- Industrial Chemistry Laboratory, Department of Chemistry, National and Kapodistrian University of Athens, Athens, Greece
Levulinic acid (LA) is classified as a key platform chemical for the development of future biorefineries, owing to its broad spectrum of potential applications and because it is simply available from lignocellulosic biomass through inexpensive and high-yield production routes. Catalytic hydrogenation reactions of LA into the pivotal intermediate compound γ-valerolactone (GVL), and beyond GVL to yield valeric acid (VA), 1,4-pentanediol (1,4-PDO), and 2-methyltetrahydrofuran (2-MTHF) have gained considerable attention in the last decade. Among the various transition metals used as catalysts in LA hydrogenation reactions, ruthenium-based catalytic systems have been the most extensively applied by far, due to the inherent ability of ruthenium under mild conditions to hydrogenate the keto functionality of LA selectively into an alcohol group to form 4-hydroxyvaleric acid intermediate, which yields GVL spontaneously after dehydration and cyclization. This review focuses on recent advances in the field of aqueous-phase ruthenium-catalyzed hydrogenation reactions of LA toward GVL, VA, 1,4-PDO, 2-MTHF, 2-pentanol, and 2-butanol. It employs heterogeneous catalysts on solid supports, and heterogeneous water-dispersible catalytic nanoparticles or homogeneous water-soluble catalytic complexes with biphasic catalyst separation, for the inter alia production of advanced biofuels such as valeric biofuels and other classes of liquid transportation biofuels, value-added fine chemicals, solvents, additives to gasoline, and to food as well. The significance of the aqueous solvent to carry out catalytic hydrogenations of LA has been highlighted because the presence of water combines several advantages: (i) it is highly polar and thus an ideal medium to convert polar and hydrophilic substrates such as LA; (ii) water is involved as a byproduct; (iii) the presence of the aqueous solvent has a beneficial effect and enormously boosts hydrogenation rates. In sharp contrast, the use of various organic solvents gives rise to a dramatic drop in catalytic activities. The promotional effect of water was proven by numerous experimental investigations and several theoretical studies employing various types of catalytic systems; (iv) the large heat capacity of water renders it an excellent medium to perform large scale exothermic hydrogenations more safely and selectively; and (v) water is a non-toxic, safe, non-inflammable, abundantly available, ubiquitous, inexpensive, and green/sustainable solvent.
Introduction
Currently, renewable biomass as a raw material is considered to be the grand challenge in the development of Green-Sustainable chemistry which decisively contributes to the transition from a fossil-based society to a bio-resources based economy. Biomass possesses a high potential as a raw material that sustainable biorefineries can use to manufacture biofuels, bio-based chemicals, solvents, materials, energy, power, pharmaceuticals, and food. The use of biomass as an industrial feedstock also combines environmental benefits because it mitigates local air pollution and the global warming problems caused by greenhouse gas emissions. Moreover, the use of biomass is associated with further economic, environmental, social, health and safety benefits which are based, inter alia, on the renewable nature of biomass, contrasted to the nature of fossil raw materials which possess a limited capacity in the domestic production of biofuels, and thus on the country's independence from fossil feedstocks, on the profits of local agriculture, on the implementations of financial incentives, and on biodegradability and biocompatibility, as well as on the mechanical and physicochemical properties of biomass-based products. The high potential of biomass is even more remarkable when one considers that the nature's global biomass production capacity is huge, namely about 2.0·1011 t/a compared to only 7·109 t/a of all extracted fossil fuels, and furthermore that only 3.3% of the annual biomass production capacity is used for food, feed, and non-food applications (Van Bekkum and Gallezot, 2004; Sheldon, 2014; Li et al., 2018; Badgujar et al., 2020). Biomass consists of about 75% carbohydrates, 20% lignin, and 5% triglycerides, i.e., fats and oils, terpenes and proteins (Sheldon, 2014). Storage carbohydrates include the polysaccharides starch and inulin and the disaccharide sucrose. However, the most abundant biomass is lignocellulose, the constituent of plant cell walls, which is not edible and therefore without competition in food and consists of 40–50% cellulose, 25–35% hemicellulose, and 15–20% lignin (Climent et al., 2014). Cellulose is a linear biopolymer consisting entirely of glucose units linked by β-1,4-glycosidic bonds. The presence of extensive hydrogen bonding of both types inter- and intra-chain bonds imparts crystallinity to cellulose and strength to the structure of the plant, which makes cellulose recalcitrant to chemical or enzymatic hydrolysis. Cellulose is the organic compound with the largest capacity in the world. Hemicellulose is an amorphous branched biopolymer composed of C5 and C6 sugars with the major compound being xylose. Lignin is a cross-linked polyphenolic amorphous biopolymer surrounding hemicellulose and cellulose. The rigidity and complexity of the lignin structure gives recalcitrance to the cell walls of the plant, which makes difficult the pretreatment of lignocellulosic biomass for the liberation of cellulose and hemicellulose to be applied as feedstocks for the production of fuels, platform chemicals and materials.
One of the most promising platform chemicals obtained from lignocellulose is levulinic acid (LA, Figure 1), which was selected by the US Department of Energy (DOE) as one of the top twelve platform chemicals according to its report released in 2004 (Werpy and Petersen, 2004), as well as to the updated, revised and extended DOE's report in 2010 (Bozell and Petersen, 2010). LA is classified as a key platform chemical for the development of future biorefineries because of its broad spectrum of potential applications and because it is simply available with inexpensive and high yield production routes from lignocellulose biomass (Tang et al., 2014; Ye et al., 2020). The first industrial continuous process for the production of LA was developed by BioMetics Inc. in the earlies 1990's, which was well-known as the Biofine process, and proceeds in a proprietary two-reactor system using lignocellulose biomass as feedstock together with a sulfuric acid solution as a reagent. After the deconstruction of lignocellulose, sulfuric acid-catalyzed hydrolysis of cellulose and hemicellulose to yield C6 and C5 carbohydrates such as glucose and xylose takes place in the first reactor (Figure 1). Glucose is further isomerized into fructose and after dehydration of fructose the 5-hydroxymethylfurfural intermediate (HMF, Figure 1) is obtained under conditions to minimize polymerization side reactions, which yield undesired insoluble side products called humins. HMF is continuously removed and subsequently introduced as feedstock in the second reactor where, after hydration reactions, LA is produced with formic acid (Figure 1). The solid humins are separated from the solution of levulinic acid and burnt to generate heat and electricity for the process. From dehydration reactions of C5 carbohydrates e.g., xylose, furfural is produced. This is separately collected and acts as an intermediate after hydrogenation to furfuryl alcohol and hydration to also produce LA (Figure 1). The route of hemicellulose to LA possesses the advantage of milder acidic conditions and therefore of lower formation of undesired humins, because of the easier hydrolysis step of amorphous hemicellulose into C5 and C6 carbohydrates compared to the hydrolysis step of crystalline and recalcitrant cellulose into glucose intermediate, which is further isomerized into fructose and in the acid-catalyzed dehydration step to HMF unavoidable higher amounts of humins are obtained. The process engineering of Biofine was revised and redesigned by the GFBiochemicals company, which developed an acid catalyzed industrial process (Atlas TechnologyTM) for the manufacture of LA from lignocellulose biomass with a production capacity of 10,000 t/a. Several other industrial companies produce LA worldwide using lignocellulosic biomass as feedstock (Huber et al., 2006; Bozell and Petersen, 2010; Serrano-Ruiz et al., 2012; Bond et al., 2014; Climent et al., 2014; Leitner et al., 2017; Makhubela and Darkwa, 2018; Home page of GFBiochemicals company., 2019).
Among the various routes for the valorization of LA, the catalytic hydrogenation route has been considered as an important pathway that is gaining more interest in recent years because of the wide range of potential applications of the LA's hydrogenation products, which include, inter alia, advanced biofuels such as valeric biofuels and other classes of liquid transportation biofuels, fine chemicals, solvents, additives to gasoline as well as additives to food. In the last decade, the hydrogenation reactions of LA into γ-valerolactone (GVL, Figure 2), which is a key intermediate compound, and beyond GVL to yield 1,4-pentanediol (1,4-PDO, Figure 2) and valeric acid (VA, Figure 2), have gained considerable attention. Numerous heterogeneous catalytic systems based on precious and non-noble metals and water-soluble transition metal catalytic complexes have been developed in the absence and presence of organic or aqueous solvents (Serrano-Ruiz et al., 2011, 2012; Tang et al., 2014; Yan et al., 2015a,b; Omoruyi et al., 2016; Pileidis and Titirici, 2016; Osatiashtiani et al., 2017; Makhubela and Darkwa, 2018; Xue et al., 2018; Dutta et al., 2019; Yu et al., 2019; Ye et al., 2020). The catalytic hydrogenation reaction of LA in the aqueous solvent is a more attractive and promising processing mode because the use of water combines several advantages: (i) the highly polar nature of the aqueous solvent makes it an ideal medium to convert polar, with high oxygen content, and hydrophilic platform chemicals such as the water-soluble starting material LA; (ii) water is involved as a byproduct to obtain the GVL intermediate, which is further hydrogenated into 1,4-PDO in the aqueous medium and, after dehydration and cyclization reactions 2-methyltetrahydrofuran (2-MTHF, Figure 2), is formed creating an aqueous/organic two-phase system which provides for the easy separation of the polar aqueous reaction medium from a polar organic product 2-MTHF by a simple phase separation. The same procedure could also be applied in the aqueous/organic biphasic system created with alkyl valerates obtained from LA hydrogenation product valeric acid (VA, Figure 2) after esterification with alcohols. This biphasic processing mode results in substantial energy savings, lower emissions and economic benefits; (iii) novel types of catalytic reactivities have been observed in water: in the hydrogenation reaction of LA into GVL, and beyond in the aqueous solvent employing conventional heterogeneous catalytic systems, catalytic nanoparticles, and water-soluble transition metal catalytic complexes, the presence of water has a beneficial effect and accelerates reaction rates, whereas in organic solvents much lower activities were observed. This promotional effect of water in the hydrogenation reaction of LA was proved by several experimental and theoretical studies using various types of catalytic systems; (iv) the large heat capacity of water makes it an excellent medium to perform exothermic reactions, such as the catalytic hydrogenation reaction of LA, more safely and selectively—which is especially important in large scale industrially-applied catalytic hydrogenation processes of LA; (v) water is a non-toxic, non-inflammable, abundantly available, inexpensive, ubiquitous, green and sustainable solvent.
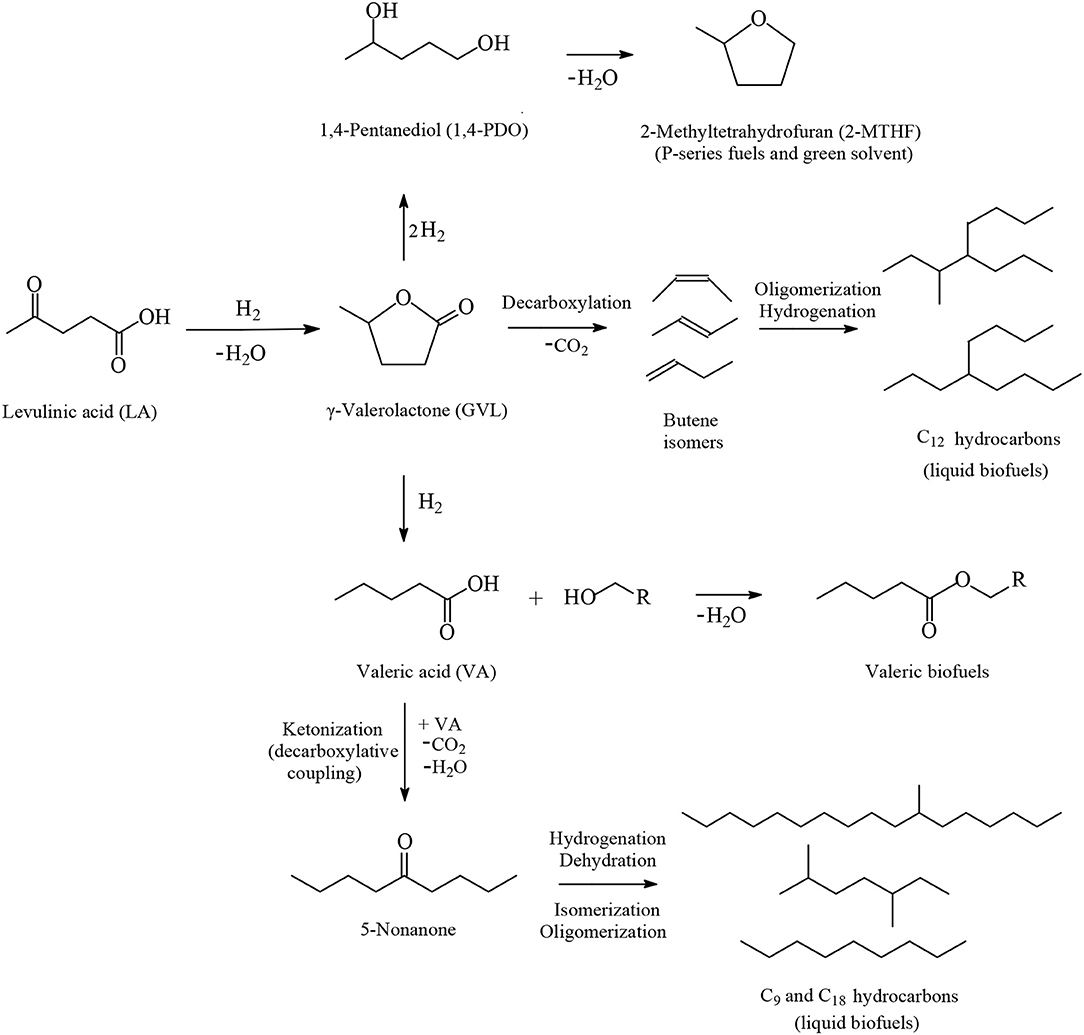
Figure 2. Routes based on the catalytic hydrogenation of LA to obtain advanced biofuels, chemicals and solvents.
In the LA hydrogenation reaction, ruthenium-based catalytic systems have been by far more extensively applied by than their catalytic counterparts based on other transition metals. This is because of the inherent and unique ability of ruthenium under mild reaction conditions to effectively hydrogenate the keto moiety of LA into an alcohol functionality to form the 4-hydroxyvaleric acid intermediate which spontaneously after dehydration and cyclization yields GVL. This review focuses on recent advances in the field of aqueous-phase ruthenium-catalyzed hydrogenation reactions of LA into GVL and beyond, to obtain VA, 1,4-PDO and 2-MTHF as well as 2-pentanol and 2-butanol employing heterogeneous catalytic systems on solid supports, heterogeneous water-dispersible catalytic nanoparticles and homogeneous water-soluble catalytic complexes for the production, inter alia, of advanced biofuels such as valeric biofuels and other classes of liquid transportation biofuels, value-added fine chemicals, solvents, additives to gasoline and to food as well. The significance of the aqueous solvent in such catalytic hydrogenation reactions has been also highlighted.
