- Energy Storage Research Group, Faculty of Ocean Engineering Technology and Informatics, Universiti Malaysia Terengganu, Terengganu, Malaysia
In this study, the modification of the desorption behavior of LiAlH4 by the addition of K2NbF7 was explored for the first time. The addition of K2NbF7 causes a notable improvement in the desorption behavior of LiAlH4. Upon the addition of 10 wt.% of K2NbF7, the desorption temperature of LiAlH4 was significantly lowered. The desorption temperature of the LiAlH4 + 10 wt.% K2NbF7 sample was lowered to 90°C (first-stage reaction) and 149°C (second-stage reaction). Enhancement of the desorption kinetics performance with the LiAlH4 + 10 wt.% K2NbF7 sample was substantiated, with the composite sample being able to desorb hydrogen 30 times faster than did pure LiAlH4. Furthermore, with the presence of 10 wt.% K2NbF7, the calculated activation energy values for the first two desorption stages were significantly reduced to 80 and 86 kJ/mol; 24 and 26 kJ/mol lower than the as-milled LiAlH4. After analysis of the X-ray diffraction result, it is believed that the in situ formation of NbF4, LiF, and K or K-containing phases that appeared during the heating process promoted the amelioration of the desorption behavior of LiAlH4 with the addition of K2NbF7.
Introduction
The excessive consumption of fossil fuels and the emission of carbon dioxide are the roots of environmental pollution. As a resolution to this global issue, the utilization of clean, and sustainable energy resources such as hydrogen, wind, and solar has become an inescapable need. Recently, hydrogen has received a large amount of attention as a future energy carrier. Hydrogen promises to be a clean and renewable energy carrier. Moreover, the production of hydrogen can be achieved from various resources, both renewable (e.g., solar, wind, and hydro) and non-renewable (e.g., natural gas and coal; Winter, 2009; Parra et al., 2019). Furthermore, energy production via hydrogen-oxygen reaction will only produce water as a by-product (Crabtree et al., 2004).
In pursuit of the success of hydrogen as a future energy carrier, the need for an efficient and reliable storage method has become the top priority. In general, there are three forms of hydrogen storage which are: (i) compressed hydrogen gas, which requires high pressure, (ii) liquefaction, and (iii) solid-state hydrogen storage via hydrides (Dalebrook et al., 2013; Zhang et al., 2016; Barthelemy et al., 2017). Solid-state hydrogen storage has been perceived to be an efficient and favorable method because of its safety, storage requirements, and storage capacity.
Lithium aluminum hydride (LiAlH4) has major benefits and is the preferable solid-state material. LiAlH4 is attractive due to its low temperature of hydrogen release and high storage capacity (10.6 wt.%; Andrei et al., 2005; Ares et al., 2008). The desorption process of LiAlH4 occurs in three stages, as follows:
The first reaction (1) occurs in a temperature range of 150–175°C and desorbs 5.2 wt.% of the hydrogen. The second reaction (2) takes places at 180–220°C and desorbs 2.6 wt.% of the hydrogen, while the third reaction (3) happens at temperatures > 400°C, with 2.6 wt.% of the hydrogen desorbed.
In spite of its advantages, LiAlH4 has some shortcomings, such as irreversible and slow desorption kinetics (Pukazhselvan et al., 2012). Moreover, the thermal decomposition in reaction 3 is considered incompatible with applied applications due to its high requirement for temperature (>400°C) to release hydrogen. Tremendous efforts have been devoted to overcoming the shortcomings of LiAlH4, such as the implementation of the ball milling method (Balema et al., 2000, 2001; Liu et al., 2009) and impurity-doping with various catalysts such as metals (Resan et al., 2005; Xueping et al., 2009; Langmi et al., 2010; Varin and Parviz, 2012), metal oxides (Zhai et al., 2012; Li Z. et al., 2013; Li et al., 2014; Liu et al., 2014; Sulaiman and Ismail, 2017; Ali et al., 2019; Sazelee et al., 2019), Ti-based additives (Ismail et al., 2011; Amama et al., 2012; Wohlwend et al., 2012; Li L. et al., 2013), and metal halides (Fernandez et al., 2007; Suttisawat et al., 2007; Xueping et al., 2007; Sun et al., 2008; Li et al., 2012).
Among these catalysts, previous studies have revealed that metal halides provide essential catalytic effects on the performance of LiAlH4. Cao et al. (2018) reported that the addition of ScCl3 had a superior effect on the performance of lithium alanates. The desorption process of the LiAlH4-10 mol% ScCl3 sample began at a lower temperature (~120°C), while the undoped LiAlH4 released hydrogen from around 150°C. Besides, the time needed to complete the dehydrogenation process was shortened with the addition of 1–10 mol% ScCl3. Meanwhile, Sun et al. (2008) found that NiCl2 significantly boosted the desorption behavior of LiAlH4. A composite sample of LiAlH4-NiCl2 demonstrated three times the desorption rate of pure LiAlH4, which was not able to desorb any hydrogen at 100°C. It was believed that the LiAlH4-NiCl2 sample presented this notable improvement due to the formation of Ni, which plays a vital role in accelerating the LiAlH4-NiCl2 system. Another investigation of the catalytic effect of metal halides was carried out by Liu et al. (2012). They proved that a LiAlH4-TiCl3 sample could release hydrogen at a lower temperature (80°C) than the pure LiAlH4. Furthermore, the dehydrogenated sample had good cyclability, with the composite sample able to retain a high capacity for hydrogen (6.4 wt.%) even after completing the 3rd cycle. Moreover, Ismail et al. (2010) observed that the composite sample of LiAlH4-1 mol NbF5 showed a 5–6 times faster dehydrogenation rate than the milled LiAlH4. Additionally, the LiAlH4-NbF5 composite sample had lower activation energy; 67 kJ/mol (first-stage reaction) and 77 kJ/mol (second-stage reaction), respectively. However, the improvement of LiAlH4 through the addition of a catalyst is still lacking, and further enhancements still need to be carried out. Moreover, different catalysts will enable different effects and performances. Therefore, it is interesting to enhance the desorption performance of LiAlH4 by the addition of other metal halides.
