- 1Key Laboratory of Molecular and Nano Probes, Ministry of Education, College of Chemistry, Chemical Engineering and Materials Science, Collaborative Innovation Center of Functionalized Probes for Chemical Imaging in Universities of Shandong, Institute of Molecular and Nano Science, Shandong Normal University, Jinan, China
- 2College of Chemical Engineering and Safety, Binzhou University, Binzhou, China
The ever-growing requirement of ammonia for industry and energy supply motivates people to find clean and cost-effective alternatives to overcome the shortcomings of the century-old Haber-Bosch process. Electrocatalytic N2 reduction (NRR) is considered a prospective way for ammonia production at ambient conditions. Recently, two-dimensional (2D) metal-free materials are emerging as highly efficient and robust electrocatalysts for NRR owing to their advanced features: highly exposed surface, abundant active sites, tunable electronic states, and long-term stability compared to metal-based and bulk catalysts. In this minireview, we briefly summarize the latest edge reports on 2D metal-free materials catalyzed NRR. Also, we discuss the challenges and perspectives on the design and synthesis of novel 2D metal-free catalysts.
Introduction
As a fundamental chemical with fast-growing industrial demand due to increasing population and economy, ammonia (NH3) is not only an essential source of manmade fertilizer, but also a promising candidate of carbon-free carrier of hydrogen energy (Schloegl, 2003; Service, 2014). The most important way for producing ammonia is the fixation (reduction) of atmospherically abundant dinitrogen gas (N2). Besides the natural biological N2 fixation by nitrogenase enzymes, to date, industrial-scale NH3 production is still relying on traditional Haber-Bosch process with iron based catalysts at high temperatures (350–600°C) and pressures (150–350 atm), which annually requires about 1.4% of global energy consumption and a considerable CO2 emission (Kandemir et al., 2013; van der Ham et al., 2014). Therefore, searching for alternative ways for inexpensive, clean, and sustainable ammonia production is imperative. In the past years, studies on biomimetic catalysis, photocatalysis, and photo(electro)catalysis for N2 fixation under mild conditions have emerged (Li et al., 2015; Wang et al., 2017). Among them, electrocatalytic nitrogen reduction reaction (NRR) is considered a promising process for its cost-effective and environ-friendly advantages (Seh et al., 2017; Lv et al., 2018b; Hao et al., 2019).
Generally, for a heterogeneous electrocatalyst, there are two possible mechanisms for NRR: associative and dissociative (Suryanto et al., 2019). The dissociative mechanism is relatively hard to proceed because it requires a high energy barrier to break the N≡N triple bond. In associative mechanism, hydrogenation of nitrogen may occur through distal or alternating pathways (Nazemi et al., 2018). Both of the two pathways need the participation of protons. However, on most heterogeneous catalyst surfaces, protons and electrons in aqueous solution prefer to undergo hydrogen evolution reaction (HER) rather than NRR, leading to low selectivity and unsatisfactory Faradaic efficiencies (FEs) (Singh et al., 2017). So far, a majority of reported NRR catalysts are based on noble/transition/p-block metals (Li et al., 2019; Wang et al., 2019). The scarcity of noble metals impedes their widespread application (Bao et al., 2017). For transition metals, the NH3 yield and FE are severely limited by the poor contact of N2 and catalyst surface as well as high HER competitiveness due to the easy formation of metal-H bonds (Su et al., 2012; Zheng et al., 2015; Guo et al., 2018).
In the past decade, metal-free electrocatalysts have attracted substantial attention in the field of energy conversion (Liu and Dai, 2016; Feng et al., 2017; Xie et al., 2018; Qin et al., 2019). Notably, metal-free catalysts also have huge potential for catalyzing NRR owing to their beneficial properties including good conductivity, controllable porosity, and intrinsic activity for N2 reduction (Zhao et al., 2019). Some metal-free catalysts can deliver favorable NRR selectivity due to the synergistic interactions derived by adjustable heteroatom doping. Moreover, metal-free catalyst could have good durability by avoiding the inherent corrosion susceptibility of metal-based catalysts in acidic/basic media (Chu et al., 2019). In the family of metal-free catalysts, two-dimensional (2D) materials (graphene, g-C3N4, boron sheet, black phosphorus, etc.) have been a research hotspot for the unique features absent from their bulk counterparts: high lateral-area-to-thickness ratio, tunable electronic states, and rich surface defects with active bonding sites. The above advances of 2D metal-free materials are greatly beneficial for constructing electrocatalytically active catalysts. In this minireview, we briefly summarize the recent progresses and challenges in carbon/boron/phosphorus based 2D metal-free electrocatalysts for NRR.
Carbon-Based Catalysts
Although graphene has been widely explored with its intrinsic advantages such as high conductivity, pristine graphene, or chemically reduced graphene oxide (RGO), it is considered to have less catalytic activity. Doping with nonmetallic heteroatoms is an encouraging way for enhancing the catalytic performance of graphene. In recent years, there have been reports of nitrogen doped porous carbons/carbon nanospikes/boron-rich covalent organic frameworks (COFs) with superior catalytic activities for NRR (Liu et al., 2018b, 2019; Mukherjee et al., 2018; Song et al., 2018). The enhanced catalytic performance of doped carbon may be ascribed to the strong electronegativity of nitrogen dopants which positively charges the adjacent carbon atoms (Hu and Dai, 2017).