Hydrogenation of LA Into Value-Added Chemicals and Advanced Biofuels
The catalytic hydrogenation reaction of LA yields GVL (Figure 2), which is an important C5 platform chemical and pivotal intermediate compound for the efficient conversion of LA to advanced biofuels of various classes, chemicals and solvents by several different routes as depicted in Figure 2. GVL is also used as an aprotic, polar, and sustainable solvent, as an additive to gasoline and suitable for use as a food additive. Hydrogenation and ring-opening reactions of GVL afford 1,4-PDO and VA intermediates (Figure 2). 1,4-PDO tends to undergo dehydration and cyclization reactions to yield 2-methyltetrahydrofuran (2-MTHF, Figure 2) which is considered as a green solvent (Yan et al., 2010, 2015a,b; Serrano-Ruiz et al., 2012) with a polarity placed between tetrahydrofuran and diethyl ether possessing, however, a potential to form explosive peroxides under air (Aycock, 2007; Fábos et al., 2009; Byrne et al., 2017). 2-MTHF is one of the three components of an alternative type of fuel, namely P-series fuels, that are approved by DOE as fuels that can substitute gasoline. Furthermore, 2-MTHF could be blended up to 70% in conventional gasoline fuels (Yan et al., 2010, 2015a,b; Serrano-Ruiz et al., 2012). Esterification reactions of VA with alcohols produce alkyl valerate advanced biofuels well-known as valeric biofuels, which are suitable to be blended with gasoline or diesel fuels depending on the length of the alkyl chain of added alcohol (Figure 2). When shorter chain alcohols such as methanol and ethanol are used in the esterification of VA the methyl and ethyl valerate products are suitable as gasoline fuel, whereas with longer chain alcohols such as butanol and pentanol, the butyl and pentyl valerates are more appropriate as diesel fuel with excellent energy density as well as volatility and ignition properties (Lange et al., 2010; Yan et al., 2010, 2015a,b; Serrano-Ruiz et al., 2012). VA could alternatively be used as starting material in decarboxylative coupling reactions i.e., ketonization reactions to produce 5-nonanone, which is an interesting platform to be applied as starting material in hydrogenation, dehydration, isomerization, and oligomerization reactions for the manufacture of C9 and C18 linear and branched hydrocarbons which are gasoline, diesel, and jet biofuels (Figure 2) (Serrano-Ruiz et al., 2012; Simakova and Murzin, 2016). Another route for the production of jet biofuels is based on GVL, which in the first step undergoes decarboxylation into butenes, followed by oligomerization to form higher alkenes which after hydrogenation are suitable as aviation biofuels (Figure 2) (Bond et al., 2010, 2014).
Hydrogenation of LA Into GVL
GVL is a water-soluble, non-toxic liquid that is stable at neutral pH in aqueous solvent and air without any formation of a measurable amount of peroxides when in a glass flask exposed to the air for several weeks, making it safe for industrial scale use. Furthermore, GVL does not form an azeotropic mixture with the aqueous solvent and therefore the manufacture of GVL constitutes a less energy demanding process compared to that for the production of absolute ethanol. Two possible reaction pathways were proposed for the synthesis of GVL from LA which are shown in Figure 3. According to the first pathway the keto functionality of LA is hydrogenated probably by heterolytic H2 cleavage into an alcohol group to form the 4-hydroxyvaleric acid intermediate, which then readily undergoes dehydration to give, by favorable intramolecular esterification, the cyclic ester product GVL (Figures 3, 4). The heterolytic dihydrogen cleavage mechanism may be facilitated by heterogenous catalytic systems comprising a transition metal and an oxide as support, such as Ru/TiO2, Ru/Al2O3, by the interaction between the metal with the support in the way that on the surface of transition metal is formed the H− moiety of dihydrogen and on the oxygen atom of the support the H+ moiety (Kubas, 2005). The second possible pathway of Figure 3 involves acid-catalyzed endothermic dehydration of LA at reaction temperatures higher than 180°C to form α-angelica lactone by intramolecular esterification, which is subsequently hydrogenated to yield GVL. In both mechanisms depicted in Figure 3 the hydrogenation steps are influenced by the nature and activity of the transition metal of the catalysts, whereas the dehydration steps depend on the acidity of the catalysts and of the medium. At elevated temperatures and/or in the presence of acidic heterogeneous catalytic systems the dehydration reaction of LA is promoted to give α-angelica lactone, which easily polymerizes on acidic surfaces to form coke, resulting in a severe deactivation of the heterogeneous catalytic system.
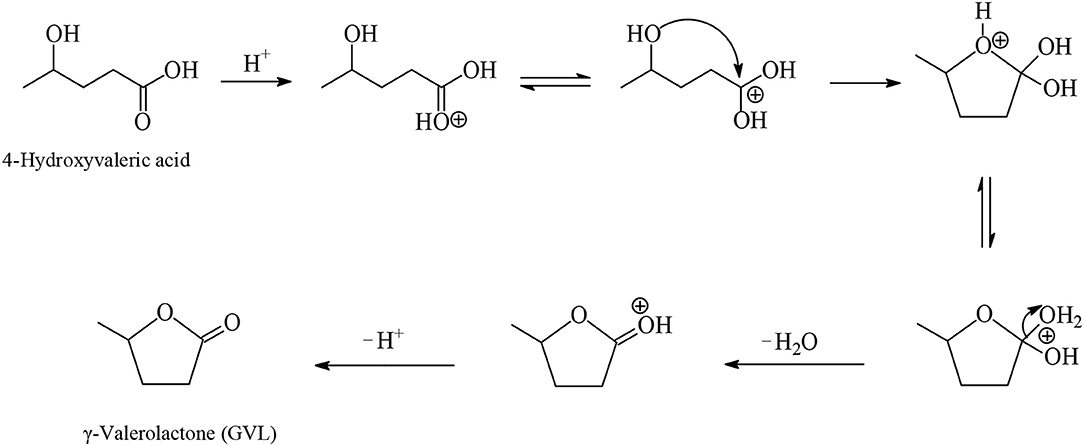
Figure 4. Proposed mechanism for the dehydration of 4-hydroxyvaleric acid intermediate to yield by intramolecular esterification GVL (Ruppert et al., 2015).
Heterogeneous Catalysts on Solid Supports
Numerous heterogeneous ruthenium-based catalytic systems have been successfully applied in the hydrogenation reaction of LA into GVL usually employing relatively more forcing conditions, i.e., higher temperatures and dihydrogen pressures in aqueous media. The use of heterogeneous catalysts combines the easy catalyst separation from reaction products and catalyst recycling with the possibility to operate easily in a continuous process.
Tan et al. (2015) synthesized ruthenium-supported catalysts with low 0.5 wt.%, moderate 1 wt.% and higher 2 wt.% ruthenium loading on various oxides such as Al2O3, SiO2, ZrO2, and TiO2 and investigated their catalytic activity and selectivity on the hydrogenation reaction of LA into GVL at molar ratios of LA/Ru between 8,707 and 2,177, reaction temperatures of 130 and 70°C under 40 bar of dihydrogen pressure in aqueous and organic media. With a higher ruthenium loading of 2 wt.% all supported catalysts at 130°C exhibited a TOF value of 4,353 per hour with quantitative conversions of LA. At a low ruthenium loading of 0.5 wt.% the Ru/TiO2 catalytic system exhibited at 130°C within 3 h reaction time the highest catalytic activity of TOF = 2,769 h−1 with a conversion of LA of 95.4 mol%, compared with the other ruthenium-supported catalysts under the same conditions which have shown lower activities with e.g., 0.5 wt.% Ru/Al2O3 the catalytic activity has been dropped down to 401 TOF's per hour and the conversion of LA to 13.8 mol% (Table 1). A much higher catalytic activity of TOF = 7,662 h−1 has been obtained with Ru/TiO2 catalysts with a moderate ruthenium loading of 1 wt.% even at a lower temperature of 70°C within a 15 min reaction time in the aqueous solvent. The higher catalytic activity of Ru/TiO2 could be explained by both the small size of ruthenium nanoparticles and the better dispersion of the metal on the support, which was proven by TEM analysis in showing average size of ruthenium nanoparticles on the TiO2 support were smaller, namely 2.0 nm, and more uniform compared with their counterparts on the ZrO2 support. Furthermore, the nature of the support plays a crucial role in the heterogeneously ruthenium-catalyzed LA hydrogenation reaction, because the support activates the C=O functionality of LA and ruthenium nanoparticles dissociates dihydrogen. It is highly probable that a strong interaction between small ruthenium particles and TiO2 support takes place which, after activation of the carbonyl moiety on TiO2, generates highly active sites on the coordination sphere of the metal by catalytic relevant ruthenium species for efficient H2 dissociation. With all ruthenium-supported catalysts under various reaction conditions in aqueous medium, the only product obtained was GVL with essentially quantitative selectivity. A remarkable solvent effect has been observed in the LA hydrogenation reaction with 1 wt.% Ru/TiO2 catalysts at 130°C and 40 bar in the presence of aqueous and organic solvents. When water was used as a solvent in the LA hydrogenation the catalytic activity was exceptionally high and reached a value of TOF = 8,707 h−1, whereas with organic solvents such as ethanol and 1,4-dioxane, the catalytic activity dramatically drops down to 3,261 and 1,337 TOF's per hour, respectively, under the same reaction conditions (Table 1). The promotional effect of water was rationalized by assuming that water acts not only as a solvent but also participates as a reactant after dissociation in this aqueous-phase catalytic hydrogenation reaction. To verify this assumption, Tan et al. carried out the LA hydrogenation reaction in D2O and found by NMR and GC/MS analysis of the products that a D atom was bonded to the γ-C atom of the GVL product, which originates not from the dihydrogen reactant but from D2O solvent (Figure 5). Furthermore, the authors mixed GVL with D2O without a D atom to be detected on γ-C atom of GVL, and ruled out an alternative pathway of the incorporation of the D atom in GVL during the hydrogenation reaction of LA in D2O,
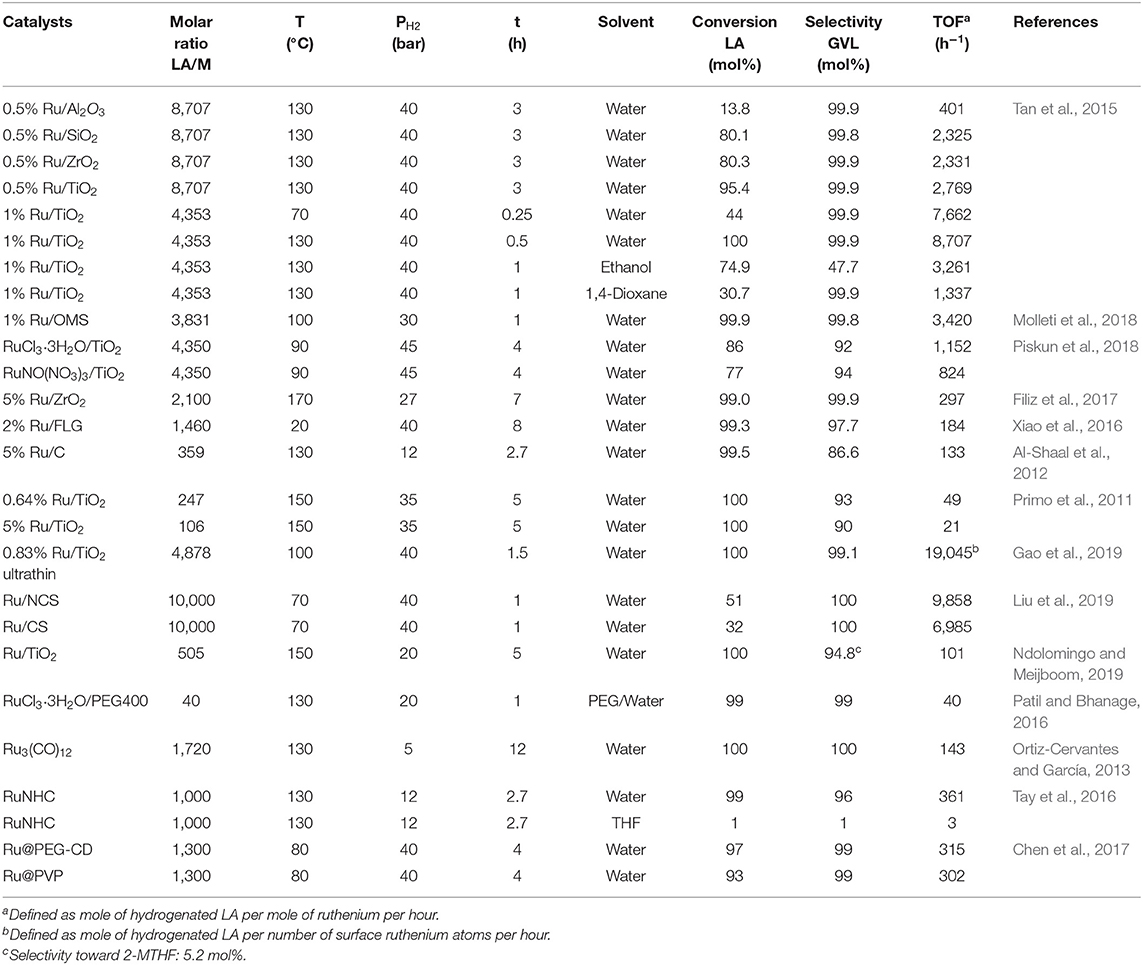
Table 1. Ruthenium-based heterogeneous catalysts on solid supports and water-dispersible catalytic nanoparticles for the hydrogenation of LA toward GVL.