Metal halides, especially fluorides, are known to be highly effective catalysts for solid-state materials (Sulaiman et al., 2016; Yap et al., 2017; Youn et al., 2017). A number of researchers have reported that niobium fluoride exhibits a notable effect on the hydrogenation behavior of metal hydrides and the complex hydrides. A study conducted by Luo et al. (2008) revealed that the addition of 2 mol of NbF5 led to faster desorption kinetics for the MgH2-NbF5 sample as compared to pure MgH2. At 573 K, the MgH2-NbF5 sample could desorb 4.7 wt.% of hydrogen, while the pristine MgH2 desorbed almost no hydrogen. Other than that, Kou et al. (2014) added NbF5 to LiBH4 and demonstrated notable improvement on the desorption performance of LiBH4. In comparison to the milled LiBH4, which started to desorbed hydrogen at >400°C, the composite sample of LiBH4-NbF5 had a lower desorption temperature, 60°C. Moreover, Wang and colleagues (Wang et al., 2020) showed that a composite of Mg(BH4)2-doped NbF5 possessed the best dehydrogenation performance, with the ability to release hydrogen at low temperature (120°C), as compared to amorphous Mg(BH4)2 (126.9°C), and pristine Mg(BH4)2 (282.7°C). Meanwhile, Cheng et al. (2018) demonstrated that upon the addition of NbF5, the composite of 4LiBH4-MgH2-Al exhibited excellent kinetics and reversibility performance. It took <4 h to achieve 90% of the total amount of hydrogen desorption. Other than that, Xiao et al. (2012) proved that the performance of LiBH4/MgH2 was significantly improved with the addition of NbF5. Here, the addition of NbF5 not only had reduced the onset decomposition temperature but also improved the dehydrogenation and absorption rates.
On the other hand, potassium (K) is another well-known additive for hydrogen storage systems. Wang et al. (2009) demonstrated that the addition of K significantly boosted the desorption process of Mg(NH2)2/LiH by reducing the overall reaction temperature. Furthermore, Dong et al. (2014) revealed that superior results for the hydrogenation performance of the LiH-NH3 system were obtained by the addition of various potassium compounds.
In respect to this matter, it is interesting to mix niobium fluoride with potassium as a ternary compound in the form of K2NbF7 and to study its potential catalytic effect. To date, no studies have been conducted using doped K2NbF7 as a catalyst for LiAlH4. Moreover, previous studies reported that K2NbF7 enables a remarkable improvement in the hydrogen storage performance of MgH2 (Yahya et al., 2018; Yahya M. S. and Ismail M., 2018). Thus, it is of great interest to explore the influence of K2NbF7 on the desorption performances of LiAlH4. It is anticipated that the addition of K2NbF7 will have notable effects on the desorption and kinetic performances of LiAlH4.
Experimental Details
Commercial powders of LiAlH4 (purity 95%) and K2NbF7 (purity 98%) were obtained from Sigma Aldrich and were used without any modification. To minimize exposure to oxygen and water moisture, the samples were prepared and handled in the Ar-filled Mbraun Unilab glove box. In this study, 10 wt.% of K2NbF7 was mechanically milled together with LiAlH4 to explore its effect on the desorption behavior of LiAlH4. The milling process was done in a planetary ball mill (NQM-0.4) for 1 h, starting with 0.5 h of milling, followed by 6 min of rest time, and then another 0.5 h of milling in a different rotation direction at a speed of 400 rpm. The samples were placed in a hardened stainless-steel jar with four stainless balls, each 1 cm in size. The ratio of the balls to the weight of the powder was 40:1. For comparison purposes, the as-received LiAlH4 was treated under the same conditions.
The hydrogenation performances of LiAlH4 + 10 wt.% K2NbF7 were studied with temperature-programmed desorption (TPD) using Sievert-type pressure-composition-temperature (PCT) equipment (Advanced Materials Corporation). In order to determine the initial decomposition temperature, the sample was heated from room temperature to 250°C (heating rate: 5°C/min). Other than that, the desorption kinetics performances were evaluated at 90°C under 1.0 atm of pressure. The apparent activation energy, EA, was determined using differential scanning calorimetry (DSC, Mettler Toledo, DSC/TGA 1), loading 5–7 mg of the samples into a crucible and heating from 25 to 300°C at heating ramps of 15, 20, 25, and 30°C/min under an argon flow (50 ml/min). In terms of the morphology and phase structure characterizations, the samples were analyzed using scanning electron microscopy (SEM: JEOL JSM 6350LA), X-ray diffractometry (XRD, Rigaku Miniflex), and Fourier transform infrared (IR Shimadzu Tracer-100).
Results and Discussion
Figure 1 demonstrates the TPD results of the LiAlH4 and modified LiAlH4 system. The results show that the as-received and as-milled LiAlH4 have similar desorption processes that occur in two stages of desorption, as in Equations (1, 2), with 7.4 wt.% hydrogen capacity. Before the ball milling process, the first stage of desorption occurred at 147°C, with 5 wt.% of hydrogen released. Meanwhile, the desorption process for the second stage was recorded to happen at around 175°C, with a capacity of 2.4 wt.% of the hydrogen. After the milling process, the initial desorption temperature of the sample was similar to that of pure LiAlH4 but with slight temperature reductions to 144°C (first stage) and 174°C (second stage). This phenomenon showed that the 1-h milling process had an insignificant effect on the desorption behavior of LiAlH4. In contrast, the addition of 10 wt.% of K2NbF7 significantly decreased the decomposition temperature for both stages, to 90 and 149°C. However, the amount of hydrogen released from the LiAlH4 + 10 wt.% K2NbF7 sample was decreased to 6.3 wt.%. This is expected due to the dead weight of K2NbF7, which does not hold any hydrogen.
