/29
Pauzat, F., Ellinger, Y., Pilmé, J., and Mousis, O. (2009). H as a trap for noble gases-3: multiple trapping of neon, argon, and krypton in XnH (n = 1-3). J. Chem. Phys. 130:174313. doi: 10.1063/1.3126777
Perry, A. J., Hodges, J. N., Markus, C. R., Kocheril, G. S., and McCall, B. J. (2014). Communication: high precision sub-Doppler infrared spectroscopy of the HeH+ ion. J. Chem. Phys. 141:101101. doi: 10.1063/1.4895505
Rayleigh, and Ramsay, W. (1895). Argon, a new constituent of the atmosphere. Philos. Trans. R. Soc. London A 186, 187–241.
Ritschel, T., Kuntz, P. J., and Zülicke, L. (2005). Structure and dynamics of cationic van-der-Waals clusters. Eur. Phys J. 33, 421–432. doi: 10.1140/epjd/e2005-00070-4
Ritschel, T., Zuelicke, L., and Kuntz, P. J. (2004). Cationic van-der-Waals complexes: Theoretical study of Ar2H+ structure and stability. Z. Phys. Chem. 218, 377–390. doi: 10.1524/zpch.218.4.377.29196
Ritschel, T., Zuhrt, C., Zülicke, L., and Kuntz, P. J. (2007). Structure and dynamics of cationic van-der-Waals clusters. Eur. Phys J. D 41, 127–141. doi: 10.1140/epjd/e2006-00191-2
Roberge, W., and Dalgarno, A. (1982). The formation and destruction of HeH+ in astrophysical plasmas. Astrophys. J. 255, 489–496.
Rodríguez-Cantano, R., Bartolomei, M., Hernández, M. I., Campos-Martínez, J., González-Lezana, T., Villarreal, P., et al. (2017). Comparative investigation of pure and mixed rare gas atoms on coronene molecules. J. Chem. Phys. 146:034302. doi: 10.1063/1.4973890
Rogers, S. A., Brazier, C. R., and Bernath, P. F. (1987). The infrared spectrum of XeH+. J. Chem. Phys. 87, 159–162. doi: 10.1063/1.453611
Saenko, E. V., and Feldman, V. I. (2016). Radiation-induced transformations of methanol molecules in low-temperature solids: a matrix isolation study. Phys. Chem. Chem. Phys. 18, 32503–32513. doi: 10.1039/c6cp06082j
Savić, I., Gerlich, D., Asvany, O., Jusko, P., and Schlemmer, S. (2015). Controlled synthesis and analysis of He-H in a 3.7 K ion trap. Mol. Phys. 113, 2320–2332. doi: 10.1080/00268976.2015.1037802
Schilke, P., Neufeld, D. A., Müller, H. S. P., Comito, C., Bergin, E. A., Lis, D. C., et al. (2014). Ubiquitous argonium (ArH+) in the diffuse interstellar medium: a molecular tracer of almost purely atomic gas. Astron. Astrophys. 566:A29. doi: 10.1051/0004-6361/201423727
Schneider, F., Havemann, U., Zülicke, L., Pacák, V., Birkinshaw, K., and Herman, Z. (1976). Dynamics of the reaction H(He;H)HeH+. comparison of beam experiments with quasi-classical trajectory studies. Chem. Phys. Lett. 37, 323–328. doi: 10.1016/0009-2614(76)80225-4
Sekhar, P., Ghosh, A., and Ghanty, T. K. (2015). Noble gas inserted protonated silicon monoxide cations: HNgOSi+ (Ng = He, Ne, Ar, Kr, and Xe). J. Phys. Chem. A 119, 11601–11613. doi: 10.1021/acs.jpca.5b09018
Sirohiwal, A., Manna, D., Ghosh, A., Jayasekharan, T., and Ghanty, T. K. (2013). Theoretical prediction of rare gas containing hydride cations: HRgBF+ (Rg = He, Ar, Kr, and Xe). J. Phys. Chem. A 117, 10772–10782. doi: 10.1021/jp4064824
Stephan, C. J., and Fortenberry, R. C. (2017). The interstellar formation and spectra of the noble gas, proton-bound HeHHe+, HeHNe+ and HeHAr+ complexes. Mon. Not. R. Astron. Soc. 469, 339–346. doi: 10.1093/mnras/stx937
Stevenson, D. P. (1957). Ion-molecule reactions. J. Phys. Chem. 61, 1453–1456. doi: 10.1021/j150557a001
Szidarovszky, T., and Yamanouchi, K. (2017). Full-dimensional simulation of the laser-induced alignment dynamics of H2He+. Mol. Phys. 115, 1916–1926. doi: 10.1080/00268976.2017.1297863
Tan, J. A., and Kuo, J.-L. (2019). A theoretical study on the infrared signatures of proton-bound rare gas dimers (Rg–H+-Rg), Rg = {Ne, Ar, Kr, and Xe}. J. Chem. Phys. 150:124305. doi: 10.1063/1.5090031
Theis, R. A., and Fortenberry, R. C. (2015). Trihydrogen cation with neon and argon: structural, energetic, and spectroscopic data from quartic force fields. J. Phys. Chem. A 119, 4915–4922. doi: 10.1021/acs.jpca.5b03058
Theis, R. A., Morgan, W. J., and Fortenberry, R. C. (2015). ArH and NeH as global minima in the Ar+/Ne+ + H2 reactions: energetic, spectroscopic, and structural data. Mon. Not. R. Astron. Soc. 446, 195–204. doi: 10.1093/mnras/stu1785
Keywords: noble-gas ions, noble-gas chemistry, interstellar chemistry, gas-phase chemistry, mass spectrometry, theoretical calculations
Citation: Grandinetti F (2020) Cationic Noble-Gas Hydrides: From Ion Sources to Outer Space. Front. Chem. 8:462. doi: 10.3389/fchem.2020.00462
Received: 23 February 2020; Accepted: 04 May 2020;
Published: 19 June 2020.
Edited by:
Sudip Pan, University of Marburg, GermanyReviewed by:
Vladimir Feldman, Lomonosov Moscow State University, RussiaAntonio Frontera, University of the Balearic Islands, Spain
Gabriel Merino, Centro de Investigacion y de Estudios Avanzados - Unidad Mérida, Mexico
Copyright © 2020 Grandinetti. This is an open-access article distributed under the terms of the Creative Commons Attribution License (CC BY). The use, distribution or reproduction in other forums is permitted, provided the original author(s) and the copyright owner(s) are credited and that the original publication in this journal is cited, in accordance with accepted academic practice. No use, distribution or reproduction is permitted which does not comply with these terms.
*Correspondence: Felice Grandinetti, ZmdyYW5kaSYjeDAwMDQwO3VuaXR1cy5pdA==
†ORCID: Felice Grandinetti orcid.org/0000-0001-7217-9490