which might be due to an H/D exchange between D2O and GVL. Thus, is proven that the promotional effect of the aqueous solvent in the LA hydrogenation is based on the involvement of water after dissociation as a reactant in the mechanism of this aqueous-phase catalytic reaction. Michel et al. (2014) applied Ru/TiO2, Pt/TiO2, and Pd/TiO2 catalysts possessing a similar metal particle size between 2.1 and 3.2 nm with an uniform distribution of each metal on the titania support in the LA hydrogenation reaction to GVL, under mild reaction conditions of 70°C and 50 bar dihydrogen pressure, using water and tetrahydrofuran as solvents. The catalytic activity of Ru/TiO2 is strongly influenced by the nature of reaction solvent. In the aqueous medium Ru/TiO2 catalysts exhibited the highest catalytic activity to obtain essentially quantitative conversions of LA with a yield of 95 mol% of GVL, whereas in organic solvents such as tetrahydrofuran the Ru/TiO2 system shows no catalytic activity in this hydrogenation reaction. In sharp contrast, the solvent did not influence the catalytic activities of Pt/TiO2 and Pd/TiO2 to obtain with Pt/TiO2 catalysts in both aqueous and organic medium low conversions of LA in the range of 20 up to 30 mol % and yields of GVL between 15 and 20 mol%. A very low catalytic activity has been observed with Pd/TiO2 catalysts under these mild reaction conditions. To explain the obtained experimental results, the authors performed Density Functional Theory (DFT) calculations using acetone as a model substrate containing a C=O functionality chemisorbed on Ru(0001) surface, and proved that the nature of the aqueous solvent is the reason for the higher activity of Ru/TiO2 catalysts in water, because the hydrogen bond of a chemisorbed water molecule on Ru-surface formed with the oxygen atom of an adjacent chemisorbed alkoxy intermediate involved in the reaction mechanism enormously lowers the activation energy barrier of the reaction pathway, resulting in enhanced reaction rates among Ru/TiO2-catalyzed hydrogenation reactions in aqueous media. It should be mentioned that in these DFT calculations the higher activity of ruthenium catalysts in the hydrogenation of acetone in aqueous media was attributed to the formation of hydrogen bonds between chemisorbed water and carbonyl intermediate compounds, and in these calculations the possibility that water may also act as a reactant after dissociation on the ruthenium surface has not been considered. Michel and Gallezot (2015) pointed out that in order to be able to understand the high catalytic activity of ruthenium compared with other transition metals in hydrogenation reactions of starting materials bearing C=O functionalities in water where two mechanisms are operative (the first one includes chemisorbed water molecules which, by hydrogen bonding, lower the energetic span of the reaction pathway to yield the –CH2OH functionality by dissociation of hydrogen, and the second one involves dissociation of water to produce hydrogen atoms to participate in the hydrogenation reaction of the C=O group into a –CH2OH moiety), it is necessary to carry out more isotope labeling experiments and also to perform further improved theoretical investigations including both of these mechanisms. Continuous aqueous-phase hydrogenation reactions of LA in a fixed-bed reactor have been carried out over Ru/C, Ru/Al2O3 and Ru/MgO catalysts at a high temperature of 275°C under atmospheric pressure of dihydrogen, and the results revealed that Ru/C catalysts exhibited higher conversion of LA with higher selectivity toward GVL compared with Ru/Al2O3 and Ru/MgO catalytic systems (Velisoju et al., 2018). The authors further investigated the role of water on the activity over Ru/C catalysts in the continuous hydrogenation of LA toward GVL using various concentrations of LA in water from 70 wt.% down to 5 wt.%, and found that with increasing amounts of water increased both the conversion of LA and the selectivity to GVL, employing probe-adsorbed diffuse reflectance infrared Fourier transform (DRIFT) spectroscopy. Velisoju et al. found that -OH groups were generated in the presence of water on the surface of ruthenium of Ru/C catalyst. According to their proposed mechanism such hydroxyl groups initiate the dehydration step of the enol form of LA to yield α-angelica lactone intermediate, and after isomerization to β-angelica lactone surface ruthenium metal hydride species are responsible for the hydrogenation step of the -C=C- functionality of β-angelica lactone to form GVL (Figure 6). Therefore, the higher catalytic activity of Ru/C catalysts in the presence of water in the vapor phase is because of the easier generation of –OH groups on the surface of ruthenium, and also due to the presence of a larger active ruthenium metal surface area in comparison to the other Ru/Al2O3 and Ru/MgO catalysts. Mamun et al. (2019) studied solvent effects on the reaction kinetics of the hydrogenation reaction of LA into GVL over Ru(0001) catalysts under various conditions, using three media of different polarity—namely water, methanol, and 1,4-dioxane. The presence of the aqueous solvent enormously facilitated the hydrogenation reaction kinetics and this solvent effect is much stronger at temperatures lower than 100°C. For example, at the low reaction temperature of 50°C, a rate increase of 4 orders of magnitude was obtained due to the solvation effect of water. The strongest solvent effect has been observed in the highly polar solvent water and this effect decreases with decreasing polarity of the solvent, i.e., in the order: water > methanol > 1,4-dioxane. Various transition metals such as ruthenium, palladium, platinum, and nickel supported on hydroxyapatite (HAP) have been applied as catalysts in the liquid phase hydrogenation reaction of LA to GVL at 70°C under 5 bar H2 pressure in various solvents such as water, ethanol, and toluene (Sudhakar et al., 2014). The highest catalytic activity exhibited ruthenium catalysts and the rates decrease in the order: Ru > Pt > Pd > Ni. With Ru/HAP catalysts the highest activity has been found to be in the aqueous solvent and the catalytic activity decreased with decreasing polarity of the solvent: water > ethanol > toluene. Sudhakar et al. (2016) further examined the activity of ruthenium, palladium, platinum copper, and nickel catalysts supported on HAP in the vapor phase hydrogenation of LA in the presence of water. The obtained rates with such catalysts have the following order: Ru > Pt > Cu > Pd > Ni. At the reaction temperature of 275°C in the Ru/HAP-catalyzed hydrogenation in the presence of water the LA conversion was 65.1 mol% and the selectivity toward GVL 99.8 mol%. In sharp contrast in the absence of water under the same conditions the conversion of LA gives rise to a dramatic drop to 4.0 mol%, and the only product observed was α-angelica lactone. At the higher temperature of 425°C in the presence of water with Ru/HAP catalysts, the conversion of LA increased to 94.0 mol% with the selectivity to GVL to decrease to 80.5 mol% with concomitant formation of α-angelica lactone (13.0 mol%) and β-angelica lactone (5.5 mol%). Ru/HAP catalysts showed a better activity in the presence of water in comparison to organic solvents such as ethanol and methanol. Gundekari and Srinivasan (2019) prepared α novel hydrous ruthenium oxide (HRO) catalyst precursor by a precipitation method using an aqueous solution of RuCl3·3H2O with CaCO3 and applied HRO systems in the hydrogenation reaction of LA under mild conditions of 50°C and 10 bar dihydrogen pressure to obtain quantitative conversions of LA and yields toward GVL within 30 min in the aqueous solvent. The HRO precursor which possess water molecules strongly bonded to RuOx surface was reduced to catalytic active Ru(0) species under hydrogenation reaction conditions. Quantitative conversions of LA by HRO catalysts precursors were obtained only in the presence of the aqueous solvent, whereas in organic solvents such as THF and methanol the conversions of LA were very low, i.e., between 2 and 5 mol% at 100°C, 10 bar dihydrogen within 15 min reaction time. HRO catalyst precursors supported on H-β zeolite could be recycled in five consecutive runs with the Ru-HRO/H-β catalyst to retain its activity. Guo et al. (2016) investigated the catalytic properties of Ru@MIL-101(Cr) and Ru@MIL-100(Cr) Metal-Organic Frameworks as well as of Ru@HY-zeolite (Si/Al ≥ 5.2) in the hydrogenation of LA into GVL in aqueous media. The highest catalytic activity was exhibited by the Ru@MIL-101(Cr) system to obtain quantitative conversion of LA with an essentially quantitative selectivity toward GVL under mild reaction conditions at 70°C under 10 bar dihydrogen pressure within 5 h reaction time in water. The authors investigated the catalytic behavior of Ru@MIL-101(Cr) in the presence of water and various organic solvents. The strongest solvent effect, i.e., the highest catalytic activity, has been observed in the highly polar aqueous solvent. This effect decreases with decreasing polarity of the solvent, in the order: water > methanol > ethanol > 2-propanol > 1,4-dioxane. Ultrafinely dispersed ruthenium nanoparticles with a mean size of 2.1 nm have been supported on N-doped hierarchically-porous carbon (Ru/NHPC) with the support to be prepared from cellulose, and applied as catalysts in the aqueous-phase hydrogenation of LA to obtain higher activities, selectivity toward GVL, and (especially) stabilities compared with conventional Ru/C catalytic systems (Wei et al., 2018). Recycling experiments have shown that the Ru/NHPC catalytic system could be reused in thirteen consecutive hydrogenation runs at 50°C, 10 bar H2 pressure within 3 h in aqueous media without any obvious deactivation, whereas the Ru/C catalyst under identical conditions was severely deactivated already in the 3rd recycling consecutive run. The authors further compared the catalytic activity of Ru/NHPC systems in water and different organic solvents such as DMF, 1,4-dioxane, cyclohexane, methanol, and ethanol, and found that the highest yields toward GVL were obtained in water in comparison to all used organic solvents.
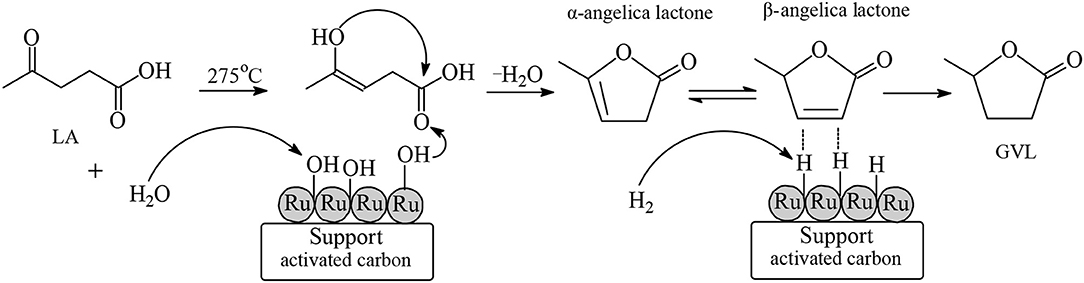
Figure 6. Proposed mechanism for the hydrogenation of LA to GVL at 275°C on the surface of Ru/C catalyst in aqueous media.
In studies of ruthenium-based catalytic systems a main focus is on the supports, with the aim to improve the efficiency of the catalyst via pre-treatment methods, modification of the nature and control of the properties, and the structure of the support material. Novel ruthenium supported on manganese oxide octahedral molecular sieve (Ru/OMS) systems have been synthesized and applied as catalysts in the hydrogenation of LA at 100°C under 30 bar dihydrogen pressure and a molar ratio of LA/Ru = 3,831 within 1 h in aqueous media (Molleti et al., 2018). Under these conditions the catalytic activity exhibited Ru/OMS catalyst was high (TOF = 3,420 h−1) to give near quantitative conversion of LA and selectivity toward GVL with retained catalytic activity in four recycling consecutive experiments (Table 1). This high catalytic activity exhibited Ru/OMS catalysts could be explained by the moderate acidity of the novel supported system and the high dispersion of ruthenium nanoparticles on the surface. The apparent activation energy of Ru/OMS catalyst in the aqueous-phase hydrogenation of LA was calculated and amounts to only 49.16 kJ/mol. Piskun et al. (2018) have shown that the performance of Ru/TiO2 (anatase) catalysts applied in the aqueous-phase hydrogenation of LA to GVL strongly depends on the synthesis protocol of the catalytic system by varying the nature of the ruthenium precursor, the calcination and/or reduction step, and the amount of dihydrogen in the gas during the reduction step. The highest initial reaction rates in the aqueous-phase hydrogenation of LA to GVL at 90°C and 45 bar dihydrogen pressure were obtained with RuCl3·3H2O catalysts precursors compared to RuNO(NO3)3 precursors (Table 1) without an intermediate calcination step using only 10% H2 in the reduction gas, whereas a calcination step and the presence of a H2 rich reduction gas gives rise to a drop in catalytic activity. The influence of different phases of titania supports, i.e., rutile and anatase on ruthenium and platinum catalysts, has been investigated in the hydrogenation of LA into GVL at 30°C or 70°C under 50 bar of H2 pressure in aqueous media (Ruppert et al., 2015). It has been surprisingly found that the influence of the type of support was not the same for ruthenium and platinum catalysts. The highest catalytic activity was exhibited by ruthenium on TiO2 support, consisting of 10% rutile and 90% anatase to obtain at 70°C quantitative conversion of LA and yield to GVL within 1 h, whereas with Ru on TiO2 support consisting of 100% anatase the conversion of LA was only 38 mol% and the yield to GVL of only 31 mol% under the same conditions. Microscopy studies showed that the ruthenium particles were exclusively located on the less rutile crystallites and could be understood due to a higher adhesion of ruthenium on rutile compared to anatase. In contrast, with platinum on TiO2 support consisting of 10% rutile and 90% anatase, the conversion of LA was 22 mol% and the yield to GVL of 18 mol%, whereas a higher conversion of LA of 54 mol% and a higher yield to GVL of 50 mol% were obtained by platinum catalysts on TiO2 support consisting of 100% of anatase. Novel Ru/TiO2 systems have been synthesized by photoassisted controlled modification of the TiO2 support by addition of calcium and applied as catalysts in the transfer hydrogenation of LA using formic acid as an internal hydrogen source in aqueous media (Wojciechowska et al., 2019). The Ca-modification of Ru/TiO2 catalysts enhanced the performance of both catalytic steps, the transfer hydrogenation of LA into GVL, and also the dehydrogenation of formic acid to yield the dihydrogen reactant. The Ca-modification caused both a decreased size of the anatase crystallite and the formation of a new phase of calcium titanate, resulting in smaller ruthenium metal particles stabilized on the support. The superior catalytic activity of Ca-modified Ru/TiO2 systems could be further understood probably due to the strong interaction between the smaller ruthenium particles with the Ca-modified TiO2 support. Gao et al. (2018) disclosed results on the stability of 2.5 wt.% Ru/ZrO2 catalysts applied in the transfer hydrogenation reaction of LA to GVL with a mixture of formic acid/formate at 150°C in aqueous media, and found that the incorporation of 0.1 wt.% SiO2 to the support enormously stabilized the system and the catalyst Ru/ZrO2-SiO2 retained its activity after recycling in three consecutive runs, whereas the activity of Ru/ZrO2 catalyst significantly decreased after recycling already in the first consecutive run. The catalytic properties of four different Ru/ZrO2 systems have been evaluated in the hydrogenation of LA to GVL in aqueous media; catalytic activity is strongly influenced by the pre-treatment method and the most active catalyst was obtained after impregnation of RuCl3·3H2O on pre-calcined zirconia support, followed by reduction with dihydrogen to give ruthenium metal particles of a size between 3.0 and 4.0 nm with an uniform dispersion (Filiz et al., 2017). Using this Ru/ZrO2 catalyst in the aqueous phase hydrogenation of LA at 170°C under 27 bar of H2 within 7 h at a molar ratio of LA/Ru = 2100, the catalytic activity obtained was 297 TOFs per hour with a conversion of LA of 99.0 mol% and a selectivity toward GVL of 99.9 mol% (Table 1). Stability studies of Ru/TiO2, Ru/ZrO2, and Ru/C catalytic systems have been reported on the hydrogenation reaction of LA to GVL at 150°C under 30 bar dihydrogen pressure in 1,4-dioxane and found that the Ru/TiO2 benchmark catalyst was deactivated just after the first recycling run, whereas the Ru/ZrO2 catalyst maintained its activity even after five recycling consecutive runs (Ftouni et al., 2016). The authors have clearly shown that the presence of the aqueous solvent has a promotional effect in the Ru/ZrO2-catalyzed hydrogenation reaction of LA to GVL. Thus, in a solvent mixture of 1 wt.% water with 99 wt.% 1,4-dioxane an increase in the GVL yield of 25 mol% was observed compared to anhydrous 1,4-dioxane solvent and in a mixture of 10 wt.% water with 90 wt.