Further study on the catalytic activity of K2NbF7 was performed based on the desorption kinetics experiment. Figure 2 depicts a comparison of the hydrogen desorption at 90°C for LiAlH4 and LiAlH4 modified by the addition of 10 wt.% K2NbF7. It is noticeable that within 120 min, the undoped LiAlH4 was only able to desorb a small amount of hydrogen; 0.1 wt.% for the as-received LiAlH4 and 0.4 wt.% for the as-milled LiAlH4. Surprisingly, with the addition of 10 wt.% K2NbF7, the doped sample desorbed ~3.2 wt.% H2 within the same duration. This desorption rate was 30 times faster than that of the as-received LiAlH4. This enhancement may be correlated to the formation of surface defects and active materials through the reaction of the LiAlH4 + 10 wt.% K2NbF7 composite (Cai et al., 2016).
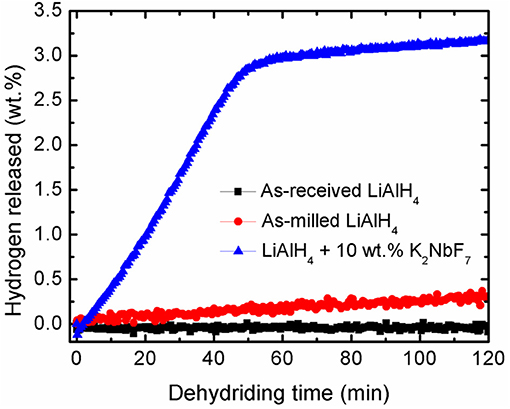
Figure 2. Dehydriding kinetics curves of as-received LiAlH4, as-milled LiAlH4, and LiAlH4 + 10 wt.% K2NbF7 at 90°C.
In terms of thermal behavior, DSC experiments were conducted for the doped and un-doped LiAlH4 samples. Figure 3 displays the DSC curves of the samples at a heating ramp of 15°C/min. Both the doped and un-doped LiAlH4 have two endothermic and exothermic peaks. The first exothermic peak corresponds to the reaction of LiAlH4 with surface hydroxyl groups, while the first endothermic peak is ascribed as its melting process. The second exothermic peak is attributed to the decomposition of LiAlH4, as described in Equation (1), and the second endothermic peak correlates with the decomposition of Li3AlH6, as described by Equation (2). Both samples exhibit similar thermal behavior, but the peaks of the LiAlH4 + 10 wt.% K2NbF7 sample occur at a lower temperature as compared to as-milled LiAlH4.
Fundamentally, the enhancement of the initial temperature to release hydrogen and the faster desorption kinetics rates are correlated with the energy barrier of LiAlH4. In this study, the decomposition activation energy (EA) is the least possible amount of energy needed by LiAlH4 to begin the hydrogen desorption process. Figure 4 shows DSC traces for several heating ramps (15, 20, 25, and 25°C/min). By referring to the plots, the activation energies for both decomposition stages of the as-milled LiAlH4 and LiAlH4 + 10 wt.% K2NbF7 samples were determined using the Kissinger analysis, as in equation (4):
where β, Tp, R, and A are the heating rate, peak temperature in the DSC curve, gas constant, and linear constant, respectively. The apparent activation energy was determined from the slope of ln [β / Tp 2] versus 1000/Tp, as shown in Figure 5.
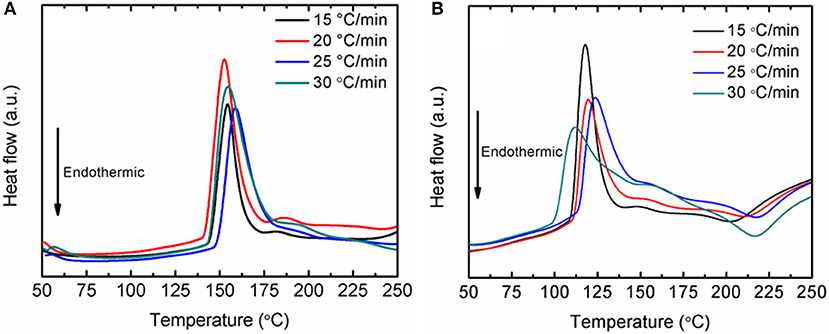
Figure 4. DSC traces at various heating ramps of the LiAlH4 when (A) as-milled and (B) with 10 wt.% of K2NbF7.
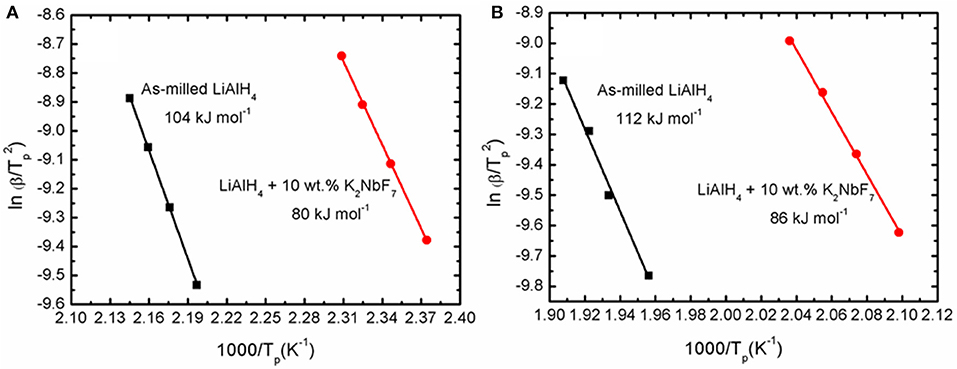
Figure 5. Corresponding Kissinger plots of the as-milled LiAlH4 and LiAlH4 + 10 wt.% K2NbF7 for the (A) first stage and (B) second stage of reaction.