Unlike nitrogen, boron is less electronegative than carbon (2.04 vs. 2.55) (Yang et al., 2011). When forming a B-C bond, the doped B atom is positively charged with an empty orbital which can bind with the lone pair electrons in N2. Moreover, B can act as a Lewis acid site, which is beneficial to the adsorption of weak Lewis base N2 and repulsion of Lewis acid H+ in acidic media. Yu et al. introduced B into graphene framework by heating H3BO3 and graphene oxide in H2-Ar mixed atmosphere (Yu et al., 2018) (Figure 1A). The B doping did not change the typical planar structure of graphene, confirmed by transmission electron microscopy (TEM) images. X-ray photoelectron spectroscopy (XPS) showed the electron redistribution in graphene sheet by different B doping structures at the edge or defect sites. Temperature-programmed desorption (TPD) revealed that B atom could afford chemical adsorption sites for N2. Density functional theory (DFT) calculations indicated that in the several types of boron-doped carbon structures, B atom in BC3 is more positively charged. And BC3 structure provided the lowest energy barrier for N2 fixation reaction. The resulted catalyst presents a superior NH3 evolution rate of 9.8 μg h−1 cm−2 and excellent FE of 10.8% at −0.5 V vs. RHE, five and 10 times of those of undoped graphene, respectively.
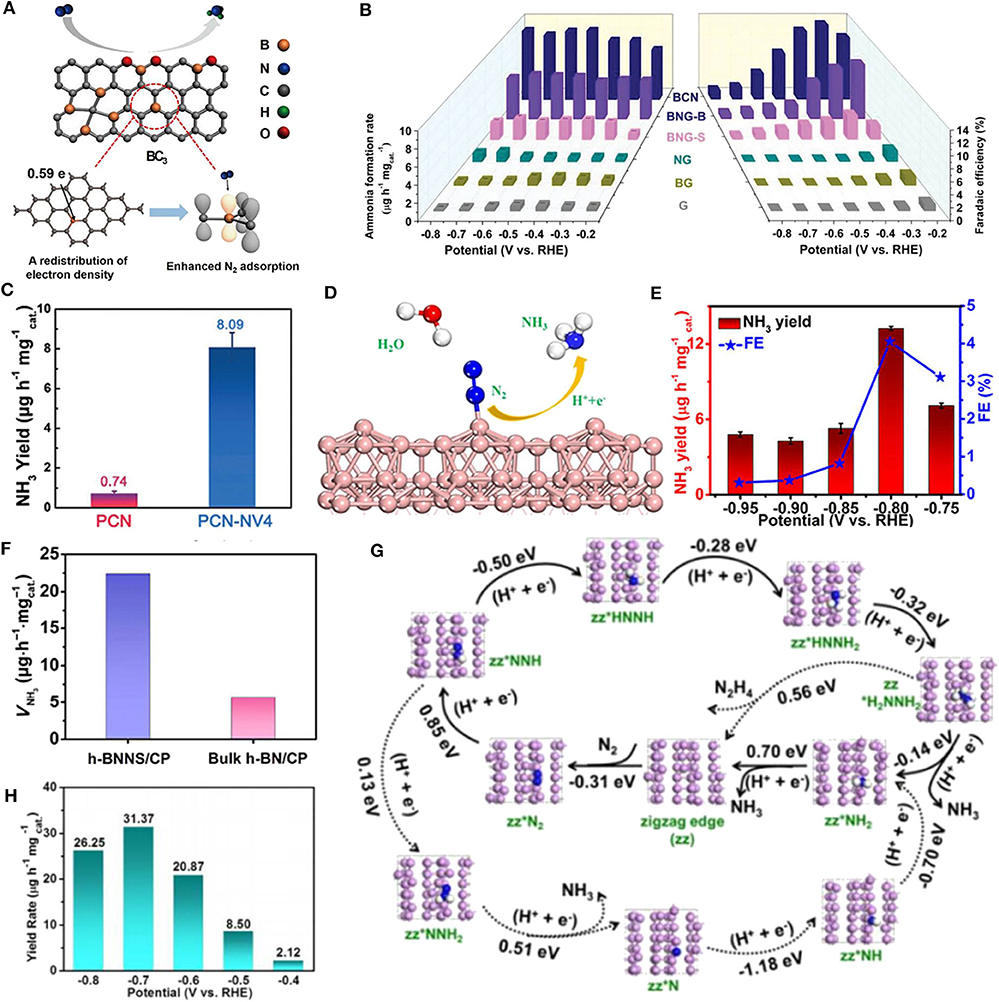
Figure 1. (A) Schematic illustration of boron doped graphene catalyzed NRR. BC3 structure provided the lowest energy barrier for N2 fixation reaction. Reproduced with permission from Yu et al. (2018). Copyright 2018 Cell. (B) NH3 yields and Faradaic efficiencies of relative catalysts. Reproduced with permission from Chen et al. (2019a). Copyright 2019 Wiley-VCH Verlag GmbH & Co. KGaA. (C) NH3 yields of carbon nitride with and without nitrogen vacancies at −0.2 V vs. RHE. Reproduced with permission from Lv et al. (2018a). Copyright 2018 Wiley-VCH Verlag GmbH & Co. KGaA. (D) Schematic illustration of boron nanosheet (BNS) catalyzed NRR. Compared to bare BNS, the B atoms of both oxidized and H-deactivated BNS have better activity for catalyzing NRR. (E) NH3 production rates and Faradaic efficiencies for BNS/CP at various potentials. Both (D,E) are reproduced with permission from Zhang et al. (2019b). Copyright 2019 American Chemical Society. (F) NH3 yields of h-BNNS/CP and bulk h-BN/CP at −0.75 V vs. RHE after 2 h electrolysis. Reproduced with permission from Zhang et al. (2019c). Copyright 2019 Springer. (G) Supposed NRR mechanisms at the zigzag edge of FL-BP NSs (solid line: low energy route, dotted line: unfavorable route). (H) NH3 yields of FL-BP NSs/CF at corresponding potentials. Both (G,H) are reproduced with permission from Zhang et al. (2019a). Copyright 2019 Wiley-VCH Verlag GmbH & Co. KGaA.