% 1,4-dioxane the catalytic activity further increased to obtain quantitative GVL yields. Ren et al. (2019) studied a cascade process for the direct conversion of carbohydrates fructose and glucose, and of storage or structural polysaccharides starch or cellulose toward GVL catalyzed by a system comprising the heteropoly acid-based ionic liquid 1-methyl-3-(3-sulfopropylimidazolium) silicotungstate and Ru/ZrO2 in aqueous media. Using this catalytic system at 180°C under argon for 3 h for the dehydration step and then under 40 bar dihydrogen pressure within 10 h for the hydrogenation step, the GVL yields obtained from fructose, glucose, starch, and cellulose were 63, 68, 60, and 60mol%, respectively. Recycling experiments with four consecutive runs have shown deactivation of Ru/ZrO2 catalyst component due to massive coke deposition formed probably from decomposition of humins and α-angelica lactone obtained as side products and intermediates at the reaction temperature of 180°C under acidic conditions. Raspolli Galletti et al. (2013) investigated the one pot approach for the conversion of giant reed (Arundo donax L.) directly to GVL by a combination process of acid catalyzed dehydration and catalytic hydrogenation reactions employing a bifunctional catalyst comprising of Ru/C and niobium phosphate or niobium oxide at a low temperature of 70°C and low dihydrogen pressure, i.e., 5 bar, to yield up to 16.6 mol% GVL, based on dry biomass with an essentially quantitative conversion of the LA intermediate in aqueous media. Xiao et al. (2016) synthesized few-layered graphene (FLG)-supported ruthenium nanoparticles and applied as them catalysts in the hydrogenation of LA in aqueous media to obtain at room temperature under 40 bar of H2 within 8 h a conversion of LA of 99.3 mol% and selectivity toward GVL of 97.7 mol% (Table 1). In five recycling experiments the 2.0% Ru/FLG catalyst retained its activity and exhibited four times higher activity compared with ruthenium catalysts on conventional support of activated carbon (Ru/C). Various ruthenium supported catalysts such as Ru/C, Ru/SiO2, and Ru/Al2O3 have been screened in the hydrogenation of LA into GVL under mild reaction conditions (25°C, 12 bar H2) in the absence of solvents with the Ru/C catalyst to show the highest catalytic activity, whereas Ru/SiO2 and Ru/Al2O3 exhibited significantly lower yields toward GVL (Al-Shaal et al., 2012). The commercial 5 wt.% Ru/C catalytic system has been further applied in the aqueous-phase hydrogenation of LA at 130°C under 12 bar of dihydrogen pressure within 2.7 h reaction time, and exhibited a moderate catalytic activity of 133 TOFs per hour (Table 1). It should be mentioned that conventional Ru/C catalysts suffer from enormous deactivation even at low temperatures and pressures, probably because of the suppressed mass transport of LA through the small-sized micropores resulting in a pore blocking of the carbon material. Primo et al. (2011) investigated the activity of 5 wt.% Ru/TiO2 and 0.64 wt.% Ru/TiO2 catalysts in the aqueous-phase hydrogenation of LA into GVL and found that the activity of 0.64 wt.% Ru/TiO2 is about two times higher compared with the activity of 5 wt.% Ru/TiO2 catalyst (Table 1), and could be explained due to the synergism between the small size of ruthenium nanoparticles and the activation of the carbonyl functionality of LA on the TiO2 support. Highly active ruthenium nanoparticles of a mean size of 1.4 nm supported on ultrathin TiO2 nanosheets with an unique two dimensional (2D) structure have been applied as catalysts in the hydrogenation of LA toward GVL (Table 1) to obtain impressively higher intrinsic catalytic activities (TOF = 19,045 h−1) under relative mild reaction conditions of 100°C and 40 bar dihydrogen pressure within 90 min reaction time, compared with those of conventional Ru/SiO2, Ru/MoS2, and Ru/C catalytic systems, which exhibited 7,089, 693, and 1,909 TOFs per hour, respectively, under identical conditions in aqueous media. It is relevant to point out that the calculation of TOF values in this work (Table 1) is based on surface ruthenium atoms and not on the bulk ruthenium loading (Gao et al., 2019). Recycling experiments have shown that the Ru/TiO2 ultrathin nanosheets catalyst could be reused in six consecutive hydrogenation runs without any deactivation. The apparent activation energy of the Ru/TiO2 ultrathin nanosheets catalyst in the aqueous-phase hydrogenation of LA was calculated and amounts to only 43.4 kJ/mol, which is much lower than that of Ru/C catalyst (Eapp= 87.66 kJ/mol), indicating a different nature of catalytically active sites. Employing high-resolution transmission electron microscopy (HRTEM), X-ray photoelectron spectroscopy (XPS), and temperature programmed reduction of hydrogen (H2-TPR) techniques has been revealed that in the Ru/TiO2 ultrathin nanosheets catalyst the ruthenium atoms are chemically bonded to oxygen atoms of TiO2 support to form Ru-O-Ti bonds, which cause a strong interfacial interaction. Whereas, in Ru/SiO2 and Ru/MoS2 catalysts the ruthenium atoms interact weakly with their supports, although a strong coordination has been observed between each ruthenium with their neighboring ruthenium atoms. The authors performed DFT calculations using a Ru/TiO2 modeled interface or a model of the most exposed Ru (0002) surface for the hydrogenation of LA, and have shown that the preferential mechanistic pathway is hydrogenation of the keto functionality with formation of , cyclization to GVL-OH and dehydroxylation to give GVL, independent of the structure of the surface. Interestingly, with the Ru/TiO2 interfacial structure, the rate determining step changes from hydrogenation to cyclization compared to Ru (002) surface, where the rate determining step is the hydrogenation one. In addition, to rationalize the results of exceptionally high catalytic activities exhibited by Ru/TiO2 ultrathin nanosheets catalysts where a strong interfacial interaction takes place due to the presence of Ru-O-Ti chemical bonds, we assume that such a system enormously facilitates both the heterolytic dihydrogen cleavage mechanism necessary for the easy hydrogenation of the keto functionality of LA into an alcohol group to yield the 4-hydroxyvaleric acid intermediate (vide supra, Unit 2.1 Hydrogenation of LA into GVL) and the dehydration step, as well to yield GVL. Van Nguyen et al. (2019) prepared via de novo synthesis a RuCl3@MIL-53-NH2 Metal-Organic Framework which was reduced at 500°C under H2/N2 gas flow to obtain the Ru@C-Al2O3 catalyst which consists of ruthenium nanoparticles confined in the C-Al2O3 honeycomb system. Ru@C-Al2O3 catalysts have been applied in the hydrogenation of LA under very mild conditions, i.e., at room temperature and atmospheric pressure of dihydrogen, to obtain a quantitative conversion of LA and a GVL yield of 99.9 mol% within 6 h of reaction time in the aqueous medium. Recycling experiments at 60°C showed that Ru@C-Al2O3 catalyst in aqueous media retained its activity in seven consecutive runs, indicating a stable catalytic system. The apparent activation energy of Ru@C-Al2O3 catalyst was calculated and amounts to 34.66 kJ/mol. Ru/γ-Al2O3 catalysts in the aqueous-phase hydrogenation of LA suffer from mediocre activities and stabilities mainly due to the non-uniform distribution of ruthenium on the support and because γ-Al2O3 is unstable in water (due to the existence of surface hydroxyl moieties) and tends after rehydration to form γ-AlOOH, i.e., boehmite. Tan et al. (2016) prepared functionalized γ-alumina supports such as NH2-γ-Al2O3 to obtain highly dispersed ruthenium nanoparticles (Figure 7) and applied them as catalytic systems in the hydrogenation reaction of LA selectively to GVL in aqueous media. Ru/NH2-γ-Al2O3 catalysts exhibited superior activity and stability compared with their Ru/γ-Al2O3 counterparts. For example, at 70°C with Ru/NH2-γ-Al2O3 catalysts, a TOF value of 3,355 per hour was obtained in the aqueous-phase hydrogenation of LA, whereas with Ru/γ-Al2O3 catalysts the activity was very lower, namely 432 TOFs per hour under the same reaction conditions. In ten recycling experiments the Ru/NH2-γ-Al2O3 catalyst retained its activity albeit the reaction temperature was high i.e., 130°C. Raspolli Galletti et al. (2012) investigated the hydrogenation of LA to GVL catalyzed by commercial 5 wt.% Ru/Al2O3 or 5 wt.% Ru/C catalysts combined with an acid co-catalyst such as the ion exchange resins of the type Amberlyst A70 and Amberlyst A15, niobium phosphate, and niobium oxide in aqueous media. The catalytic system 5 wt.% Ru/C-Amberlyst A70 exhibited the highest catalytic activity (TOF = 558 h−1) at 70°C under 5 bar dihydrogen pressure within 3 h, whereas with the 5 wt.% Ru/C catalyst in the absence of the acid co-catalyst the activity was rather low (TOF = 74 h−1) under the same reaction conditions. Piskun et al. (2016) have screened a series of 1 wt.% ruthenium catalysts supported on carbon, carbon nanotubes, Al2O3, SiO2, TiO2, ZrO2, Nb2O5, and zeolite β-12.5 at 90°C, 45 bar dihydrogen in aqueous media. Ru/β-12.5 catalysts exhibited by far higher catalytic activities even with a factor of 5 in comparison to the other ruthenium-supported catalysts, to obtain a conversion of LA of 94 mol% and selectivity to GVL, and 4-hydroxyvaleric acid of 66 and 34 mol%, respectively, within 2 h of reaction time in water. Zhang et al. (2017) applied highly stable Ru/ZSM-5 catalysts in the aqueous-phase hydrogenation of LA to GVL at a low reaction temperature of 70°C and performed recycling experiments with 10 consecutive runs without any loss of catalytic activity indicating no deactivation of the catalyst which is mainly caused by ruthenium aggregation, leaching and carbon deposition on the catalytically active sites. It has been found that a strong ruthenium-support interaction takes place at a higher tetrahedral–coordinated framework Al content, which minimizes the ruthenium aggregation and leaching under the acidic reaction conditions. Highly dispersed ruthenium nanoparticles consisting of Ru(0) to 59% and of RuO2 to 41%, with an average particle size of 2.3 nm on N-doped carbon nanospheres (Ru/NCS), were used as highly active catalysts (TOF= 9,858 h−1) in the hydrogenation of LA to GVL (Table 1) in aqueous media (Liu et al., 2019). The incorporation of N-dopant in the carbon matrix of the support plays a crucial role in the high dispersion of ruthenium nanoparticles because ruthenium nanoparticle catalysts in the absence of N-doping on carbon nanospheres (Ru/CS) were less active (TOF = 6,985 h−1) and displayed a clear sintering of ruthenium nanoparticles in the aqueous solvent (Table 1). Ndolomingo and Meijboom (2019) immobilized ruthenium, palladium, copper, and chromium nanoparticles with a mean particle size of 2–6 nm on mesoporous metal oxides such as TiO2, MnO2, and NiO, and applied them as catalysts in the LA hydrogenation reaction in aqueous media. At a reaction temperature of 150°C under 20 bar H2 within 5 h, the highest activity (TOF = 101 h−1) was obtained by Ru/TiO2 or Ru/MnO2 catalysts (Table 1) compared to Ru/NiO systems. Under the same conditions the reactivity exhibited Cu/TiO2 catalyst was rather low (TOF = 64 h−1). The catalytic activity of immobilized metal nanoparticles follows the order: Ru ≈ Pd > Cu > Cr and was revealed to be higher in water compared to the solvent-free system. Ruthenium nanoparticles with a mean size of 1.4 nm were embedded on dendrimers, followed by immobilization of the whole system on mesoporous TiO2 supports and then applied as catalysts in the LA hydrogenation reaction in the aqueous solvent in order to obtain LA conversions of 92 mol% with selectivities toward GVL and 2-MTHF of 98 and 2 mol%, respectively, and in organic solvents such as 1,4-dioxane where the conversion of LA was slightly higher (98 mol%) with selectivities to GVL and 2-MTHF of 99 and 1 mol%, respectively, at a reaction temperature of 150°C under 10 bar H2 pressure within 5 h of reaction duration (Nenamashi et al., 2018). Alginates which are polysaccharides in cell walls of macro algae were used to synthesize high surface area TiO2 and binary TiO2/ZrO2 supports for the immobilization of ruthenium nanoparticles applied as catalysts in the hydrogenation of LA toward GVL in aqueous media (Ruppert et al., 2019). The best catalytic performance was obtained with ruthenium nanoparticles possessing a bi-modal particle size distribution consisting of a mean particle size of 1.9 nm and an average size of 3.7 nm on bare TiO2 support, to achieve a conversion of LA of 79 mol% with a yield to GVL of 76 mol% under mild reaction conditions namely 30°C, and 50 bar of dihydrogen within 1 h of reaction time. Ruthenium nanoparticles with an average size of 2.8 nm diameter were encapsulated in sulfonated exchange resins such as DOWEX, and applied as bifunctional catalysts in the aqueous phase hydrogenation of LA to GVL under batch conditions to obtain an activity in the order of 102 TOF's per hour, with a conversion of LA of 98.3 mol% at 70°C, 10 bar H2 within 4 h of reaction time, and under continuous conditions at 70°C with dihydrogen pressures between 48 and 70 bar and contact times of 62–211s to observe conversions of LA between 89 and 100 mol% and excellent durability of the bifunctional Ru@ DOWEX catalyst (Moreno-Marrodan and Barbaro, 2014). The surfactant hexadecyl(2-hydroxyethyl)dimethylammonium dihydrogen phosphate (HHDMA) was used as a water-soluble ligand to modify ruthenium nanoparticles with an average diameter of 1.3 nm on TiSi2O6 support and applied as catalyst in the continuous hydrogenation of LA in water at 100–150°C, under pressures between atmospheric and up to 60 bar of H2 with contact times of 4–16s to obtain selectivities toward GVL higher than 95 mol%, with the selectivity to 1,4-PDO to be in the range of 5 mol% without any deactivation of the catalyst for a period of 15 h (Albani et al., 2017). A comparison to a conventional Ru/C catalyst with an average ruthenium particle diameter of 1.5 nm has shown that the Ru/HHDMA/TiSi2O6 catalytic system exhibited four times higher activity compared with the benchmark Ru/C catalyst which even suffers from severe deactivation due to formation of RuO2. Energy dispersive X-ray (EDX) maps of ruthenium and phosphorus in the Ru/HHDMA/TiSi2O6 system have shown that P is located in the areas where also Ru appears, and therefore it is highly probable that the HHDMA ligand is bounded to ruthenium nanoparticles with the phosphate moiety and to the TiSi2O6 carrier by the NCH2CH2OH functionality. The authors rationalized the results regarding the difference in stability of 0.24wt.% Ru/HHDMA/TiSi2O6 and 5wt% Ru/C catalysts by assuming that in the 0.24wt.% Ru/HHDMA/TiSi2O6 catalyst the phosphate groups of the ligands (ratio of HHDMA/RuNP ~ 250) shield the ruthenium nanoparticles and make difficult the oxygen approach, and also that the ligand-ruthenium interfacial acidity protects ruthenium nanoparticles from the oxidation reaction to form RuO2. Furthermore, DFT calculations revealed that these HHDMA ligand-ruthenium interfacial acidic properties in the highly polar aqueous solvent make it possible that the Ru/HHDMA/TiSi2O6-catalyzed reaction follows a mechanistic path with low energy barriers, resulting in a fourfold increase in catalytic activity.
Heterogeneous Water-Dispersible Catalytic Nanoparticles
Compared to the widely applied heterogeneous transition metal(0) catalytic nanoparticles (NPs) immobilized on the surface of various solid supports, the application of heterogeneous water-dispersible NPs catalysts constitutes a relatively novel but emerging approach in the field of aqueous-phase catalytic conversions of renewable biomass-derived platform chemicals. The aqueous solvent provides for a higher dispersion of the water-dispersible NPs able to exhibit great catalytic activities and impressive stabilities depending on the nature of the transition metal and especially on the choice of an appropriate stabilizer which plays a key role in keeping NPs with small particle size diameters avoiding aggregation under the reaction conditions, and thus keeping a large surface area due to the high ratio of surface metal atoms to bulk metal loading, which makes available a large number of catalytic active sites to convert the starting material. Consequently, a broad spectrum of various types of NPs stabilizers have been applied in different catalytic reactions which include, inter alia, surfactants, polymers, dendrimers, and also phosphines or nitrogen-containing ligands which are typically applied to modify homogeneous catalytic complexes (Roucoux et al., 2002; Yan et al., 2010; Dykeman et al., 2012; Yuan et al., 2012; Bouriazos et al., 2014; Bulut et al., 2015; Duan et al., 2015). Similar to the homogeneous water-soluble catalytic molecular complexes, the cumbersome separation and recycling of water-dispersible catalytic NPs in the aqueous solvent could be achieved by extraction after external addition of an organic solvent to create a biphasic system, followed by separation of the two phases.