The activation energy was calculated based on the second exothermic (decomposition of LiAlH4) and second endothermic (decomposition of Li3AlH6) reactions. For the as-milled LiAlH4, the activation energy values were 104 and 112 kJ/mol for the first two stages of reaction, respectively. After the addition of 10 wt.% K2NbF7, the activation energy values dropped to 80 kJ/mol (first stage) and 86 kJ/mol (second stage), 23% lower than those of the un-doped LiAlH4. These results are in good agreement with other studies that prove the addition of a catalyst is able to reduce the activation energy of LiAlH4. Table 1 lists the activation energy from previous studies for comparison purposes. The reduction in these activation energies verifies that K2NbF7 plays a major role in enhancing the desorption kinetics performance of LiAlH4.
The morphological structures of the doped and un-doped LiAlH4 were examined using SEM equipment. Figure 6 shows SEM images of the un-doped and doped-LiAlH4 samples. As shown in Figure 6, the pure LiAlH4 exhibits larger particle sizes than the milled sample. The as-received LiAlH4 (Figure 6A) has larger (15–40 μm), non-uniform rod-shaped particles. Furthermore, the as-received LiAlH4 shows a uniform size distribution and consists of “blocky” particles, consistent with the report by Varin and Zbroniec (2010). Meanwhile, after 1 hour of milling, the milled LiAlH4 (Figure 6B) displays a reduction in particle sizes but with some agglomeration and inconsistency in particle size. Then, with the addition of 10 wt.% of K2NbF7 (Figure 6C), the morphological structure of the sample was notably enhanced. The doped sample has smaller particle sizes and is less agglomerated. This observation is in line with numerous research results that have shown a reduction of particle sizes with the addition of a catalyst (Aguey-Zinsou et al., 2007; Ali et al., 2018; Yahya M. and and Ismail M., 2018). In this study, K2NbF7 functioned as a dispersing agent that impeded the sample from agglomerating. The particle size is important because smaller particles provide more area for surface defects and additional grain boundaries (Schulz et al., 1999; Sakintuna et al., 2007; Ranjbar et al., 2009). As a consequence, the desorption kinetics of LiAlH4 will be improved.
Figure 7 presents the XRD profiles of the as-received LiAlH4, as-milled LiAlH4, and LiAlH4-K2NbF7 sample. The XRD characterization was performed to explore the reaction process and the mechanism that operated during the milling process. Figure 7A displays the XRD pattern of the as-received LiAlH4 and shows that only the LiAlH4 phase was detected, which confirms the purity of the LiAlH4. The XRD pattern of the milled LiAlH4 Figure 7B shows similar peaks to the as-received LiAlH4. This result shows that LiAlH4 has high stability during the milling process and agrees well with a previous study (Ismail et al., 2010). Meanwhile, with the addition of 10 wt.% of K2NbF7 (Figure 7C), only LiAlH4 and Al peaks are visible and no peak of K2NbF7 was detected, suggesting that the amount of catalyst was too small to be picked up by the XRD. The appearance of Al peaks indicates that a part of the LiAlH4 had decomposed to Li3AlH6 and Al (reaction 1) during the milling process in the presence of 10 wt.% K2NbF7. Surprisingly, the XRD result for the 10 wt.% K2NbF7-doped LiAlH4 sample does not show any peaks of Li3AlH6. Additional characterization was carried out for a doped sample with 30 wt.% K2NbF7 (Figure 7D). A K2NbF7 peak was against not detected by the XRD for this sample. This may be because the K2NbF7 is in an amorphous state. Similar phenomena were reported by previous studies, where several catalysts like TiO2 and TiF3 were not detected by the XRD after the milling process (Ismail et al., 2011; Zang et al., 2015). However, for the LiAlH4 + 30 wt.% K2NbF7 sample, diffraction peaks corresponding to the decomposition product, Al and Li3AlH6, were detected. Meanwhile, unlike for the LiAlH4 + 10 wt.% K2NbF7 sample, for which only peaks of Al were detected while peaks of Li3AlH6 could not be discovered by XRD.
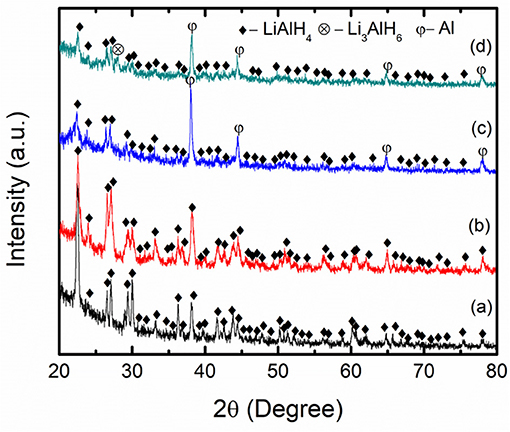
Figure 7. XRD patterns of (a) as-received LiAlH4, (b) as-milled LiAlH4, (c) LiAlH4 + 10 wt.% K2NbF7, and (d) LiAlH4 + 30 wt.% K2NbF7.
Figure 8 shows the IR spectra of the as-received LiAlH4, as-milled LiAlH4, and LiAlH4 + 10 wt.% K2NbF7 in the range of 800 to 2,000 cm−1. The FTIR characterizations were conducted to identify the presence of Li3AlH6 in the 10 wt.% K2NbF7-doped LiAlH4 sample. For all samples, two distinct regions of Al-H modes were detected at around 800–900 cm−1 ([AlH4]− stretching modes) and 1,600–1,800 cm−1 ([AlH4]− bending modes), respectively. Furthermore, with the addition of 10 wt.% K2NbF7, a weak IR absorption peak at 1,398 cm−1 was detected, which indicates the presence of Li3AlH6. This result suggests that with the addition of 10 wt.% K2NbF7, LiAlH4 was partially decomposed to Li3AlH6 and Al (reaction 1) during the milling process, consistent with the XRD results (Figure 7C).