It's worth noting that multiple dopants may work synergistically to improve catalytic performance. For instance, a metal-free electrocatalyst of boron/nitrogen co-doped carbon (BCN) is reported (Chen et al., 2019a) (Figure 1B). At first, B, N co-doped graphene was synthesized by annealing graphene oxide and boric acid in NH3 gas (BNG-B) or in Ar gas (BNG-S). In BNG-B, B-N pairs were formed through the reaction of boric acid and ammonia. The authors further prepared defect enriched BCN by pyrolysis of boric acid, urea, and PEG-2000 in Argon gas. DFT calculations and electrochemistry performance revealed that the co-doping of N and B was favorable for NRR and inactive for HER, which significantly promoted FE. In particular, the B-N pairs could act as active triggers for nitrogen reduction, and the adjacent edge carbon atoms provided the reactive sites. Thus, the doping of B-N pairs plays a key role in activating NRR as well as inhibiting the unfavorable HER process. As a result, the BCN catalyst delivered an excellent NH3 formation rate (7.75 μg h−1 mg−1cat.) and Faradaic efficiency (13.79%) at −0.3 V vs. RHE, even outperforming most of the metal-based electrocatalysts.
Recently, Asiri, Sun, and their coworkers constructed oxygen doped porous carbon nanosheet (O-CNS) via a simple annealing process of sodium citrate in Ar (Chen et al., 2019b). The as-prepared catalyst delivered sheet-like structure confirmed by TEM images. The energy dispersive X-ray (EDX) elemental mapping and XPS suggested that O has been uniformly doped into carbon nanosheet. To evaluate the catalytic activity, NRR analysis was tested in 0.1 M HCl solution with O-CNS loading amount of 0.1 mg cm−2 on carbon paper. The as-obtained catalyst reached a high NH3 production of 18.03 μg h−1 mg−1cat. at −0.55 V and promising FE of 10.3% at −0.45 V with good durability. The same group also incorporated sulfur into graphene to form an efficient and stable electrocatalyst (S-G) for NRR (Xia et al., 2019). S-G catalyst was synthesized through a heat treatment process of graphene oxide with benzyl disulfide. TEM images showed that compared to undoped graphene, S-G presented the same nanosheet morphology. Raman spectra suggested that S-G was more disordered in structure than pristine graphene. DFT calculation demonstrated that by the substitution of sulfur, the neighboring carbon atoms could perform as active sites for N2 fixation. Consequently, this catalyst supported on carbon paper delivered outstanding electrochemical activity and stability (NH3 yield of 27.3 μg h−1 mg−1cat. at −0.6 V, FE of 11.5% at −0.5 V).
Graphitic carbon nitride (g-C3N4), as a metal-free catalyst with 2D layered structure, has been substantially studied for its promising application in photo/electrocatalytic reactions of energy conversion (Wang et al., 2012; Cao et al., 2015). It has been considered that vacancies on 2D catalyst materials may play the crucial role of providing active sites for surface reactions (Xie et al., 2020). Lv and Qian et al. proposed polymeric carbon nitride (PCN) with nitrogen vacancy (NV) defects (Lv et al., 2018a) (Figure 1C). The introduction of NVs can be easily controlled by a re-calcination process in Ar atmosphere. XRD and Fourier-transform infrared (FTIR) spectroscopy showed that the NVs destroyed long-range periodicity in PCN while inherited the short-range structure such as s-triazine ring and aromatic C-N heterocycles. Electron paramagnetic resonance (EPR) spectra further illustrated that the NVs could change the electron distribution on the nearby carbon atoms. Theoretical calculations reveal that N2 molecule could form a dinuclear end-on bound structure with NVs when adsorbing on PCN sheet, during which the N≡N bond of adsorbed N2 is strongly activated and stretches longer. In NRR test, the PCN with NVs defect engineering presented surprising electrocatalytic performance. Compared to bare PCN, PCN with NVs promoted NH3 yield for over 10-fold (8.09 μg h−1 mg−1cat.), and a superior Faradaic efficiency of 11.59% was achieved at −0.2 V vs. RHE.