In 2016, Patil and Bhanage studied the hydrogenation reaction of LA catalyzed by water-dispersible Ru(0) nanoparticles (RuNPs) formed in situ from RuCl3·3H2O precursor and polyethylene glycol (PEG) 400, used both as solvent and stabilizer at 110°C within 4 h reaction time in a solvent mixture PEG400/Water of a volume ratio 70/30. They reported a catalytic activity of TOF= 10 h−1 with a conversion of LA and selectivity to GVL of 99 mol%. At a higher temperature of 130°C the catalytic activity increased up to TOF= 40 h−1 with 99 mol% of both conversion of LA and selectivity toward GVL (Table 1). The formation of ruthenium nanoparticles was confirmed by TEM analysis and found nanoparticles of a very large size, i.e., 100–200 nm. Recycling experiments of the RuCl3·3H2O/PEG400/H2O system from the monophasic hydrogenation reaction mixture, followed by recovery of the catalyst by extraction and phase separation of a biphasic system created after addition of diethyl ether, have shown that the catalyst is stable without losing its activity and selectivity for six successive runs. Lower catalytic activities have been obtained in the absence of water using PEG400 alone or various organic solvents such as toluene, 1,4-dioxane, and ethanol. In, 2013 Ortiz-Cervantes and García applied water-dispersible RuNPs with a mean size of 2.4 nm as catalysts in the hydrogenation of LA prepared in situ from Ru3(CO)12 precursors in the absence of stabilizers. This exhibited a catalytic activity of TOF = 143 h−1 with a quantitative conversion of LA and selectivity to GVL at 130°C in aqueous media (Table 1). The 1,4-PDO product was obtained with a selectivity of 4 mol% at a higher temperature and pressure (150°C, 35 bar H2) and longer reaction time (24 h), with the selectivity of GVL to remain 96 mol% at a quantitative conversion of LA. Using RuNPs in organic solvents such as THF, the catalytic activities were lower with those obtained in water, and in the presence of alcohols such as methanol and propanol the main products were their corresponding levulinate esters. Tay et al. (2016) investigated hydrogenation reaction of LA to GVL catalyzed by water-dispersible RuNPs obtained in situ from both monodentate and bidentate p-cymene ruthenium(II) N-heterocyclic carbene (RuNHC) complexes as catalyst precursors in aqueous media. p-cymene RuNHC catalyst precursors with monodentate N-heterocyclic carbene ligands form RuNPS in both water and organic solvents and exhibited much higher catalytic activities in water (TOF = 361 h−1) compared with those obtained in organic solvents such as THF (TOF= 3 h−1), methanol, and isopropanol (Table 1). However, in organic solvents, p-cymene RuNHC catalyst precursors with bidentate N-heterocyclic carbene ligands form stable homogeneous complexes without any formation of RuNPs under hydrogenation reaction conditions and showed moderate catalytic activities. In 2017, Chen et al. synthesized water-dispersible RuNPs on cross-linked β-cyclodextrin with polyethylene glycol diglycidyl ether (PEG-CD) stabilizers and applied them as catalysts in the hydrogenation of LA to GVL in aqueous media. Ru@PEG-CD catalysts with an average particle size of 1.7 nm exhibited slightly higher activity compared to RuNPs with polyvinyl pyrrolidone (PVP), one of the most applied stabilizers, with a mean size of 2.3 nm at 80°C in water (Table 1). However, the Ru@PEG-CD catalytic system could be recycled in four consecutive runs without losing its activity, whereas the Ru@PVP catalyst already suffered from serious deactivation in the second recycling run. Protsenko et al. (2016, 2017, 2018) prepared RuO2 nanoparticles on hypercrosslinked polystyrene stabilizers containing amino groups (5% Ru/MN100) and on stabilizers without functionalities (5% Ru/MN270), and systematically studied the influence of operating reaction parameters on the catalytic activity of both systems applied in the hydrogenation reaction of LA selectively to GVL under mild conditions in aqueous media. It was found that 5 wt.% Ru/MN100 catalysts exhibited the highest activity (conversion of LA: 85 mol%), which was even superior to the activities obtained with commercial 5 wt.% Ru/C catalysts (conversion of LA: 64 mol%) under the same conditions (90°C, 20 bar H2, 50 min) in aqueous media, albeit the surface of Ru/C catalyst consists to 12.5% of Ru(0) and to 87.5% of RuO2 with a different degree of hydration, whereas the composition of the surface of Ru/MN100 catalyst consists to 100% of RuO2 with different degrees of hydration. This is remarkable because Ru(0) catalysts usually exhibit much higher activities compared to RuO2 in the LA hydrogenation reaction in aqueous media. Kubo et al. (2016) prepared water-dispersible boronate nanoparticles coated by polyethyleneimine (BNP) with an average diameter of 121 nm and used as support materials for ruthenium, palladium, and platinum nanoparticles with mean diameters <1 nm to catalyze the hydrogenation reaction of LA in aqueous media under mild conditions (100°C, 8 bar H2, 4 h). The highest conversion of LA (94.6 mol%) was obtained with Ru/BNP catalyst, which was stable in four recycling runs, whereas Pt/BNP and Pd/BNP catalysts exhibited lower activities to give conversions of LA of 46.9 and 24.4 mol%, respectively, with essentially quantitative selectivity toward GVL with all three catalysts. The authors further applied Ru/BNP catalysts in the LA hydrogenation reaction in organic media such as ethanol and toluene with however much lower activities in both solvents in comparison to water. RuNPs with an average size of 3.0 nm randomly distributed on the surface of a cross-linked sulfonated polyethersulfone (SPES) support were used as catalysts for the hydrogenation of LA to afford GVL in quantitative selectivity with a conversion of LA up to 87.9 mol% at 70°C under 30 bar H2 within 2 h in aqueous medium (Yao et al., 2014). The swelling properties of SPES support in water facilitated both the adsorption of LA to ruthenium catalytic active sites for its hydrogenation to yield 4-hydroxyvaleric acid intermediate, and to access easily the –SO3H acid sites for its dehydration reaction to give by intramolecular esterification the GVL product.
Homogeneous Water-Soluble Catalytic Complexes
The use of homogeneous water-soluble transition metal catalytic complexes in aqueous media is of great interest, particularly in the aqueous/organic biphasic mode, owing to the possibility for the heterogenization of homogeneous catalysis, which combines many advantages: (i) high activities and selectivities even under mild conditions by fine tuning of the coordination sphere of the transition metal employing a wide spectrum of water-soluble ligands in the aqueous solvent (Papadogianakis and Sheldon, 1996), (ii) easy and quantitative recovery of the catalyst in active form from organic reaction products by simple phase separation of the biphasic system and facile catalyst recycling. Consequently, numerous steps in classical homogeneous industrial processes are rendered superfluous and process engineering is enormously simplified, resulting in substantial energy savings and lower emissions (Papadogianakis and Sheldon, 1997) and (iii) new types of catalytic reactivities have been observed in the aqueous medium. The catalytic activities were much higher in water compared to organic solvents in various and different types of catalytic reactions such as hydrogenation (Moustani et al., 2018), hydrocarboxylation (Papadogianakis et al., 1997), and hydroformylation reactions (Fremy et al., 1995), which contrasts with the general perception that aqueous-phase catalysis normally exhibits lower rates compared to analogous catalytic reactions in organic solvents. Water-soluble rhodium catalytic complexes with trisulfonated triphenylphosphine ligands (TPPTS, Figure 8) have found important industrial applications such as in the Ruhrchemie/Rhône-Poulenc process for the hydroformylation of the lower olefins propylene and 1-butene, which is rich in the raffinate II mixture [raffinate II consists of 1-butene, 2-butenes (cis/trans) and butanes (n-/iso-) obtained from C4-stream of naphtha crackers] and in the Rhône-Poulenc process for the synthesis of vitamin E and A intermediates in aqueous/organic two-phase systems. Moreover, water-soluble palladium and rhodium complexes with monosulfonated triphenylphosphine ligands (TPPMS, Figure 8) have been used as catalysts in the aqueous/organic biphasic system in the Kuraray process, which is a four step process for the hydrodimerization of 1,3-butadiene to produce 1,9-non-anediol and 1-octanol as well (Papadogianakis et al., 1997). The hydrogenation reaction of LA in the aqueous solvent catalyzed by water-soluble ruthenium complexes proceeds in a monophasic system mode because LA is a polar and hydrophilic starting material. Therefore, the separation of water-soluble ruthenium catalytic complexes from aqueous one-phase LA hydrogenation reaction mixture proceeds with biphasic recovery of the catalyst in active form from organic reaction products by extraction and simple phase separation of an aqueous/organic two-phase system created after external addition of an organic solvent such as ethyl acetate or diethyl ether, which finally results in a facile recycling of the water-soluble catalyst.
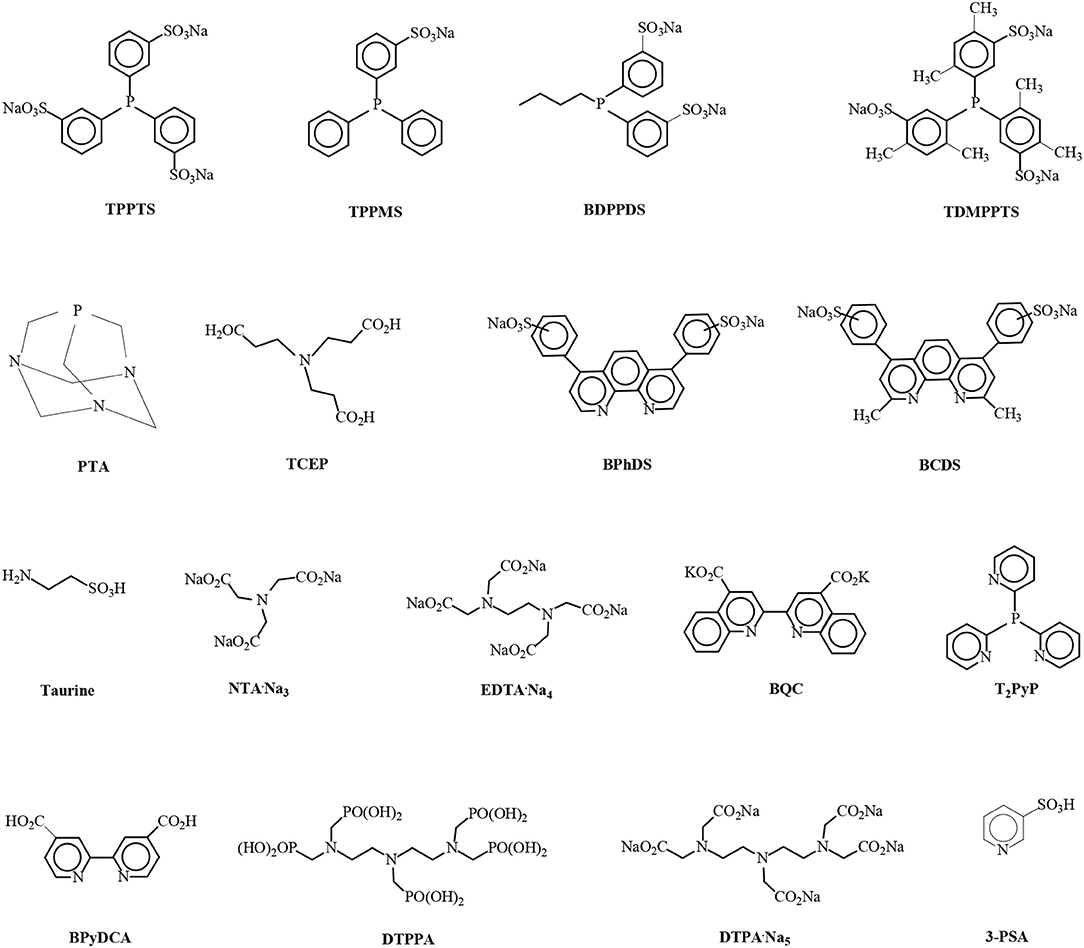
Figure 8. Structures of the water-soluble ligands triphenylphosphinetrisulfonic acid trisodium salt (TPPTS), triphenylphosphinemonosulfonic acid monosodium salt (TPPMS), n-butyldiphenylphosphinedisulfonic acid disodium salt (BDPPDS), tris(2,4-dimethylphenyl)phosphinetrisulfonic acid trisodium salt (TDMPPTS), 1,3,5-triaza-7-phosphaadamantane (PTA), tris(2-carboxyethyl)phosphine (TCEP), bathophenanthrolinedisulfonic acid disodium salt (BPhDS), bathocuproinedisulfonic acid disodium salt (BCDS), 2-aminoethanesulfonic acid (Taurine), nitrilotriacetic acid trisodium salt (NTA·Na3), ethylenediaminetetraacetic acid tetrasodium salt (EDTA·Na4), 2,2′-biquinoline-4,4′dicarboxylic acid dipotassium salt (BQC), tris(2-pyridyl)phosphine (T2PyP), N,N′-2,2′-bipyridine-4,4′-dicarboxylic acid (BPyDCA), diethylenetriaminepentakis(methylphosphonic acid) (DTPPA), diethylenetriaminepentaacetic acid pentasodium salt (DTPA·Na5) and 3-pyridinesulfonic acid (3-PSA).
The advent of aqueous-phase LA hydrogenation reactions catalyzed by homogeneous water-soluble ruthenium complexes could be traced back to 1977 with the pioneering work of F. Joó on hydrogenate keto carboxylic acids, such as pyruvic acid and LA by water-soluble Ru/TPPMS catalytic complexes in aqueous monophasic systems (Joó et al., 1977). Mehdi et al. (2008) reported the aqueous monophasic hydrogenation of LA catalyzed by water-soluble Ru(acac)3/TPPTS complexes at 140°C under 69 bar hydrogen pressure at molar ratios of LA/Ru = 600 and TPPTS/Ru = 10 within 12 h to yield 95% GVL, which was isolated after extraction by means of a biphasic system created by external addition of ethyl acetate (Mika et al., 2015). In Chalid et al. (2011) studied the biphasic hydrogenation of LA to GVL catalyzed by water-soluble RuCl3·3H2O/TPPTS systems at molar ratios of LA/Ru= 100 and TPPTS/Ru = 1, a pH value of 7, a reaction temperature of 90°C under 45 bar hydrogen pressure within 1 h to observe a conversion of 82% of LA in a dichloromethane/water (volume ratio = 100/25) two-phase system which could allow recovery and recycling of the catalyst. In a recycling experiment, however, the RuCl3·3H2O/TPPTS catalyst was partially deactivated because in the first run the conversion of LA was 81% and in the followed catalyst recycling experiment only 55% of LA was converted. Tukacs et al. (2012) described the hydrogenation of LA catalyzed by Ru(acac)3 modified with several water-soluble sulfonated alkylphenylphosphines under solvent-free conditions and found that the Ru(acac)3 precursor modified with the disulfonated diphenylbutylphosphine ligand (BDPPDS, Figure 8) exhibited the highest catalytic activity of 3,540 TOFs per hour under 100 bar hydrogen pressure at 140°C and molar ratios of LA/Ru = 6,370 and P/Ru = 10 within 1.8 h reaction time to yield 99.9% GVL. Recycling experiments of the Ru/BDPPDS catalyst were carried out using the technique of separation by distillation of volatile compounds under reduced pressure from homogeneous catalyst and addition of a new portion of LA without a significant decrease in catalytic activity for six consecutive runs. Delhomme et al. (2013) disclosed the hydrogenation reaction of LA in aqueous monophasic systems using RuCl3·3H2O and Ru(acac)3 catalyst precursors modified with water-soluble phosphine ligands such as TPPTS, TPPMS, trisulfonated tris(2,4-dimethylphenyl)phosphine (TDMPPTS), 1,3,5-triaza-7-phosphaadamantane (PTA) and tris(2-carboxyethyl)phosphine (TCEP) (Figure 8) at 140°C under 50 bar of dihydrogen pressure, molar ratios of LA/Ru = 100–600 and of P/Ru = 3.25–10 within 5 h reaction time. The highest activity of TOF = 210 h−1 was exhibited by the RuCl3·3H2O/TPPTS catalytic system, whereas with the Ru(acac)3/TPPTS system the activity was lower to achieve 202 TOF's per hour, and the conversion of LA and selectivity to GVL were 99 and 97 mol%, respectively. Moustani et al. (2018) investigated the hydrogenation reaction of LA using RuCl3·3H2O, Ru(NO)(OAc)3, Ru(NO)(NO3)3, Ru(acac)3, [Ru(NO)]2(SO4)3, and RuO2·H2O catalyst precursors modified with water-soluble phosphine and especially with nitrogen-containinig ligands such as TPPTS, PTA, bathophenanthrolinedisulfonic acid disodium salt (BPhDS), bathocuproinedisulfonic acid disodium salt (BCDS), 2-aminoethanesulfonic acid (taurine), nitrilotriacetic acid trisodium salt (NTA·Na3), ethylenediaminetetraacetic acid tetrasodium salt (EDTA·Na4), 2,2'-biquinoline-4,4'dicarboxylic acid dipotassium salt (BQC), tris(2-pyridyl)phosphine (T2PyP), N,N'-2,2'-bipyridine-4,4'-dicarboxylic acid (BPyDCA), diethylenetriaminepentakis(methylphosphonic acid) (DTPPA), diethylenetriaminepentaacetic acid pentasodium salt (DTPA·Na5) and 3-pyridinesulfonic acid (3-PSA) (Figure 8) in the aqueous monophasic system. The highest activity of TOF = 3,000 h−1 was obtained with RuCl3·3H2O/BPhDS catalysts at 140°C, 80 bar H2 pressure within 1 h and molar ratios of LA/Ru = 3,000 and BPhDS/Ru=1 with addition of 5 ml of aqueous solvent by a ruthenium concentration of 75 ppm and pH value of 2.43 where the conversion of LA was quantitative with essentially quantitative selectivity to GVL of 99.9 mol% and formation of only 0.1 mol % of the 1,4-PDO byproduct. The apparent activation energy of the Ru/BPhDS catalytic system amounts a relative low value of 53.3 kJ/mol, which is remarkable when one considers that this catalyst reduces a less reactive keto functionality into an alcohol group. Recycling experiments of the Ru/BPhDS catalyst from the aqueous monophasic LA hydrogenation reaction mixture, followed by biphasic recovery of the catalyst in active form from organic reaction products by extraction and simple phase separation of an aqueous/organic two-phase system created after external addition of diethyl ether, has shown that the catalyst is stable without loss of activity and selectivity in a consecutive run. The presence of organic solvents gives rise to a dramatic drop in catalytic activities which was obvious during the recycling experiments of the Ru/BPhDS catalytic system. After the hydrogenation run it is crucial to add small amounts of the organic solvent, i.e., only up to 10 ml of diethyl ether to the aqueous monophasic reaction mixture (10 ml), to form a biphasic system for the recovery and recycling of the Ru/BPhDS catalyst. In the case that higher quantities of diethyl ether were added for the extraction, the catalytic activity exponentially decreased in the consecutive LA hydrogenation run due to the minor amount of diethyl ether which was inevitably dissolved in the aqueous catalyst solution.