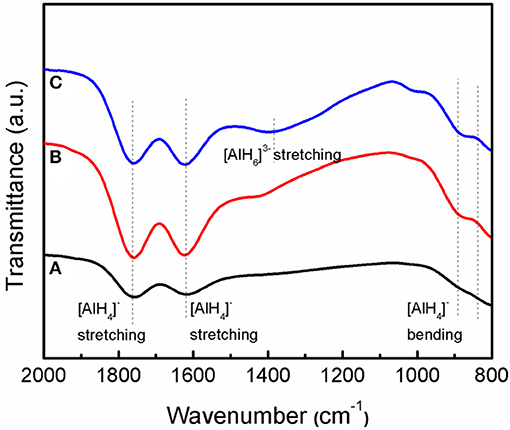
Figure 8. IR spectra of the LiAlH4 when (A) as-received, (B) as-milled, and (C) with 10 wt.% of K2NbF7.
To investigate the specific mechanism that is related to the enhanced desorption performance of LiAlH4, the dehydrogenated sample was examined using XRD. The XRD pattern for the dehydrogenated sample is depicted in Figure 9. After the dehydrogenation process at 250°C, the main peaks observed are the LiAlH4 dehydrogenation products, LiH and Al, which indicates complete dehydrogenation of LiAlH4. In addition, peaks for LiF and NbF4 were detected after the dehydrogenation process. However, the peak of the K-containing phase was not detected after the dehydrogenation process, potentially due to the low amount of catalyst.
Niobium fluoride is well established as a promising catalyst that plays a vital role in enhancing the hydrogenation performance of solid-state material (Luo et al., 2007; Malka et al., 2011; Mao et al., 2013). It is reasonable to state that the NbF4 that formed in situ after the desorption process contributes to a remarkable amelioration of the desorption behavior of LiAlH4. This result well-agreed with previous research that demonstrates the outstanding dehydrogenation performance of LiAlH4-NbF5 (Ismail et al., 2010). On the other hand, the LiF formed was believed to significantly affect the hydrogenation behavior of the doped sample based on work carried out as by Gosalawit-Utke et al. (2010). Additionally, it is believed that the formation of LiF plays a similar role in the growth of LiH and Al, since LiF has a similar cubic structure (space group: Fm-3m; Y. Liu et al., 2010). Also, LiF crystallites act as nucleation sites and facilitate the growth of LiH and Al crystallites, which promotes to the change of the nucleation morphology. These two factors significantly contribute to the kinetics enhancement achieved in the doped sample. Additionally, it is believed that K or K-containing phases also play a vital role in enhancing the desorption behavior of LiAlH4. This was deduced based on successful previous work on the application of K as a catalyst for solid-state materials (Wang et al., 2009; Dong et al., 2014). Therefore, it can be concluded that the in situ formation of LiF, NbF4, and K or K-containing phases synergistically contributed to the amelioration of the dehydrogenation kinetics of LiAlH4.
Conclusion
K2NbF7 demonstrated an excellent catalytic effect on the desorption behavior of LiAlH4. The initial temperatures at which LiAlH4 + 10 wt.% K2NbF7 released hydrogen, 90 and 149°C for the first two stages, were lower than those of the as-milled LiAlH4 (147 and 175°C). In terms of desorption kinetics behavior, the LiAlH4 + 10 wt.% K2NbF7 released 3.2 wt.% of hydrogen within 120 min, which is 30 faster than the pure LiAlH4. The addition of K2NbF7 significantly reduced the decomposition activation energy from 104 to 80 kJ/mol for the first stage and 112 to 86 kJ/mol for the second stage. The XRD spectra suggested that the in situ formation of LiF, NbF4, and K or K-containing phases acted as boosters and ameliorated the dehydrogenation behavior of LiAlH4. This work demonstrates that K2NbF7 was has a superior catalytic effect and confers better desorption behavior to LiAlH4.
Data Availability Statement
The datasets generated for this study are available on request to the corresponding author.
Author Contributions
All authors listed have made a substantial, direct and intellectual contribution to the work, and approved it for publication.
Funding
This work was financially supported by Golden Goose Research Grant (GGRG) VOT 55190, Universiti Malaysia Terengganu.
Conflict of Interest
The authors declare that the research was conducted in the absence of any commercial or financial relationships that could be construed as a potential conflict of interest.
Acknowledgments
The authors would like to acknowledge Universiti Malaysia Terengganu for providing complete facilities at which to perform this research.
References
Aguey-Zinsou, K. F., Fernandez, J. A., Klassen, T., and Bormann, R. (2007). Effect of Nb2O5 on MgH2 properties during mechanical milling. Int. J. Hydrogen Energy 32, 2400–2407. doi: 10.1016/j.ijhydene.2006.10.068
Ali, N. A., Idris, N. H., Din, M. F. M., Mustafa, N. S., Sazelee, N. A., Halim Yap, F. A., et al. (2018). Nanolayer-like-shaped MgFe2O4 synthesised via a simple hydrothermal method and its catalytic effect on the hydrogen storage properties of MgH2. RSC Adv. 8, 15667–15674. doi: 10.1039/C8RA02168F
Ali, N. A., Idris, N. H., Sazelee, N. A., Yahya, M. S., Yap, F. A. H., and Ismail, M. (2019). Catalytic effects of MgFe2O4 addition on the dehydrogenation properties of LiAlH4. Int. J. Hydrogen Energy 44, 28227–28234. doi: 10.1016/j.ijhydene.2019.09.083