Very recently, Chu et al. incorporated sulfur dopants to occupy NVs of g-C3N4 by annealing NV-C3N4 in sulfur vapor (Chu et al., 2020). The as-prepared S-NV-C3N4 showed wrinkled sheet-like structure in TEM images, similar to NV-C3N4 precursor. XPS analysis proposed that rather than with nitrogen atoms, the doped sulfur preferred to interact with carbon atoms. In contrast to S-NV-C3N4, the doping of S significantly enhanced the NRR performance: a NH3 yield of 32.7 μg h−1 mg−1cat. and an FE of 14.1% at −0.4 V vs. RHE in 0.5 M LiClO4 as well as good durability for at least 20 h. In order to examine the underlying mechanism of activity promotion by S doping, DFT calculations were performed. It shows that S dopant played the role of stabilizing *N2H and destabilizing *NH2 to break the scaling relation of them. Moreover, the high Gibbs free energy for water dissociation and low free energy for H* adsorption of S-NV-C3N4 suppressed undesired HER process, leading to a high Faradaic efficiency for N2-to-NH3 conversion.
Boron-Based Catalysts
Boron carbide (B4C) has attracted much research attention as electrodes and catalyst backbones due to its good electronic conductivity and chemical stability (Mu et al., 2016; Song et al., 2017). In 2018, Qiu et al. demonstrated that B4C nanosheet could act as a promising metal-free electrocatalyst for N2 fixation both in acidic and neutral media (Qiu et al., 2018). The B4C nanosheet was simply prepared by ultrasonic exfoliating of commercial bulk B4C in ethanol. Few-layered B4C nanosheets can be seen from TEM images of samples after exfoliation. The catalytic mechanism is explored by DFT calculation; the rate-limiting step is considered as *NH2-*NH2→*NH2-*NH3 reaction. NRR test is conducted to evaluate the electrochemical performance of as-prepared catalyst. In 0.1 M HCl, the B4C nanosheet exhibits superior NH3 yield of 26.57 μg h−1 mg−1cat. with a high Faradaic efficiency of 15.95% at −0.75 V. Moreover, this catalyst also behaves impressively in neutral media. In 0.1 M Na2SO4, the NH3 yield reaches 14.70 μg h−1 mg−1cat., and FE achieves 9.24%.
Boron has been considered to have unique bonding features due to its electron deficiency nature (Légaré et al., 2018; Liu et al., 2018a). It's noteworthy that a 2D boron sheet is reported to possess metallic character with high conductivity and good stability (Xu et al., 2015). Recently, Zhang and Wu et al. proposed boron nanosheet (BNS) as an elemental 2D electrocatalyst for efficient N2-to-NH3 reduction (Zhang et al., 2019b) (Figure 1D). The BNS was obtained by ultrasonic exfoliation of commercial bulk B powders in isopropyl alcohol (IPA) and supported on carbon paper (CP). Atomic force microscopy (AFM) images and height profile reveal the average thickness of BNS to be ~3.0 nm. N2 adsorption–desorption isotherms showed that BNS has remarkably enhanced surface area than that of bulk boron. In 0.1 M Na2SO4 solution, BNS/CP achieves inspiring NH3 yield of 13.22 μg h−1 mg−1cat. and Faradaic efficiency of 4.04% at −0.80 V vs. RHE as well as good durability (Figure 1E). The excellent electrocatalytic performance of BNS may come from the abundant exposing active sites in its 2D structure and better conductivity for electron transfer (confirmed by electrochemical impedance spectroscopy analysis) than bulk B/CP. Additionally, DFT calculations demonstrated that compared to clean BNS, the B atoms of both oxidized and H-deactivated BNS have better activity for catalyzing NRR, and the desorption of the second NH3 molecule determines the reaction rate.
In another independent report, Fan et al. proposed a liquid ultrasonic exfoliation method to efficiently synthesize single- and few-layer boron nanosheets from bulk boron (Fan et al., 2019). They found six organic solvents from 21 candidates suitable for exfoliation and dispersion boron, in which benzyl benzoate performs best. XPS showed that in boron nanosheets, the B-O peak was reduced compared to that in bulk boron, implying the dislodgment of oxygen-containing groups by exfoliation. Crystalline features analysis confirmed the β-rhombohedral B structure in as-obtained boron nanosheets. The average layer number of boron flakes was estimated to be about 13 layers, which corresponds to ca. 7.5 nm in thickness, denoted by AFM. In NRR test in 0.1 M HCl, the exfoliated boron nanosheets present a NH3 yield of 3.12 μg h−1 mg−1cat. and an FE of 4.84 % at −0.14 V, about a 2-fold enhancement to those of bulk boron. Theoretical calculations demonstrated that the icosahedron borons in 2D nanosheets could act as reactive sites and strongly adsorb N2 which benefits to the initial activation.