One-Pot Hydrogenation of LA Into VA, 1,4-PDO, 2-MTHF, 2-Pentanol and 2-Butanol
The catalytic hydrogenation of LA beyond GVL to yield VA, which in the presence of alcohols under acidic conditions forms alkyl valerate biofuels and 1,4-PDO followed by dehydration to obtain 2-MTHF (Figure 9), is a difficult reaction which usually takes place in the gas-phase often in the presence of platinum-based heterogeneous catalysts under severe conditions i.e., high temperatures and/or high dihydrogen pressures. For example, Kon et al. (2014) described how the one-pot hydrogenation of LA catalyzed by Pt/H-ZSM-5 at 200°C under solvent-free conditions yielded up to 99 mol% VA. Whereas, with methanol up to 87 mol% methyl valerate, Lange et al. (2010) disclosed the one-step conversion of GVL into valeric esters over Pt/TiO2 catalysts with a selectivity of 20–50 mol% pentyl valerate at higher temperatures of 275–300°C. Ruthenium-catalyzed one-pot hydrogenation reactions of LA into VA and 1,4-PDO are rare probably because ruthenium-based catalysts in the gas-phase suffer from drawbacks such as poor hydrogenation activity compared to analogous platinum and other transition metals-based counterparts applied in the gas-phase. The first example of one-pot hydrogenation reaction of LA into VA was reported by Luo et al. (2013), and deals with the application of the Ru/H-ZSM catalyst which contains strongly acidic sites able to catalyze the most difficult step in that sequence which is considered to be the rate-limiting step, namely the ring-opening reaction of GVL to yield at 200°C under 40 bar of dihydrogen pressure up to 45.8 mol% VA together with alkyl valerate co-products. The Ru/H-ZSM catalyst suffers from gradual deactivation, which could be attributed to dealumination resulting in a loss of catalytically active acid sites. At the higher temperature of 200°C the acid-catalyzed dehydration reaction of LA takes place with formation of α-angelica lactone, which polymerizes on the acidic sites to yield coke that after deposition also deactivates the Ru/H-ZSM catalyst. Bababrik et al. (2017) disclosed DFT calculations to present in detail the reaction mechanism of GVL conversion on Ru(0001) surfaces. The authors have shown that the GVL ring-opening step through breaking the C(1)-O(2) bond (Figure 10) proceeds rather easily with an activation energy of 25 kJ/mol and exothermicity (-31 kJ/mol), and the rate-limiting step is the hydrogenation step of the formed acyl intermediate CH3CH(O*)-(CH2)2-C*-O* with an activation barrier of 146 kJ/mol and endothermicity (+74 kJ/mol) to yield 1,4-PDO. In contrast, the alternative pathway of GVL ring-opening through breaking the C(4)-O(2) bond (Figure 10) proceeds with more difficulty with a much higher energy barrier of 122 kJ/mol, which leads to VA (Rozenblit et al., 2016). Aqueous-phase hydrogenations of GVL catalyzed by 5 wt.% Ru/C were carried out by Rozenblit et al. (2016) at 200°C under 69 bar dihydrogen pressure to obtain a conversion of GVL of 10.3 mol% and a spectrum of products with a yield of 4.95 mol% 2-butanol, formed after C-C bond cleavage in a decarbonylation step of the surface acyl intermediate CH3CH(O*)-(CH2)2-C*-O* obtained after GVL ring-opening, and further 4.63 mol% 1,4-PDO after hydrogenation of the acyl intermediate, 0.64 mol% 2-MTHF after dehydration of 1,4-PDO and cyclization, and 0.08 mol% 2-pentanol obtained after deoxygenation and further hydrogenation of the surface CH3CH(O*)-(CH2)2-C*-O* acyl intermediate. Cui et al. (2018) studied the continuous one-pot aqueous-phase hydrogenation reaction of LA catalyzed by molybdenum-modified Ru/C catalysts to obtain almost quantitative conversion of LA and selectivities of 96.7 mol% 1,4-PDO, 0.2 mol% 2-MTHF, 0.5 mol% GVL, 0.6 mol% 2-butanol and 2.0 mol% 2-pentanol using an atomic ratio of Mo/Ru= 0.25 under mild conditions at 70° C and 40 bar dihydrogen pressure. In situ FTIR investigations revealed that RuNPs are responsible for the activation and dissociation of the dihydrogen reactant and on Mo catalytically active species the absorption and activation of the keto functionality of LA starting material takes place. The Ru-MoOx/C catalyst remained stable for a period of 200 h continuous one-pot LA hydrogenation reaction toward 1,4-PDO without any deactivation. Lv et al. (2018) reported the one-pot aqueous-phase hydrogenation of LA catalyzed by nanoporous ruthenium-based catalysts to obtain at 100°C under 60 bar H2 within 6 h reaction time a yield of 74.6 mol% 1,4-PDO, 1.9 mol% 2-MTHF, 4.6 mol% GVL, 10.4 mol% 2-butanol and 5.0 mol% 2-pentanol. At a higher temperature of 140°C the yields of both 2-butanol and 2-pentanol were increased to 63.6 and 15.2 mol%, respectively, with, however, a low yield of 1,4-PDO of 0.9 mol%. Mizugaki et al. (2017) and Mizugaki and Kaneda (2019) investigated the one-pot hydrogenative C-C bond cleavage reaction of LA catalyzed by RuNPs with an average particle diameter of 3 nm supported on CeO2 to achieve almost an quantitative conversion of LA and a yield of 85 mol% 2-butanol and of 5 mol% 2-pentanol at 150°C under 30 bar dihydrogen pressure within 12 h reaction time in aqueous media. The presence of the aqueous solvent is indispensable in the Ru/CeO2-catalyzed hydrogenative C-C cleavage reaction of LA toward 2-butanol, because in organic solvents such as 2-propanol, THF or dimethoxyethane the yield to 2-butanol was only up to 5 mol% and the major products were GVL of 70 mol% and 1,2-PDO of 23 mol%. Licursi et al. (2018) studied a cascade process for the direct conversion of LA or GVL into 2-MTHF or 2-butanol and 2-pentanol, employing commercially available catalysts in the aqueous solvent. The authors used for the first time a catalyst combination of Ru/C along with Re/C, together with niobium phosphate to obtain selectivities toward 2-MTHF of 28 mol% or 65 mol % from LA or GVL substrates, respectively. In sharp contrast employing a catalyst combination comprising of Ru/C and HY zeolite at 200°C under 30 bar dihydrogen pressure, the reaction takes another course and yields a mixture of 2-butanol with 2-pentanol of 88.8 mol% and 100 mol% from LA and GVL starting materials, respectively, in aqueous media.
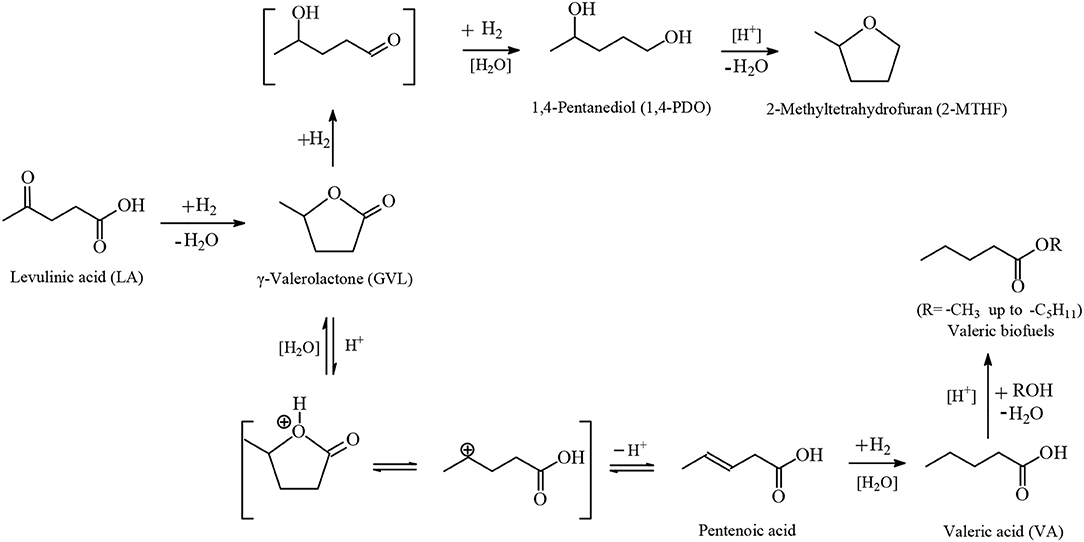
Figure 9. Hydrogenation of LA beyond GVL to yield VA for the production of valeric biofuels and 1,4-PDO followed by dehydration to form 2-MTHF.
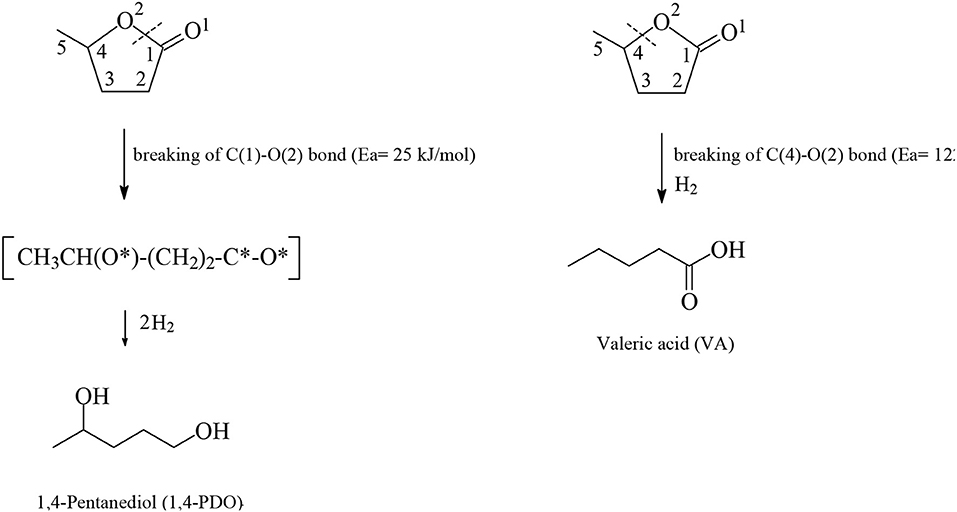
Figure 10. Ring-opening pathways of GVL through breaking the C(1)-(O)2 bond which after hydrogenation eventually leads into 1,4-PDO and through C(4)-(O)2 bond breaking into VA.
Summary and Outlook
The catalytic hydrogenation route for the valorization of LA has received considerable interest recent years because of the wide range of potential applications of LA's hydrogenation products which include, inter alia, advanced biofuels, fine chemicals, solvents and additives to gasoline or to food. In the last decade the hydrogenation reactions of LA into GVL, which is a key intermediate compound, and beyond GVL to yield VA, 1,4-PDO, 2-MTHF, 2-pentanol, and 2-butanol have gained a lot of attention and various transition metal catalytic systems have been developed in the absence and presence of organic or aqueous solvents. Remarkable progress has been made, however, in the application of ruthenium-based catalytic systems which have been used extensively due to the inherent ability of ruthenium under mild conditions to hydrogenate the carbonyl functionality of levulinic acid selectively into an alcohol group to form 4-hydroxyvaleric acid intermediate, which spontaneously after dehydration and cyclization yields GVL. This critical review has summarized and discussed the progress made in the last decade in the field of aqueous-phase ruthenium-catalyzed hydrogenation reactions of LA employing heterogeneous catalysts on solid supports and heterogeneous water-dispersible catalytic nanoparticles or homogeneous water-soluble catalytic complexes with biphasic catalyst separation. The significance of the aqueous solvent to carry out catalytic hydrogenation reactions of LA has been highlighted because the presence of water combines many environmental and economic benefits. Therefore, this review constitutes a guidance for the development of novel efficient ruthenium-based catalytic systems which would be more active and especially capable for the one-pot hydrogenation of LA toward VA, 1,4-PDO, 2-MTHF, 2-pentanol and 2-butanol. At the same time it offers guidance on more stable and recyclable systems suitable as catalysts for industrial-scale LA hydrogenation reaction in green and sustainable aqueous solvents to be integrated into biorefineries of the future.
Author Contributions
GP has written this review. All other authors have equally contributed and approved it for publication.
Funding
Financial support of this work by the Postgraduate Studies Programme on Catalysis and its Applications in the Industry of Greek Ministry of Education and by the Special Account for Research Grants of the Research Committee of the National and Kapodistrian University of Athens under contract 70/4/7568 are gratefully acknowledged.
Conflict of Interest
The authors declare that the research was conducted in the absence of any commercial or financial relationships that could be construed as a potential conflict of interest.