Amama, P. B., Grant, J. T., Shamberger, P. J., Voevodin, A. A., and Fisher, T. S. (2012). Improved dehydrogenation properties of Ti-doped LiAlH4: role of Ti precursors. J. Phys. Chem. C 116, 21886–21894. doi: 10.1021/jp307225w
Andrei, C., Walmsley, J., Blanchard, D., Brinks, H., Holmestad, R., and Hauback, B. (2005). Electron microscopy studies of lithium aluminum hydrides. J. Alloy. Compd. 395, 307–312. doi: 10.1016/j.jallcom.2004.11.058
Ares, J., Aguey-Zinsou, K. F., Porcu, M., Sykes, J., Dornheim, M., Klassen, T., et al. (2008). Thermal and mechanically activated decomposition of LiAlH4. Mater. Res. Bull. 43, 1263–1275. doi: 10.1016/j.materresbull.2007.05.018
Balema, V., Pecharsky, V., and Dennis, K. (2000). Solid state phase transformations in LiAlH4 during high-energy ball-milling. J. Alloy. Compd. 313, 69–74. doi: 10.1016/S0925-8388(00)01201-9
Balema, V., Wiench, J., Dennis, K., Pruski, M., and Pecharsky, V. (2001). Titanium catalyzed solid-state transformations in LiAlH4 during high-energy ball-milling. J. Alloy. Compd. 329, 108–114. doi: 10.1016/S0925-8388(01)01570-5
Barthelemy, H., Weber, M., and Barbier, F. (2017). Hydrogen storage: recent improvements and industrial perspectives. Int. J. Hydrogen Energy 42, 7254–7262. doi: 10.1016/j.ijhydene.2016.03.178
Cai, J., Zang, L., Zhao, L., Liu, J., and Wang, Y. (2016). Dehydrogenation characteristics of LiAlH4 improved by in-situ formed catalysts. J. Energy Chem. 25, 868–873. doi: 10.1016/j.jechem.2016.06.004
Cao, Z., Ma, X., Wang, H., and Ouyang, L. (2018). Catalytic effect of ScCl3 on the dehydrogenation properties of LiAlH4. J. Alloys Compd. 762, 73–79. doi: 10.1016/j.jallcom.2018.05.213
Cheng, C., Chen, M., Xiao, X., Huang, X., Zheng, J., and Chen, L. (2018). Superior reversible hydrogen storage properties and mechanism of LiBH4-MgH2-Al doped with NbF5 additive. J. Phys. Chem. C 122, 7613–7620. doi: 10.1021/acs.jpcc.8b00959
Crabtree, G. W., Dresselhaus, M. S., and Buchanan, M. V. (2004). The hydrogen economy. Phys. Today 57, 39–44. doi: 10.1063/1.1878333
Dalebrook, A. F., Gan, W., Grasemann, M., Moret, S., and Laurenczy, G. (2013). Hydrogen storage: beyond conventional methods. Chem. Commun. 49, 8735–8751. doi: 10.1039/c3cc43836h
Dong, B. X., Song, L., Teng, Y. L., Ge, J., and Zhang, S. Y. (2014). Enhanced hydrogen desorption reaction kinetics by optimizing the reaction conditions and doping potassium compounds in the LiH–NH3 system. Int. J. Hydrogen Energy 39, 13838–13843. doi: 10.1016/j.ijhydene.2014.03.005
Fernandez, J. A., Aguey-Zinsou, F., Elsaesser, M., Ma, X., Dornheim, M., Klassen, T., et al. (2007). Mechanical and thermal decomposition of LiAlH4 with metal halides. Int. J. Hydrogen Energy 32, 1033–1040. doi: 10.1016/j.ijhydene.2006.07.011
Gosalawit-Utke, R., Bellosta von Colbe, J. M., Dornheim, M., Jensen, T. R., Cerenius, Y., Bonatto Minella, C., et al. (2010). LiF–MgB2 system for reversible hydrogen storage. J. Phys. Chem. C 114, 10291–10296. doi: 10.1021/jp910266m
Ismail, M., Zhao, Y., Yu, X. B., and Dou, S. X. (2010). Effects of NbF5 addition on the hydrogen storage properties of LiAlH4. Int. J. Hydrogen Energy 35, 2361–2367. doi: 10.1016/j.ijhydene.2009.12.178
Ismail, M., Zhao, Y., Yu, X. B., Nevirkovets, I. P., and Dou, S. X. (2011). Significantly improved dehydrogenation of LiAlH4 catalysed with TiO2 nanopowder. Int. J. Hydrogen Energy 36, 8327–8334. doi: 10.1016/j.ijhydene.2011.04.074
Kou, H., Sang, G., Zhou, Y., Wang, X., Huang, Z., Luo, W., et al. (2014). Enhanced hydrogen storage properties of LiBH4 modified by NbF5. Int. J. Hydrogen Energy 39, 11675–11682. doi: 10.1016/j.ijhydene.2014.05.179
Langmi, H. W., McGrady, G. S., Liu, X., and Jensen, C. M. (2010). Modification of the H2 desorption properties of LiAlH4 through doping with Ti. J. Phys. Chem. C 114, 10666–10669. doi: 10.1021/jp102641p
Li, L., An, C., Wang, Y., Xu, Y., Qiu, F., Wang, Y., et al. (2014). Enhancement of the H2 desorption properties of LiAlH4 doping with NiCo2O4 nanorods. Int. J. Hydrogen Energy 39, 4414–4420. doi: 10.1016/j.ijhydene.2013.12.210
Li, L., Qiu, F., Wang, Y., Xu, Y., An, C., Liu, G., et al. (2013). Enhanced hydrogen storage properties of TiN–LiAlH4 composite. Int. J. Hydrogen Energy 38, 3695–3701. doi: 10.1016/j.ijhydene.2013.01.088
Li, L., Wang, Y., Jiao, L., and Yuan, H. (2015). Enhanced catalytic effects of Co@C additive on dehydrogenation properties of LiAlH4. J. Alloys Compd. 645(Suppl.1), S468–S471. doi: 10.1016/j.jallcom.2014.12.080
Li, Z., Li, P., Wan, Q., Zhai, F., Liu, Z., Zhao, K., et al. (2013). Dehydrogenation improvement of LiAlH4 catalyzed by Fe2O3 and Co2O3 nanoparticles. J. Phys. Chem. C 117, 18343–18352. doi: 10.1021/jp405844z