Although 2D hexagonal boron nitride (h-BN) has a wide band gap, ultrathin h-BN layers can narrow the band gap by introducing defects and improve conductivity by electron tunneling, thus deliver potential capability for catalyzing NRR. Zhang and Du et al. found that h-BN nanosheet (h-BNNS) exfoliated from bulk h-BN powders in ethanol could act as an efficient electrocatalyst for N2-to-NH3 reduction (Zhang et al., 2019c). AFM images showed that the average thickness of h-BNNS is about 1.3 nm. In NRR experiment, h-BNNS was supported on carbon paper with a loading amount of 0.1 mg cm−2, and tested in 0.1 M HCl solution. The catalyst achieved an encouraging NH3 yield of 22.4 μg h−1 mg−1cat. and a Faradic efficiency of 4.7% at −0.75 V vs. RHE with good stability in 24 h electrolysis (Figure 1F). DFT calculations further reveal that the energy barrier for NRR can be remarkably reduced through activating inert N2 molecule by the unsaturated boron at the edge site.
Phosphorus-Based Catalysts
Phosphorus is an earth-abundant element widely used as dopants in catalysts. Briefly, elemental P exists in the following allotropes: white phosphorus (WP), red phosphorus (RP), violet phosphorus (VP), and black phosphorus (BP). Among them, few-layered black phosphorus (BP) has attracted numerous research interests as a star candidate in the family of 2D structured materials. BP has been applied in field-effect transistors, gas sensors, catalysis, and energy storage due to its high hole mobility, tunable band gaps, and unique chemical features (Pang et al., 2018; Wu et al., 2018; Xing et al., 2019; Shen et al., 2020). Notably, BP has a valence electron structure (3s2 3p3) similar to that of nitrogen (2s2 2p3). Hence, holding the concept of “like dissolves like.” Zhang et al. exfoliated orthorhombic black phosphorus into few-layer nanosheets (FL-BP NSs), which are used to catalyze NRR (Zhang et al., 2019a). AFM analysis showed that FL-BP NSs have a thickness of ~4.1 nm, corresponding to five to seven layers. The NRR activity test was performed in 0.01 M HCl. The FL-BP NSs exhibit dramatically high NH3 yield of 31.37 μg h−1 mg−1cat. at −0.7 V with FE of 5.07% at −0.6 V (Figure 1H). Moreover, the active sites for N2 activation are suggested to be the zigzag and diff-zigzag edges of the FL-BP NSs by DFT calculations (Figure 1G).
Conclusion
In this minireview, the latest approaches in two-dimensional (2D) metal-free electrocatalysts for NRR are briefly summarized and discussed. Aiming to replace the Haber-Bosch process, research on electrochemical NRR has been one of the hottest points in catalysis and lots of inspiring works have been reported. However, the existing catalysts are still at a considerable distance from satisfactory NH3 yield and Faradaic efficiency for industrial use. Given the complicated reaction pathways of NRR accompanied by the competition of HER, the real active sites for N2 activation remains unclear and the exact catalytic mechanism need to be better understood. More profound insights on structure–function relationship should be obtained by further combination of experimental research and theoretical calculation. For the goal of improving NRR as well as suppressing HER, several strategies should be adopted. First, the elemental composition of current metal-free catalysts is still limited (B, C, N, P, S). The element choice for catalysts and dopants needs to be enlarged. Second, considering the advantages of 2D architecture, methods including defect engineering, doping control, adjusting surface states, and tailoring electronic structures should be carried out to optimize the catalytic performance. Third, to accurately evaluate the catalytic performance, quantitative detection of produced ammonia is of vital importance for data reliability. However, it's hard for traditional detection methods (indophenol blue, Nessler's reagent, etc.) to avoid N species contamination during the test, especially for N containing catalysts. Thus, the involvement of confirmation experiment using 15N isotope tracer is quite essential for the test proposal. At last, we anticipate that on the basis of developing highly active electrocatalysts, more attention should be put into the issues on the scalable and reproducible preparation of catalyst and fast fabrication of electrodes for the practical application of electrochemical N2 fixation in the future.
Author Contributions
BT supervised the project. XX and BT mainly wrote the paper. BL and SL co-wrote the paper. All authors discussed the results and commented on the manuscript and agree to be accountable for the content of the work.
Conflict of Interest
The authors declare that the research was conducted in the absence of any commercial or financial relationships that could be construed as a potential conflict of interest.
Acknowledgments
We thank the National Natural Science Foundation of China (21535004, 91753111, 21927811, 21390411, 21301110) and the Natural Science Foundation of Shandong Province of China (ZR2013BQ011) for supporting this work.