References
Albani, D., Li, Q., Vilé, G., Mitchell, S., Almora-Barrios, N., Witte, P. T., et al. (2017). Interfacial acidity in ligand-modified ruthenium nanoparticles boosts thehydrogenation of levulinic acid to gamma-valerolactone. Green Chem. 19, 2361–2370. doi: 10.1039/C6GC02586B
Al-Shaal, M. G., Wright, W. R. H., and Palkovits, R. (2012). Exploring the ruthenium catalyzedsynthesis of γ-valerolactone in alcohols and utilisation of mild solvent-free reactionconditions. Green Chem. 14, 1260–1263. doi: 10.1039/c2gc16631c
Aycock, D. F. (2007). Solvent applications of 2-methyltetrahydrofuran in organometallic and biphasic reactions. Org. Process Res. Rev. 11, 156–159. doi: 10.1021/op060155c
Bababrik, R. M., Wang, B., and Resasco, D. (2017). Reaction mechanism for the conversion of γ-valerolactone (GVL) over a Ru catalyst: a first-principles study. Ind. Eng. Chem. Res. 56, 3217–3222. doi: 10.1021/acs.iecr.7b00196
Badgujar, K. C., Badgujar, V. C., and Bhanage, B. M. (2020). A review on catalytic synthesis ofenergy rich fuel additive levulinate compounds from biomass derived levulinic acid. Fuel Process. Technol. 197:106213. doi: 10.1016/j.fuproc.2019.106213
Bond, J. Q., Alonso, D. M., Wang, D., West, R. M., and Dumesic, J. A. (2010). Intergrated catalyticconversion of γ-valerolactone to liquid alkenes for transportation fuels. Science 327, 1110–1114. doi: 10.1126/science.1184362
Bond, J. Q., Upadhye, A. A., Olcay, H., Tompsett, G. A., Jae, J., Xing, R., et al. (2014). Production of renewable jet fuel range alkanes and commodity chemicals from integrated catalytic processing of biomass. Energy Environ. Sci. 7, 1500–1523. doi: 10.1039/C3EE43846E
Bouriazos, A., Sotiriou, S., Stathis, P., and Papadogianakis, G. (2014). Superior aqueous-phasecatalytic hydrogenation activity of palladium modified with nitrogen-containing ligands compared with the TPPTS benchmark modifier in micellar nanoreactors. Appl. Catal. B: Environ. 150–151, 345–353. doi: 10.1016/j.apcatb.2013.12.032
Bozell, J. J., and Petersen, G. R. (2010). Technology development for the production of biobased products from biorefinery carbohydrates - the US Department of Energy's “Top 10” revisited. Green Chem. 12, 539–554. doi: 10.1039/b922014c
Bulut, S., Fei, Z., Siankevich, S., Zhang, J., Yan, N., and Dyson, P. J. (2015). Aqueous-phase hydrogenation of alkenes and arenes: The growing role of nanoscale catalysts. Catal. Today 247, 96–103. doi: 10.1016/j.cattod.2014.09.002
Byrne, F., Forier, B., Bossaert, G., Hoebers, C., Farmer, T. J., Clark, J. J., et al. (2017). 2,2,5,5-Tetramethyltetrahydrofuran (TMTHF): a non-polar, non-peroxide forming ether replacement for hazardous hydrocarbon solvents. Green Chem. 19, 3671–3678. doi: 10.1039/C7GC01392B
Chalid, M., Broekhuis, A. A., and Heeres, H. J. (2011). Experimental and kinetic modeling studies on the biphasic hydrogenation of levulinic acid to γ-valerolactone using a homogeneous water-soluble Ru-(TPPTS) catalyst. J. Mol. Catal. A: Chem. 341, 14–21. doi: 10.1016/j.molcata.2011.04.004
Chen, M., Dong, Q., Ni, W., Zhao, X., Gu, Q., Tang, G., et al. (2017). Cyclodextrin-based polymer-assisted Ru nanoparticles for the aqueous hydrogenation of biomass-deriver platform molecules. ChemistrySelect 2, 10537–10545. doi: 10.1002/slct.201702229
Climent, M. A., Corma, A., and Iborra, S. (2014). Conversion of biomass platform molecules into fuel additives and liquid hydrocarbon fuels. Green Chem. 16, 516–547. doi: 10.1039/c3gc41492b
Cui, J., Tan, J., Zhu, Y., and Cheng, F. (2018). Aqueous hydrogenation of levulinic Acid to 1,4-pentanediol over Mo-modified Ru/activated carbon catalyst. ChemSusChem. 11, 1316–1320. doi: 10.1002/cssc.201800038
Delhomme, C., Schaper, L.-A., Zhang-Preße, M., Raudaschl-Sieber, G., Weuster-Botz, D., and Kühn, F. E. (2013). Catalytic hydrogenation of levulinic acid in aqueous phase. J. Organomet. Chem. 724, 297–299. doi: 10.1016/j.jorganchem.2012.10.030
Duan, H., Wang, D., and Li, Y. (2015). Green chemistry for nanoparticle synthesis. Chem. Soc. Rev. 44, 5778–5792. doi: 10.1039/C4CS00363B
Dutta, S., Yu, I. K. M., Tsang, D. C. W., Ng, Y. H., Ok, Y. S., Sherwood, J., et al. (2019). Green synthesis of gamma-valerolactone (GVL) through hydrogenation of biomass-derived levulinic acid using non-noble metal catalysts: a critical review. Chem. Eng. J. 372, 992–1006. doi: 10.1016/j.cej.2019.04.199
Dykeman, R. R., Yuan, Y., Yan, N., Asakura, H., Teramura, K., Tanaka, T., et al. (2012). Rational design of a molecular nanocatalyst-stabilizer that enhances both catalytic activity and nanoparticle stability. ChemCatChem 4, 1907–1910. doi: 10.1002/cctc.201200552
Fábos, V., Koczó, G., Mehdi, H., Boda, L., and Horváth, I. T. (2009). Bio-oxygenates and the peroxide number: a safety issue alert. Energy Environ. Sci. 2, 767–769. doi: 10.1039/b900229b
Filiz, B. C., Gnanakumar, E. S., Martínez-Arias, A., Gengler, R., Rudolf, P., Rothenberg, G., et al. (2017). Highly selective hydrogenation of levulinic acid to γ-valerolactone over Ru/ZrO2 catalysts. Catal. Lett. 147, 1744–1753. doi: 10.1007/s10562-017-2049-x
Fremy, G., Monflier, E., Carpentier, J.-F., Castanet, Y., and Mortreux, A. (1995). Enhancement of catalytic activity for hydroformylation of methyl acrylate by using biphasic and “supported aqueous phase” systems. Angew. Chem. Int. Ed Engl. 34, 1474–1476. doi: 10.1002/anie.199514741
Ftouni, J., Murillo, A. M., Goryachev, A. E., Hofmann, J. P., Hensen, E. J. M., Lu, L., et al. (2016). ZrO2 is preferred over TiO2 as support for the Ru-catalyzed hydrogenation of levulinic acid to γ-valerolactone. ACS Catal. 6, 5462–5472. doi: 10.1021/acscatal.6b00730
Gao, X., Zhu, S., Dong, M., Wang, J., and Fan, W. (2019). Ru nanoparticles deposited on ultrathin TiO2 nanosheets as highly active catalyst for levulinic acid hydrogenation to γ-valerolactone. Appl. Catal. B: Environ. 259:118076. doi: 10.1016/j.apcatb.2019.118076
Gao, Y., Zhang, H., Han, A., Wang, J., Tan, H.-R., Tok, E.-S., et al. (2018). Ru/ZrO2 catalysts for transfer hydrogenation of levulinic acid with formic acid/formate mixtures: importance of support stability. Chem. Select 3, 1343–1351. doi: 10.1002/slct.201702152
Gundekari, S., and Srinivasan, K. (2019). Hydrous ruthenium oxide: a new generation remarkable catalyst precursor for energy efficient and sustainable production of γ-valerolactone from levulinic acid in aqueous medium. Appl. Catal. A Gen. 569, 117–125. doi: 10.1016/j.apcata.2018.10.018
Guo, Y., Li, Y., Chen, J., and Chen, L. (2016). Hydrogenation of levulinic acid into γ-valerolactone over ruthenium catalysts supported on Metal-Organic Frameworks in aqueous medium. Catal Lett. 146, 2041–2052. doi: 10.1007/s10562-016-1819-1
Home page of GFBiochemicals company. (2019). Available online at: http://www.gfbiochemicals.com/company/ (accessed January 20, 2020)
Huber, G. W., Iborra, S., and Corma, A. (2006). Synthesis of transportation fuels from biomass: chemistry, catalysts, and engineering. Chem. Rev. 106, 4044–4098. doi: 10.1021/cr068360d
Joó, F., Tóth, Z., and Beck, M. T. (1977). Homogeneous hydrogenations in aqueous solutions catalyzed by transition metal phosphine complexes. Inorg. Chim. Acta 25, L61–L62 doi: 10.1016/S0020-1693(00)95645-7
Kon, K., Onodera, W., and Shimizu, K.-I. (2014). Selective hydrogenation of levulinic acid to valeric acid and valeric biofuels by a Pt/HMFI catalyst. Catal. Sci. Technol. 4, 3227–3234. doi: 10.1039/C4CY00504J
Kubas, G. J. (2005). Catalytic processes involving dihydrogen complexes and other sigma-bond Complexes. Catal. Lett. 104, 79–101. doi: 10.1007/s10562-005-7440-3
Kubo, Y., Kakizaki, D., Kogo, M., and Magatani, Y. (2016). Water-dispersible boronate nanoparticles as support materials for noble metals in the hydrogenation of levulinic acid to γ-valerolactone. Supramol. Chem. 28, 91–97. doi: 10.1080/10610278.2015.1086764
Lange, J. P., Price, R., Ayoub, P. M., Louis, J., Petrus, L., Clarke, L., et al. (2010). Valeric biofuels: a platform of cellulosic transportation fuels. Angew. Chem. Int. Ed. 49, 4479–4483. doi: 10.1002/anie.201000655
Leitner, W., Klankermayer, J., Pischinger, S., Pitsch, H., and Kohse-Höinghaus, K. (2017). Advanced biofuels and beyond: chemistry solutions for propulsion and production. Angew. Chem. Int. Ed. 56, 5412–5452. doi: 10.1002/anie.201607257
Li, G., Liu, W., Ye, C, Li, X., and Si, C. L., (2018). Chemocatalytic conversion of cellulose into key platform chemicals. Int. J. Polym. Sci. 2018:21. doi: 10.1155/2018/4723573
Licursi, D., Antonetti, C., Fulignati, S., Giannoni, N., and Raspolli Galletti, A. M. (2018). Cascade strategy for the tunable catalytic valorization of levulinic acid and γ-valerolactone to 2-methyltetrahydrofuran and alcohols. Catalysts 8:277. doi: 10.3390/catal8070277
Liu, X., Lan, G., Boyjoo, Y., Qian, L., Gu, S., Price, C. A. H., et al. (2019). N-doped carbon spheres impregnated with highly monodispersed ruthenium nanoparticles as a hydrogenation catalyst. Chem. Eng. J. 374, 895–903. doi: 10.1016/j.cej.2019.05.213
Luo, W., Deka, U., Beale, A. M., Van Eck, E. R. H., Bruijnincx, P. C. A., and Weckhuysen, B. M. (2013). Ruthenium-catalyzed hydrogenation of levulinic acid: Influence of the support and solvent on catalyst selectivity and stability. J. Catal. 301, 175–186. doi: 10.1016/j.jcat.2013.02.003
Lv, J., Rong, Z., Sun, L., Liu, C., Lu, A.-H., Wang, Y., et al. (2018). Catalytic conversion of biomass-derived levulinic acid into alcohols over nanoporous Ru catalyst. Catal. Sci. Technol. 8, 975–979. doi: 10.1039/C7CY01838J
Makhubela, B. C. E., and Darkwa, J. (2018). The role of noble metal catalysts in conversion of biomass and bio-derived intermediates to fuels and chemicals. Johnson Matthey Technol. Rev. 62, 4–31. doi: 10.1595/205651317X696261
Mamun, O., Saleheen, M., Bond, J. Q., and Heyden, A. (2019). Investigation of solvent effects in the hydrodeoxygenation of levulinic acid to γ-valerolactone over Ru catalysts. J. Catal. 379, 164–179. doi: 10.1016/j.jcat.2019.09.026
Mehdi, H., Fábos, V., Tuba, R., Bodor, A., Mika, L. T., and Horváth, I. T. (2008). Integration of homogeneous and heterogeneous catalytic processes for a multi-step conversion of biomass: from sucrose to levulinic acid, γ-valerolactone, 1,4-pentanediol, 2-methyl-tetrahydrofuran, and alkanes. Top. Catal. 48, 49–54. doi: 10.1007/s11244-008-9047-6
Michel, C., and Gallezot, P. (2015). Why is ruthenium an efficient catalyst for the aqueous-phase hydrogenation of bio-sourced carbonyl compounds? ACS Catal 5, 4130–4132. doi: 10.1021/acscatal.5b00707
Michel, C., Zaffran, J., Ruppert, A. M., Matras-Michalska, J., Jedrzejczyk, M., Grams, J., et al. (2014). Role of water in metal catalyst performance for ketone hydrogenation: a joint experimental and theoretical study on levulinic acid conversion into gamma-valerolactone. Chem. Commun. 50, 12450–12453. doi: 10.1039/C4CC04401K
Mika, L. T., Cséfalvay, E., and Horváth, I. T. (2015). The role of water in catalytic biomass-based technologies to produce chemicals and fuels. Catal. Today 247, 33–46. doi: 10.1016/j.cattod.2014.10.043
Mizugaki, T., and Kaneda, K. (2019). Development of high performance heterogeneous catalysts for selective cleavage of C-O and C-C bonds of biomass-derived oxygenates. Chem. Rec. 19, 1179–1198. doi: 10.1002/tcr.201800075
Mizugaki, T., Togo, K., Maeno, Z., Mitsudome, T., Jitsukawa, K., and Kaneda, K. (2017). New routes for refinery of biogenic platform chemicals catalyzed by cerium oxide-supported ruthenium nanoparticles in water. Sci. Rep 7:14007. doi: 10.1038/s41598-017-14373-1
Molleti, J., Tiwari, M. S., and Yadav, G. D. (2018). Novel synthesis of Ru/OMS catalyst by solvent-free method: Selective hydrogenation of Levulinic acid to γ-valerolactone in aqueous medium and kinetic modelling. Chem. Eng. J. 334, 2488–2499. doi: 10.1016/j.cej.2017.11.125
Moreno-Marrodan, C., and Barbaro, P. (2014). Energy efficient continuous production of γ-valerolactone by bifunctional metal/acid catalysis in one pot. Green Chem. 16, 3434–3438. doi: 10.1039/c4gc00298a
Moustani, C., Anagnostopoulou, E., Krommyda, K., Panopoulou, C., Koukoulakis, K. G., Bakeas, E. B., et al. (2018). Novel aqueous-phase hydrogenation reaction of the key biorefinery platform chemical levulinic acid into γ-valerolactone employing highly active, selective and stable water-soluble ruthenium catalysts modified with nitrogen-containing ligands. Appl. Catal. B: Environ. 238, 82–92. doi: 10.1016/j.apcatb.2018.07.009
Ndolomingo, M. J., and Meijboom, R. (2019). Noble and base-metal nanoparticles supported on mesoporous metal oxides: efficient catalysts for the selective hydrogenation of levulinic acid to γ-valerolactone. Catal. Lett. 149, 2807–2822. doi: 10.1007/s10562-019-02790-y
Nenamashi, M., Noh, J.-H., and Meijboom, R. (2018). Hydrogenation of biomass-derived levulinic acid to γ-valerolactone catalyzed by mesoporous supported dendrimer-derived Ru and Pt catalysts: an alternative method for the production of renewable biofuels. Appl. Catal. A Gen. 550, 77–89. doi: 10.1016/j.apcata.2017.10.015
Omoruyi, U., Page, S., Hallett, J., and Miller, P. W. (2016). Homogeneous catalyzed reactions of levulinic acid: to γ-valerolactone and beyond. ChemSusChem 9, 2037–2047. doi: 10.1002/cssc.201600517
Ortiz-Cervantes, C., and García, J. J. (2013). Hydrogenation of levulinic acid to γ-valerolactone using ruthenium nanoparticles. Inorg. Chimica Acta 397, 124–128. doi: 10.1016/j.ica.2012.11.031
Osatiashtiani, A., Lee, A. F., and Wilson, K. (2017). Recent advances in the production of γ- valerolactone from biomass-derived feedstocks via heterogeneous catalytic transfer hydrogenation. J. Chem. Technol. Biotechnol. 92, 1125–1135. doi: 10.1002/jctb.5213
Papadogianakis, G., and Sheldon, R. A. (1996). Catalytic conversions in water: environmentally attractive processes employing water soluble transition metal complexes. New J. Chem. 20, 175–185
Papadogianakis, G., and Sheldon, R. A. (1997). Catalytic conversions in water. Part 7: An environmentally benign concept for heterogenization of homogeneous catalysis. Catalysis, Specialist Periodical Reports, Royal Soc. Chem. 13, 114–193. doi: 10.1039/9781847553256-00114
Papadogianakis, G., Verspui, G., Maat, L., and Sheldon, R. A. (1997). Catalytic conversions in water. Part 6. A novel biphasic hydrocarboxylation of olefins catalyzed by palladium TPPTS complexes (TPPTS= P(C6H4-m-SO3Na)3). Catal. Lett. 47, 43–46.