Li, Z., Liu, S., Si, X., Zhang, J., Jiao, C., Wang, S., et al. (2012). Significantly improved dehydrogenation of LiAlH4 destabilized by K2TiF6. Int. J. Hydrogen Energy 37, 3261–3267. doi: 10.1016/j.ijhydene.2011.10.038
Liu, S., Ma, Q., Lü, H., Zheng, X., Feng, X., Xiao, G., et al. (2014). Study on hydrogen release capacity of LiAlH4 doped with CeO2. Rare Metal. Mat. Eng. 43, 544–547. doi: 10.1016/S1875-5372(14)60073-4
Liu, S. S., Sun, L. X., Zhang, Y., Xu, F., Zhang, J., Chu, H. L., et al. (2009). Effect of ball milling time on the hydrogen storage properties of TiF3-doped LiAlH4. Int. J. Hydrogen Energy 34, 8079–8085. doi: 10.1016/j.ijhydene.2009.07.090
Liu, X., Beattie, S. D., Langmi, H. W., McGrady, G. S., and Jensen, C. M. (2012). Ti-doped LiAlH4 for hydrogen storage: rehydrogenation process, reaction conditions and microstructure evolution during cycling. Int. J. Hydrogen Energy 37, 10215–10221. doi: 10.1016/j.ijhydene.2012.04.018
Liu, Y., Wang, F., Cao, Y., Gao, M., Pan, H., and Wang, Q. (2010). Mechanisms for the enhanced hydrogen desorption performance of the TiF4-catalyzed Na2LiAlH6 used for hydrogen storage. Energy Environ. Sci. 3, 645–653. doi: 10.1039/b920270f
Luo, Y., Wang, P., Ma, L. P., and Cheng, H. M. (2007). Enhanced hydrogen storage properties of MgH2 co-catalyzed with NbF5 and single-walled carbon nanotubes. Scripta Mater. 56, 765–768. doi: 10.1016/j.scriptamat.2007.01.016
Luo, Y., Wang, P., Ma, L. P., and Cheng, H. M. (2008). Hydrogen sorption kinetics of MgH2 catalyzed with NbF5. J. Alloys Compd. 453, 138–142. doi: 10.1016/j.jallcom.2006.11.113
Malka, I., Pisarek, M., Czujko, T., and Bystrzycki, J. (2011). A study of the ZrF4, NbF5, TaF5, and TiCl3 influences on the MgH2 sorption properties. Int. J. Hydrogen Energy 36, 12909–12917. doi: 10.1016/j.ijhydene.2011.07.020
Mao, J., Guo, Z., Yu, X., and Liu, H. (2013). Combined effects of hydrogen back-pressure and NbF5 addition on the dehydrogenation and rehydrogenation kinetics of the LiBH4-MgH2 composite system. Int. J. Hydrogen Energy 38, 3650–3660. doi: 10.1016/j.ijhydene.2012.12.106
Parra, D., Valverde, L., Pino, F. J., and Patel, M. K. (2019). A review on the role, cost and value of hydrogen energy systems for deep decarbonisation. Renew. Sust. Energ Rev. 101, 279–294. doi: 10.1016/j.rser.2018.11.010
Pukazhselvan, D., Kumar, V., and Singh, S. (2012). High capacity hydrogen storage: basic aspects, new developments and milestones. Nano Energy 1, 566–589. doi: 10.1016/j.nanoen.2012.05.004
Ranjbar, A., Guo, Z., Yu, X., Wexler, D., Calka, A., Kim, C., et al. (2009). Hydrogen storage properties of MgH2-SiC composites. Mater. Chem. Phys. 114, 168–172. doi: 10.1016/j.matchemphys.2008.09.001
Resan, M., Hampton, M. D., Lomness, J. K., and Slattery, D. K. (2005). Effects of various catalysts on hydrogen release and uptake characteristics of LiAlH4. Int. J. Hydrogen Energy 30, 1413–1416. doi: 10.1016/j.ijhydene.2004.12.009
Sakintuna, B., Lamari-Darkrim, F., and Hirscher, M. (2007). Metal hydride materials for solid hydrogen storage: a review. Int J Hydrogen Energy 32, 1121–1140. doi: 10.1016/j.ijhydene.2006.11.022
Sazelee, N., Yahya, M., Idris, N., Din, M. M., and Ismail, M. (2019). Desorption properties of LiAlH4 doped with LaFeO3 catalyst. Int. J. Hydrogen Energy 44, 11953–11960. doi: 10.1016/j.ijhydene.2019.03.102
Schulz, R., Huot, J., Liang, G., Boily, S., Lalande, G., Denis, M., et al. (1999). Recent developments in the applications of nanocrystalline materials to hydrogen technologies. Mater. Sci. Eng. A 267, 240–245. doi: 10.1016/S0921-5093(99)00098-2
Sulaiman, N., and Ismail, M. (2017). Catalytic effect of SrFe12O19 on the hydrogen storage properties of LiAlH4. Int. J. Hydrogen Energy 42, 19126–19134. doi: 10.1016/j.ijhydene.2017.06.005
Sulaiman, N., Mustafa, N., and Ismail, M. (2016). Effect of Na3FeF6 catalyst on the hydrogen storage properties of MgH2. Dalton Trans. 45, 7085–7093. doi: 10.1039/C6DT00068A
Sun, T., Huang, C., Wang, H., Sun, L., and Zhu, M. (2008). The effect of doping NiCl2 on the dehydrogenation properties of LiAlH4. Int. J. Hydrogen Energy 33, 6216–6221. doi: 10.1016/j.ijhydene.2008.08.027
Suttisawat, Y., Rangsunvigit, P., Kitiyanan, B., Muangsin, N., and Kulprathipanja, S. (2007). Catalytic effect of Zr and Hf on hydrogen desorption/absorption of NaAlH4 and LiAlH4. Int J Hydrogen Energy 32, 1277–1285. doi: 10.1016/j.ijhydene.2006.07.020
Varin, R., and Zbroniec, L. (2010). Decomposition behavior of unmilled and ball milled lithium alanate (LiAlH4) including long-term storage and moisture effects. J. Alloy. Compd. 504, 89–101. doi: 10.1016/j.jallcom.2010.05.059