References
1. Bao D., Zhang Q., Meng F.-L., Zhong H.-X., Shi M.-M., Zhang Y., et al. (2017). Electrochemical reduction of N2 under ambient conditions for artificial N2 fixation and renewable energy storage using N2/NH3 cycle. Adv. Mater. 29:1604799. doi: 10.1002/adma.201604799
2. Cao S., Low J., Yu J., and Jaroniec M. (2015). Polymeric photocatalysts based on graphitic carbon nitride. Adv. Mater. 27, 2150–2176. doi: 10.1002/adma.201500033
3. Chen C., Yan D., Wang Y., Zhou Y., Zou Y., Li Y., et al. (2019a). B-N pairs enriched defective carbon nanosheets for ammonia synthesis with high efficiency. Small 15:1805029. doi: 10.1002/smll.201805029
4. Chen J., Huang H., Xia L., Xie H., Ji L., Wei P., et al. (2019b). Oxygen-doped porous carbon nanosheet for efficient N2 fixation to NH3 at ambient conditions. ChemistrySelect 4, 3547–3550. doi: 10.1002/slct.201900253
5. Chu K., Li Q.-q., Liu Y.-p., Wang J., and Cheng Y.-h. (. (2020). Filling the nitrogen vacancies with sulphur dopants in graphitic C3N4 for efficient and robust electrocatalytic nitrogen reduction. Appl. Catal. B Environ. 267:118693. doi: 10.1016/j.apcatb.2020.118693
6. Chu K., Liu Y.-p., Wang J., and Zhang H. (2019). NiO nanodots on graphene for efficient electrochemical N2 reduction to NH3. ACS Appl. Energy Mater. 2, 2288–2295. doi: 10.1021/acsaem.9b00102
7. Fan Q., Choi C., Yan C., Liu Y., Qiu J., Hong S., et al. (2019). High-yield production of few-layer boron nanosheets for efficient electrocatalytic N2 reduction. Chem. Commun. 55, 4246–4249. doi: 10.1039/C9CC00985J
8. Feng J.-X., Xu H., Ye S.-H., Ouyang G., Tong Y.-X., and Li G.-R. (2017). Silica–polypyrrole hybrids as high-performance metal-free electrocatalysts for the hydrogen evolution reaction in neutral media. Angewandte Chemie Int. Ed. 56, 8120–8124. doi: 10.1002/anie.201702934
9. Guo C., Ran J., Vasileff A., and Qiao S.-Z. (2018). Rational design of electrocatalysts and photo(electro)catalysts for nitrogen reduction to ammonia (NH3) under ambient conditions. Energy Environ. Sci. 11, 45–56. doi: 10.1039/C7EE02220D
10. Hao Y.-C., Guo Y., Chen L.-W., Shu M., Wang X.-Y., Bu T.-A., et al. (2019). Promoting nitrogen electroreduction to ammonia with bismuth nanocrystals and potassium cations in water. Nat. Catal. 2, 448–456. doi: 10.1038/s41929–019-0241–7
11. Hu C., and Dai L. (2017). Multifunctional carbon-based metal-free electrocatalysts for simultaneous oxygen reduction, oxygen evolution, and hydrogen evolution. Adv. Mater. 29:1604942. doi: 10.1002/adma.201604942
12. Kandemir T., Schuster M. E., Senyshyn A., Behrens M., and Schlögl R. (2013). The Haber–Bosch process revisited: on the real structure and stability of “ammonia iron” under working conditions. Angewandte Chemie Int. Ed. 52, 12723–12726. doi: 10.1002/anie.201305812
13. Légaré M.-A., Bélanger-Chabot G., Dewhurst R. D., Welz E., Krummenacher I., Engels B., et al. (2018). Nitrogen fixation and reduction at boron. Science 359:896. doi: 10.1126/science.aaq1684
14. Li H., Shang J., Ai Z., and Zhang L. (2015). Efficient visible light nitrogen fixation with BiOBr nanosheets of oxygen vacancies on the exposed {001} facets. J. Am. Chem. Soc. 137, 6393–6399. doi: 10.1021/jacs.5b03105
15. Li L., Tang C., Xia B., Jin H., Zheng Y., and Qiao S.-Z. (2019). Two-dimensional mosaic bismuth nanosheets for highly selective ambient electrocatalytic nitrogen reduction. ACS Catalysis 9, 2902–2908. doi: 10.1021/acscatal.9b00366
16. Liu C., Li Q., Zhang J., Jin Y., MacFarlane D. R., and Sun C. (2018a). Theoretical evaluation of possible 2D boron monolayer in N2 electrochemical conversion into ammonia. J. Phys. Chem. C 122, 25268–25273. doi: 10.1021/acs.jpcc.8b10021
17. Liu S., Wang M., Qian T., Ji H., Liu J., and Yan C. (2019). Facilitating nitrogen accessibility to boron-rich covalent organic frameworks via electrochemical excitation for efficient nitrogen fixation. Nat. Commun. 10:3898. doi: 10.1038/s41467–019-11846-x
18. Liu X., and Dai L. (2016). Carbon-based metal-free catalysts. Nat. Rev. Mater. 1:16064. doi: 10.1038/natrevmats.2016.64
19. Liu Y., Su Y., Quan X., Fan X., Chen S., Yu H., et al. (2018b). Facile ammonia synthesis from electrocatalytic N2 reduction under ambient conditions on N-doped porous carbon. ACS Catalysis 8, 1186–1191. doi: 10.1021/acscatal.7b02165