Patil, N. M., and Bhanage, B. M. (2016). Greener, recyclable, and reusable ruthenium(III) chloride/ polyethylene glycol/water system for the selective hydrogenation of biomass-derived levulinic acid to γ-valerolactone. ChemCatChem 8, 3458–3462. doi: 10.1002/cctc.201600872
Pileidis, F. D., and Titirici, M.-M. (2016). Levulinic acid biorefineries: new challenges for efficient utilization of biomass. ChemSusChem 9, 562–582. doi: 10.1002/cssc.201501405
Piskun, A., Winkelman, J. G. M., Tang, Z., and Heeres, H. J. (2016). Support screening studies on the hydrogenation of levulinic acid to γ-valerolactone in water using Ru catalysts. Catalysts 6:131. doi: 10.3390/catal6090131
Piskun, A. S., Ftouni, J., Tang, Z., Weckhuysen, B. M., Bruijnincx, P. C. A., and Heeres, H. J. (2018). Hydrogenation of levulinic acid to γ-valerolactone over anatase-supported Ru catalysts: effect of catalyst synthesis protocols on activity. Appl. Catal. A Gen. 549, 197–206. doi: 10.1016/j.apcata.2017.09.032
Primo, A., Concepción, P., and Corma, A. (2011). Synergy between the metal nanoparticles and the support for the hydrogenation of functionalized carboxylic acids to diols on Ru/TiO2. Chem. Commun. 47, 3613–3615. doi: 10.1039/c0cc05206j
Protsenko, I. I., Abusuek, D. A., Nikoshvili, L. Z., Bykov, A. V., Matveeva, V. G., and Sulman, E. M. (2018). The use of the Ru-containing catalyst based on hypercrosslinked polystyrene in the hydrogenation of levulinic acid to γ-valerolactone. Catal. Ind. 10, 301–312. doi: 10.1134/S2070050418040128
Protsenko, I. I., Nikoshvili, L. Z., Bykov, A. V., Matveeva, V. G., Sulman, A., Sulman, E. M., et al. (2017). Hydrogenation of levulinic acid using Ru-containing catalysts based on hypercrosslinked polystyrene. Green Process. Synth. 6, 281–286. doi: 10.1515/gps-2016-0189
Protsenko, I. I., Nikoshvili, L. Z., Matveeva, V. G., Sulman, E. M., and Rebrov, E. (2016). Selective hydrogenation of levulinic acid to gamma-valerolactone using polymer-based Ru-containing catalysts. Chem. Eng. Trans. 52,679–684. doi: 10.3303/CET1652114
Raspolli Galletti, A. M., Antonetti, C., De Luise, V., and Martinelli, M. (2012). A sustainable process for the production of γ-valerolactone by hydrogenation of biomass-derived levulinic acid. Green Chem. 14, 688–694. doi: 10.1039/c2gc15872h
Raspolli Galletti, A. M., Antonetti, C., Ribechini, E., Colombini, M. P., Di Nasso, N. N., and Bonari, E. (2013). From giant reed to levulinic acid and gamma-valerolactone: a high yield catalytic route to valeric biofuels. Appl. Energy 102, 157–162. doi: 10.1016/j.apenergy.2012.05.061
Ren, H.-F., Zhu, D., Li, J.-F., Liu, C.-L., Yang, R.-Z., and Dong, W.-S. (2019). One-pot conversion of carbohydrates into γ-valerolactone under the coordination of heteropoly acid based ionic liquid and Ru/ZrO2 in water media. J. Chem. Technol. Biotechnol. 94, 2355–2363. doi: 10.1002/jctb.6031
Roucoux, A., Schulz, J., and Patin, H. (2002). Reduced transition metal colloids: a novel family of reusable catalysts? Chem. Rev. 102, 3757–3778. doi: 10.1021/cr010350j
Rozenblit, A., Avoian, A. J., Tan, Q., Sooknoi, T., and Resasco, D. E. (2016). Reaction mechanism of aqueous-phase conversion of γ-valerolactone (GVL) over a Ru/C catalyst. J. Energy Chem. 25, 1008–1014. doi: 10.1016/j.jechem.2016.11.010
Ruppert, A. M., Agulhon, P., Grams, J., Wachała, M., Wojciechowska, J., Swierczynski, D., et al. (2019). Synthesis of TiO2-ZrO2 mixed oxides via the alginate route: application in the Ru catalytic hydrogenation of levulinic acid to gamma-valerolactone. Energies 12:4706. doi: 10.3390/en12244706
Ruppert, A. M., Grams, J., Jedrzejczyk, M., Matras-Michalska, J., Keller, N., Ostojska, K., et al. (2015). Titania-supported catalysts for levulinic acid hydrogenation: influence of support and its impact on γ-valerolactone yield. ChemSusChem 8, 1538–1547. doi: 10.1002/cssc.201403332
Serrano-Ruiz, J. C., Luque, R., and Sepúlveda-Escribano, A. (2011). Transformations of biomass-derived platform molecules: from high added-value chemicals to fuels via aqueous-phase processing. Chem. Soc. Rev. 40, 5266–5281. doi: 10.1039/c1cs15131b
Serrano-Ruiz, J. C., Pineda, A., Balu, A. M., Luque, R., Campelo, J. M., Romero, A. A., et al. (2012). Catalytic transformations of biomass-derived acids into advanced biofuels. Catal. Today 195, 162–168. doi: 10.1016/j.cattod.2012.01.009
Sheldon, R. A. (2014). Green and sustainable manufacture of chemicals from biomass: state of the art. Green Chem. 16, 950–963. doi: 10.1039/C3GC41935E
Simakova, I. L., and Murzin, D. Y. (2016). Transformation of bio-derived acids into fuel-like alkanes via ketonic decarboxylation and hydrodeoxygenation: design of multifunctional catalyst, kinetic and mechanistic aspects. J. Energy Chem. 25, 208–224. doi: 10.1016/j.jechem.2016.01.004
Sudhakar, M., Kantam, M. L., Jaya, V. S., Kishore, R., Ramanujachary, K. V., and Venugopal, A. (2014). Hydroxyapatite as a novel support for Ru in the hydrogenation of levulinic acid to γ-valerolactone. Catal. Commun. 50, 101–104. doi: 10.1016/j.catcom.2014.03.005
Sudhakar, M., Kumar, V. V., Naresh, G., Kantam, M. L., Bhargava, S. K., and Venugopal, A. (2016). Vapor phase hydrogenation of aqueous levulinic acid over hydroxyapatite supported metal (M= Pd, Pt, Ru, Cu, Ni) catalysts. Appl. Catal. B: Environ. 180, 113–120. doi: 10.1016/j.apcatb.2015.05.050
Tan, J., Cui, J., Deng, T., Cui, X., Ding, G., Zhu, Y., et al. (2015). Water-promoted hydrogenation of levunilic acid to γ-valerolactone on supported ruthenium catalyst. ChemCatChem 7, 508–512. doi: 10.1002/cctc.201402834
Tan, J., Cui, J., Ding, G., Deng, T., Zhu, Y., and Li, Y.-W. (2016). Efficient aqueous hydrogenation of levulinic acid to γ-valerolactone over highly active and stable ruthenium catalyst. Catal. Sci. Technol. 6, 1469–1475. doi: 10.1039/C5CY01374G
Tang, X., Zeng, X., Li, Z., Hu, L., Sun, Y., Liu, S., et al. (2014). Production of γ-valerolactone from lignocellulosic biomass for sustainable fuels and chemicals supply. Renew. Sustain. Energy Rev. 40, 608–620. doi: 10.1016/j.rser.2014.07.209
Tay, B. Y., Wang, C., Phua, P. H., Stubbs, L. P., and Huynh, H. V. (2016). Selective hydrogenation of levulinic acid to γ-valerolactone using in-situ generated ruthenium nanoparticles derived from Ru-NHC complexes. Dalton Trans. 45, 3558–3563. doi: 10.1039/C5DT03366G
Tukacs, J. M., Király, D., Strádi, A., Novodarszki, G., Eke, Z., Dibó, G., et al. (2012). Efficient catalytic hydrogenation of levulinic acid: a key step in biomass conversion. Green Chem. 14:2057–2065. doi: 10.1039/c2gc35503e
Van Bekkum, H., and Gallezot, P. (2004). Preface on the special issue “catalytic conversion of renewables”. Top Catal. 27, 1–2. doi: 10.1023/B:TOCA.0000013637.57340.ab
Van Nguyen, C., Matsagar, B. M., Yeh, J.-Y., Chiang, W.-H., and Wu, K. C.-W. (2019). MIL-53-NH2-derived carbon-Al2O3 composites supported Ru catalyst for effective hydrogenation of levulinic acid to γ-valerolactone under ambient conditions. Mol. Catal. 475:110478. doi: 10.1016/j.mcat.2019.110478
Velisoju, V. K., Peddakasu, G. B., Gutta, N., Boosa, V., Kandula, M., Chary, K. V. R., et al. (2018). Influence of support for Ru and water role on product selectivity in the vapour phase hydrogenation of levulinic acid to γ-valerolactone: investigation by probe adsorbed FT-IR spectroscopy. J. Phys. Chem. C 122, 19670–19677. doi: 10.1021/acs.jpcc.8b06003
Wei, Z., Li, Z., Deng, J., Wang, J., Li, H., and Wang, Y. (2018). Improved catalytic activity and stability for hydrogenation of levulinic acid by Ru/N-doped hierarchically porous carbon. Mol. Catal. 448, 100–107. doi: 10.1016/j.mcat.2018.01.024
Werpy, T., and Petersen, G. (2004). Top Value Added Chemicals From Biomass. Vol. I: Results of Screening for Potential Candidates From Sugars and Synthesis Gas. NREL/TP-510-35523, U.S. Department of Energy, National Renewable Energy Laboratory, Golden, CO. Avaliable online at: https://www.nrel.gov/docs/fy04osti/35523.pdf (accessed November 30, 2019)
Wojciechowska, J., Jedrzejczyk, M., Grams, J., Keller, N., and Ruppert, A. M. (2019). Enhanced production of γ-valerolactone with an internal source of hydrogen on Ca-modified TiO2 supported Ru catalysts. ChemSusChem 12, 639–650. doi: 10.1002/cssc.201801974
Xiao, C., Goh, T.-W., Qi, Z., Goes, S., Brashler, K., Perez, C., et al. (2016). Conversion of levulinic acid to gamma-valerolactone over few-layer graphene-supported ruthenium catalysts. ACS Catal. 6, 593–596. doi: 10.1021/acscatal.5b02673
Xue, Z., Liu, Q., Wang, J., and Mu, T. (2018). Valorization of levulinic acid over non-noble metal catalysts: challenges and opportunities. Green Chem. 20, 4391–4408. doi: 10.1039/C8GC02001A
Yan, K., Jarvis, C., Gu, J., and Yan, Y. (2015b). Production and catalytic transformation of levulinic acid: a platform for specialty chemicals and fuels. Renew. Sustain. Energy Rev. 51, 986–997. doi: 10.1016/j.rser.2015.07.021
Yan, K., Yang, Y., Chai, J., and Lu, Y. (2015a). Catalytic reactions of gamma-valerolactone: a platform to fuels and value-added chemicals. Appl. Catal. B: Environ. 179, 292–304. doi: 10.1016/j.apcatb.2015.04.030
Yan, N., Xiao, C., and Kou, Y. (2010). Transition metal nanoparticle catalysis in green solvents. Coord. Chem. Rev. 254, 1179–1218. doi: 10.1016/j.ccr.2010.02.015
Yao, Y., Wang, Z., Zhao, S., Wang, D., Wu, Z., and Zhang, M. (2014). A stable and efficient Ru/polyethersufone catalyst for levulinic acid hydrogenation to γ-valerolactone in aqueous solution. Catal. Today 234, 245–250. doi: 10.1016/j.cattod.2014.01.020
Ye, L., Han, Y., Feng, J., and Lu, X. (2020). A review about GVL production from lignocellulose: Focusing on the full components utilization. Ind. Crops Prod. 144:112031. doi: 10.1016/j.indcrop.2019.112031
Yu, Z., Lu, X., Liu, C., Han, Y., and Ji, N. (2019). Synthesis of γ-valerolactone from different biomass-derived feedstocks: recent advances on reaction mechanisms and catalytic systems. Renew. Sustain. Energy Rev. 112, 140–157. doi: 10.1016/j.rser.2019.05.039
Yuan, Y., Yan, N., and Dyson, P. J. (2012). Advances in the rational design of rhodium nanoparticle catalysts: control via manipulation of the nanoparticle core and stabilizer. ACS Catal. 2, 1057–1069. doi: 10.1021/cs300142u
Keywords: hydrogenation, renewable, platform chemical, levulinic acid, γ-valerolactone, water, biofuels, biorefinery
Citation: Seretis A, Diamantopoulou P, Thanou I, Tzevelekidis P, Fakas C, Lilas P and Papadogianakis G (2020) Recent Advances in Ruthenium-Catalyzed Hydrogenation Reactions of Renewable Biomass-Derived Levulinic Acid in Aqueous Media. Front. Chem. 8:221. doi: 10.3389/fchem.2020.00221
Received: 04 February 2020; Accepted: 09 March 2020;
Published: 21 April 2020.
Edited by:
Manoj B. Gawande, Palacky University Olomouc, CzechiaReviewed by:
Benjaram M. Reddy, Indian Institute of Chemical Technology (CSIR), IndiaAnna Maria Raspolli Galletti, University of Pisa, Italy
Copyright © 2020 Seretis, Diamantopoulou, Thanou, Tzevelekidis, Fakas, Lilas and Papadogianakis. This is an open-access article distributed under the terms of the Creative Commons Attribution License (CC BY). The use, distribution or reproduction in other forums is permitted, provided the original author(s) and the copyright owner(s) are credited and that the original publication in this journal is cited, in accordance with accepted academic practice. No use, distribution or reproduction is permitted which does not comply with these terms.
*Correspondence: Georgios Papadogianakis, cGFwYWRvZ2lhbmFraXMmI3gwMDA0MDtjaGVtLnVvYS5ncg==