Varin, R. A., and Parviz, R. (2012). The effects of the micrometric and nanometric iron (Fe) additives on the mechanical and thermal dehydrogenation of lithium alanate (LiAlH4), its self-discharge at low temperatures and rehydrogenation. Int. J. Hydrogen Energy 37, 9088–9102. doi: 10.1016/j.ijhydene.2012.02.182
Wang, J., Liu, T., Wu, G., Li, W., Liu, Y., Araújo, C. M., et al. (2009). Potassium-modified Mg(NH2)2/2 LiH system for hydrogen storage. Angew Chem. Int. Ed. 48, 5828–5832. doi: 10.1002/anie.200805264
Wang, X., Xiao, X., Zheng, J., Huang, X., Chen, M., and Chen, L. (2020). In-situ synthesis of amorphous Mg(BH4)2 and chloride composite modified by NbF5 for superior reversible hydrogen storage properties. Int. J. Hydrogen Energy 45, 2044–2053. doi: 10.1016/j.ijhydene.2019.11.023
Winter, C. J. (2009). Hydrogen energy—abundant, efficient, clean: a debate over the energy-system-of-change. Int. J. Hydrogen Energy 34, S1–52. doi: 10.1016/j.ijhydene.2009.05.063
Wohlwend, J. L., Amama, P. B., Shamberger, P. J., Varshney, V., Roy, A. K., and Fisher, T. S. (2012). Effects of Titanium-containing additives on the dehydrogenation properties of LiAlH4: a computational and experimental study. J. Phys. Chem. C 116, 22327–22335. doi: 10.1021/jp3050109
Xia, Y., Zhang, H., Sun, Y., Sun, L., Xu, F., Sun, S., et al. (2019). Dehybridization effect in improved dehydrogenation of LiAlH4 by doping with two-dimensional Ti3C2. Mater. Today Nano. 8:100054. doi: 10.1016/j.mtnano.2019.100054
Xiao, X., Shao, J., Chen, L., Kou, H., Fan, X., Deng, S., et al. (2012). Effects of NbF5 addition on the de/rehydrogenation properties of 2LiBH4/MgH2 hydrogen storage system. Int. J. Hydrogen Energy 37, 13147–13154. doi: 10.1016/j.ijhydene.2012.03.140
Xueping, Z., Ping, L., Humail, I., Fuqiang, A., Guoqing, W., and Xuanhui, Q. (2007). Effect of catalyst LaCl3 on hydrogen storage properties of lithium alanate (LiAlH4). Int. J. Hydrogen Energy 32, 4957–4960. doi: 10.1016/j.ijhydene.2007.06.031
Xueping, Z., Ping, L., and Xuanhui, Q. (2009). Effect of additives on the reversibility of lithium alanate (LiAlH4). Rare. Metal Mat. Eng. 38, 766–769. doi: 10.1016/S1875-5372(10)60034-3
Yahya, M., and Ismail, M. (2018). Synergistic catalytic effect of SrTiO3 and Ni on the hydrogen storage properties of MgH2. Int. J. Hydrogen Energy 43, 6244–6255. doi: 10.1016/j.ijhydene.2018.02.028
Yahya, M. S., and Ismail, M. (2018). Improvement of hydrogen storage properties of MgH2 catalyzed by K2NbF7 and multiwall carbon nanotube. J. Phys. Chem. C 122, 11222–11233. doi: 10.1021/acs.jpcc.8b02162
Yahya, M. S., Sulaiman, N. N., Mustafa, N. S., Halim Yap, F. A., and Ismail, M. (2018). Improvement of hydrogen storage properties in MgH2 catalysed by K2NbF7. Int. J. Hydrogen Energy 43, 14532–14540. doi: 10.1016/j.ijhydene.2018.05.157
Yap, F. H., Yahya, M., and Ismail, M. (2017). Enhancement of hydrogen storage properties in 4MgH2 -Na3AlH6 composite catalyzed by TiF3. Int. J. Hydrogen Energy 42, 21096–21104. doi: 10.1016/j.ijhydene.2017.07.012
Youn, J. S., Phan, D. T., Park, C. M., and Jeon, K. J. (2017). Enhancement of hydrogen sorption properties of MgH2 with a MgF2 catalyst. Int. J. Hydrogen Energy 42, 20120–20124. doi: 10.1016/j.ijhydene.2017.06.130
Zang, L., Cai, J., Zhao, L., Gao, W., Liu, J., and Wang, Y. (2015). Improved hydrogen storage properties of LiAlH4 by mechanical milling with TiF3. J. Alloys Compd. 647, 756–762. doi: 10.1016/j.jallcom.2015.06.036
Zhai, F., Li, P., Sun, A., Wu, S., Wan, Q., Zhang, W., et al. (2012). Significantly improved dehydrogenation of LiAlH4 destabilized by MnFe2O4 nanoparticles. J. Phys. Chem. C 116, 11939–11945. doi: 10.1021/jp302721w
Keywords: hydrogen storage, lithium aluminum hydride, desorption, catalyst, metal halide
Citation: Ali NA, Sazelee N, Yahya MS and Ismail M (2020) Influence of K2NbF7 Catalyst on the Desorption Behavior of LiAlH4. Front. Chem. 8:457. doi: 10.3389/fchem.2020.00457
Received: 16 February 2020; Accepted: 01 May 2020;
Published: 12 June 2020.
Edited by:
Guanglin Xia, Fudan University, ChinaReviewed by:
Xuezhang Xiao, Zhejiang University, ChinaChu Liang, Zhejiang University of Technology, China
Copyright © 2020 Ali, Sazelee, Yahya and Ismail. This is an open-access article distributed under the terms of the Creative Commons Attribution License (CC BY). The use, distribution or reproduction in other forums is permitted, provided the original author(s) and the copyright owner(s) are credited and that the original publication in this journal is cited, in accordance with accepted academic practice. No use, distribution or reproduction is permitted which does not comply with these terms.
*Correspondence: Mohammad Ismail, bW9oYW1tYWRpc21haWwmI3gwMDA0MDt1bXQuZWR1Lm15