20. Lv C., Qian Y., Yan C., Ding Y., Liu Y., Chen G., et al. (2018a). Defect engineering metal-free polymeric carbon nitride electrocatalyst for effective nitrogen fixation under ambient conditions. Angewandte Chemie Int. Ed. 57, 10246–10250. doi: 10.1002/anie.201806386
21. Lv C., Yan C., Chen G., Ding Y., Sun J., Zhou Y., et al. (2018b). An amorphous noble-metal-free electrocatalyst that enables nitrogen fixation under ambient conditions. Angewandte Chemie Int. Ed. 57, 6073–6076. doi: 10.1002/anie.201801538
22. Mu S., Chen X., Sun R., Liu X., Wu H., He D., et al. (2016). Nano-size boron carbide intercalated graphene as high performance catalyst supports and electrodes for PEM fuel cells. Carbon 103, 449–456. doi: 10.1016/j.carbon.2016.03.044
23. Mukherjee S., Cullen D. A., Karakalos S., Liu K., Zhang H., Zhao S., et al. (2018). Metal-organic framework-derived nitrogen-doped highly disordered carbon for electrochemical ammonia synthesis using N2 and H2O in alkaline electrolytes. Nano Energy 48, 217–226. doi: 10.1016/j.nanoen.2018.03.059
24. Nazemi M., Panikkanvalappil S. R., and El-Sayed M. A. (2018). Enhancing the rate of electrochemical nitrogen reduction reaction for ammonia synthesis under ambient conditions using hollow gold nanocages. Nano Energy 49, 316–323. doi: 10.1016/j.nanoen.2018.04.039
25. Pang J., Bachmatiuk A., Yin Y., Trzebicka B., Zhao L., Fu L., et al. (2018). Applications of phosphorene and black phosphorus in energy conversion and storage devices. Adv. Energy Mater. 8:1702093. doi: 10.1002/aenm.201702093
26. Qin Q., Heil T., Schmidt J., Schmallegger M., Gescheidt G., Antonietti M., et al. (2019). Electrochemical fixation of nitrogen and its coupling with biomass valorization with a strongly adsorbing and defect optimized boron–carbon–nitrogen catalyst. ACS Appl. Energy Mater. 2, 8359–8365. doi: 10.1021/acsaem.9b01852
27. Qiu W., Xie X.-Y., Qiu J., Fang W.-H., Liang R., Ren X., et al. (2018). High-performance artificial nitrogen fixation at ambient conditions using a metal-free electrocatalyst. Nat. Commun. 9:3485. doi: 10.1038/s41467–018-05758–5
28. Schloegl R. (2003). Catalytic synthesis of ammonia—A “never-ending story”? ChemInform 34:327199. doi: 10.1002/chin.200327199
29. Seh Z. W., Kibsgaard J., Dickens C. F., Chorkendorff I., Nørskov J. K., and Jaramillo T. F. (2017). Combining theory and experiment in electrocatalysis: insights into materials design. Science 355:eaad4998. doi: 10.1126/science.aad4998
30. Service R. F. (2014). New recipe produces ammonia from air, water, and sunlight. Science 345:610. doi: 10.1126/science.345.6197.610
31. Shen Z.-K., Yuan Y.-J., Pei L., Yu Z.-T., and Zou Z. (2020). Black phosphorus photocatalysts for photocatalytic H2 generation: a review. Chem. Eng. J. 386:123997. doi: 10.1016/j.cej.2019.123997
32. Singh A. R., Rohr B. A., Schwalbe J. A., Cargnello M., Chan K., Jaramillo T. F., et al. (2017). Electrochemical ammonia synthesis—the selectivity challenge. ACS Catalysis 7, 706–709. doi: 10.1021/acscatal.6b03035
33. Song S., Xu W., Cao R., Luo L., Engelhard M. H., Bowden M. E., et al. (2017). B4C as a stable non-carbon-based oxygen electrode material for lithium-oxygen batteries. Nano Energy 33, 195–204. doi: 10.1016/j.nanoen.2017.01.042
34. Song Y., Johnson D., Peng R., Hensley D. K., Bonnesen P. V., Liang L., et al. (2018). A physical catalyst for the electrolysis of nitrogen to ammonia. Sci. Adv. 4:e1700336. doi: 10.1126/sciadv.1700336
35. Su H.-Y., Gorlin Y., Man I. C., Calle-Vallejo F., Nørskov J. K., Jaramillo T. F., et al. (2012). Identifying active surface phases for metal oxide electrocatalysts: a study of manganese oxide bi-functional catalysts for oxygen reduction and water oxidation catalysis. Phys. Chem. Chem. Phys. 14, 14010–14022. doi: 10.1039/C2CP40841D
36. Suryanto B. H. R., Du H.-L., Wang D., Chen J., Simonov A. N., and MacFarlane D. R. (2019). Challenges and prospects in the catalysis of electroreduction of nitrogen to ammonia. Nat. Catalysis 2, 290–296. doi: 10.1038/s41929–019-0252–4
37. van der Ham C. J. M., Koper M. T. M., and Hetterscheid D. G. H. (2014). Challenges in reduction of dinitrogen by proton and electron transfer. Chem. Soc. Rev. 43, 5183–5191. doi: 10.1039/C4CS00085D
38. Wang S., Hai X., Ding X., Chang K., Xiang Y., Meng X., et al. (2017). Light-switchable oxygen vacancies in ultrafine Bi5O7Br nanotubes for boosting solar-driven nitrogen fixation in pure water. Adv. Mater. 29:1701774. doi: 10.1002/adma.201701774
39. Wang X., Blechert S., and Antonietti M. (2012). Polymeric graphitic carbon nitride for heterogeneous photocatalysis. ACS Catalysis 2, 1596–1606. doi: 10.1021/cs300240x
40. Wang Y., Shi M.-m., Bao D., Meng F.-l., Zhang Q., Zhou Y.-t., et al. (2019). Generating defect-rich bismuth for enhancing the rate of nitrogen electroreduction to ammonia. Angewandte Chemie Int. Ed. 58, 9464–9469. doi: 10.1002/anie.201903969
41. Wu S., Hui K. S., and Hui K. N. (2018). 2D black phosphorus: from preparation to applications for electrochemical energy storage. Adv. Sci. 5:1700491. doi: 10.1002/advs.201700491
42. Xia L., Yang J., Wang H., Zhao R., Chen H., Fang W., et al. (2019). Sulfur-doped graphene for efficient electrocatalytic N2-to-NH3 fixation. Chem. Commun. 55, 3371–3374. doi: 10.1039/C9CC00602H
43. Xie J., Yang X., and Xie Y. (2020). Defect engineering in two-dimensional electrocatalysts for hydrogen evolution. Nanoscale 12, 4283–4294. doi: 10.1039/C9NR09753H
44. Xie J., Zhao X., Wu M., Li Q., Wang Y., and Yao J. (2018). Metal-free fluorine-doped carbon electrocatalyst for CO2 reduction outcompeting hydrogen evolution. Angewandte Chemie Int. Ed. 57, 9640–9644. doi: 10.1002/anie.201802055
45. Xing C., Zhang J., Jing J., Li J., and Shi F. (2019). Preparations, properties and applications of low-dimensional black phosphorus. Chem. Eng. J. 370, 120–135. doi: 10.1016/j.cej.2019.03.177
46. Xu J., Chang Y., Gan L., Ma Y., and Zhai T. (2015). Ultrathin single-crystalline boron nanosheets for enhanced electro-optical performances. Adv. Sci. 2:1500023. doi: 10.1002/advs.201500023
47. Yang L., Jiang S., Zhao Y., Zhu L., Chen S., Wang X., et al. (2011). Boron-doped carbon nanotubes as metal-free electrocatalysts for the oxygen reduction reaction. Angewandte Chemie Int. Ed. 50, 7132–7135. doi: 10.1002/anie.201101287
48. Yu X., Han P., Wei Z., Huang L., Gu Z., Peng S., et al. (2018). Boron-doped graphene for electrocatalytic N2 reduction. Joule 2, 1610–1622. doi: 10.1016/j.joule.2018.06.007
49. Zhang L., Ding L.-X., Chen G.-F., Yang X., and Wang H. (2019a). Ammonia synthesis under ambient conditions: selective electroreduction of dinitrogen to ammonia on black phosphorus nanosheets. Angewandte Chemie Int. Ed. 58, 2612–2616. doi: 10.1002/anie.201813174
50. Zhang X., Wu T., Wang H., Zhao R., Chen H., Wang T., et al. (2019b). Boron nanosheet: An elemental two-dimensional (2D) material for ambient electrocatalytic N2-to-NH3 fixation in neutral media. ACS Catalysis 9, 4609–4615. doi: 10.1021/acscatal.8b05134
51. Zhang Y., Du H., Ma Y., Ji L., Guo H., Tian Z., et al. (2019c). Hexagonal boron nitride nanosheet for effective ambient N2 fixation to NH3. Nano Res. 12, 919–924. doi: 10.1007/s12274–019-2323-x
52. Zhao S., Lu X., Wang L., Gale J., and Amal R. (2019). Carbon-based metal-free catalysts for electrocatalytic reduction of nitrogen for synthesis of ammonia at ambient conditions. Adv. Mater. 31:1805367. doi: 10.1002/adma.201805367
Keywords: two-dimensional, metal-free, electrocatalyst, N2 fixation, Faradaic efficiency
Citation: Xia X, Li B, Liu S and Tang B (2020) Recent Advances and Challenges in 2D Metal-Free Electrocatalysts for N2 Fixation. Front. Chem. 8:437. doi: 10.3389/fchem.2020.00437
Received: 04 April 2020; Accepted: 27 April 2020;
Published: 10 June 2020.
Edited by:
Kun Xu, Nanyang Technological University, SingaporeReviewed by:
Youwen Liu, Huazhong University of Science and Technology, ChinaLingfeng Gao, University of Jinan, China
Copyright © 2020 Xia, Li, Liu and Tang. This is an open-access article distributed under the terms of the Creative Commons Attribution License (CC BY). The use, distribution or reproduction in other forums is permitted, provided the original author(s) and the copyright owner(s) are credited and that the original publication in this journal is cited, in accordance with accepted academic practice. No use, distribution or reproduction is permitted which does not comply with these terms.
*Correspondence: Xinyuan Xia, dm9lZ2xlaW5uZSYjeDAwMDQwOzE2My5jb20=; Bo Tang, dGFuZ2ImI3gwMDA0MDtzZG51LmVkdS5jbg==