- 1School of Materials Engineering, Shanghai University of Engineering Science, Shanghai, China
- 2School of Management, University of Shanghai for Science and Technology, Shanghai, China
The performance of anodes of lithium-ion batteries relies largely on the architecture and composition of the hybrid active materials. We present a two-step, seed-free, solution-based method for the direct growth of hierarchical charantia-like TiO2/Fe2O3 core/shell nanotube arrays on carbon cloth substrates. An ultrahigh loading of the nanomaterial on carbon fibers was achieved with this method without the use of a binder. This three-dimensional porous hollow architecture and its direct contact with the CC current collector ensure an efficient electronic pathway. The hollow TiO2 framework effectively protects the hierarchical charantia-like TiO2/Fe2O3 hollow core/shell arrays from collapsing because of its negligible volume change during cycling. Meanwhile, the self-assembled α-Fe2O3 hollow nanospheres guarantee a large capacity and contact area with the electrolyte. This flexible anode with a 3D porous charantia-like hollow architecture exhibits high cycle performance, reversible capacity, and rate capability. These nanotube arrays maintain a high reversible capacity of 875 mAh g−1 after 200 cycles at a current density of 200 mA g−1. This simple, cost-effective, and scalable electrode fabrication strategy can be implemented in the fabrication of high-performance wearable energy storage devices.
Introduction
Lithium-ion batteries (LIBs) are popular energy storage devices because of their high energy density, high open-circuit voltage, lack of memory effects, and shape controllability (Joshi et al., 2016; Zhao et al., 2016; Shen et al., 2018; Deng et al., 2019). The design of electrode materials with high energy density and cycling stability is important for enhancing the performance of LIBs. Graphite—the most widely used commercial anode material—cannot fulfill the increasing demands with regard to the energy density and stability of an electrode owing to its low theoretical capacity (372 mAh g−1), poor rate performance, and intrinsic stability. Therefore, much attention has been focused on exploring anode materials with higher capacity for next-generation LIBs (Liu et al., 2019; Partheeban and Sasidharan, 2019; Shen et al., 2019).
TiO2 is a promising anode candidate owing to its relatively good conductivity, low cost, higher operation voltage, and low volume expansion. However, its practical application is impeded by its low theoretical capacity. Owing to its unique physical and chemical properties as a nanomaterial, TiO2 is engineered into various nanostructures with different morphologies to improve the specific capacity (Moretti et al., 2013; Ying et al., 2017; Smith et al., 2019). Despite immense progress in this area, the effect of scaling down on the performance of TiO2 has been limited due to its theoretical capacity limitation. Recent studies have indicated that TiO2-based nanocomposites obtained by combining them with high-capacity metal oxides could facilitate high performance at a smaller scale (Zhu et al., 2014; Wang et al., 2015; Lee et al., 2017; Yang et al., 2018; Zhang et al., 2019). Hematite (α-Fe2O3) is a promising mineral for preparing a hybrid with TiO2 because of its relatively higher theoretical capacity (~1,000 mA h g−1), non-toxicity, high corrosion resistance, and abundant resource (Gao et al., 2014; Li et al., 2016; Jiang et al., 2017; Zhao et al., 2019). When Fe2O3 is anchored onto the surface of a TiO2 nano-backbone, its high theoretical capacity compensates for the deficient capacity of the TiO2 nano-backbone, while the good conductivity and low volume expansion of TiO2 ensures excellent cyclic stability of the hybrid material (Luo et al., 2012; Yang et al., 2017). One-dimensional (1D) nanotube arrays and three-dimensional (3D) hollow spheres can promote rate capability by offering an efficient electronic pathway (Han et al., 2011; Wang et al., 2012; He et al., 2018). Therefore, we fabricated 3D nanotube arrays by coating Fe2O3 hollow nanospheres on TiO2 nanotube arrays to realize high-performance rechargeable batteries. However, the direct growth of a 3D porous hollow composite architecture on a flexible substrate without a binder remains a major challenge.
In this study, 3D hierarchical charantia-like TiO2/Fe2O3 nanotube arrays were grown directly on a carbon cloth (CC) substrate by a facile hydrothermal method. The CC/TiO2 nanotube arrays decreased the fracture risk caused by the volume fluctuation during lithium-ion insertion and extraction, while the α-Fe2O3 nanosphere shell assembled on the surface of TiO2 nanotube increased the surface area and number of active sites. Direct contact of the active material with the CC substrate and the hollow structure facilitated an efficient electronic pathway. Their unique hollow core-shell structure endowed the final electrodes with high energy, reversible capacity, and cycle performance.
Experimental Method
Synthesis of TiO2 Nanotube Arrays on CC
Before the experiment, the CC substrates were immersed in nitric acid at 30°C for 3 h, and then ultrasonically cleaned with distilled water and ethanol for 30 min. TiO2 nanotube arrays were synthesized by a facile hydrothermal method. Analytically pure reagents were used without further purification. Typically, 1 mL of tetrabutyl titanate, 12 mL of glycerinum, and 45 mL of ethanol were magnetically stirred at room temperature to form a homogeneous precursor solution. The precursor was then poured into a 100 mL Teflon-lined autoclave with a piece of clean CC (7 ×5.0 cm) and maintained at 175°C for 20 h. After the reaction, the white byproduct on the CC was washed with ethanol and distilled water, and CC/TiO2 nanotube arrays were obtained after heating the products at 350°C for 1 h.
Growth of Fe2O3 Nanospheres on CC/TiO2 Nanotube Arrays
The as-prepared CC/TiO2 nanotube arrays were used as scaffolds for growing α-Fe2O3 hollow nanospheres via a simple hydrothermal method. FeCl3·6H2O (1.688 g) and ammonia (0.1 mL) were dissolved in 40 mL of deionized water in a 100 mL Pyrex beaker and stirred to form a clear tan solution. The final mixture was transferred to an 80 mL Teflon-lined autoclave and heated at 95°C for 4 h. The products were washed several times with ethanol and water. The dried products were further annealed in a N2 atmosphere at 450°C for 2 h.
Characterization of the Structure and Morphology
Phase purity of the synthesized products was examined by X-ray powder diffraction (XRD, Panalytical X'Pert, Netherlands) carried out with Cu-Kα radiation (λ = 1.5418 Å). The morphology of the products was characterized by field-emission scanning electron microscopy (SEM, Model: JEOL JSM-7000F, Japan) and high-resolution transmission electron microscopy (HRTEM, Model: FEI Titan X 60-300, USA). FA1004 electronic balance was used to measure the weight difference between the prepared electrode and CC substrate, and the mass loading of the active material was obtained. X-ray photoelectron spectroscopy (XPS) was performed on a Perkin-Elmer model PHI 5600 XPS system, with a monochromated aluminum anode as the X-ray source. The specific surface area of the obtained products was calculated by the Brunauer–Emmett–Teller (BET) method using the nitrogen adsorption–desorption isotherm acquired from a Micrometics Tristar 3000 system.
Electrochemical Measurements
Electrochemical tests were performed in CR 2032 coin half-cells assembled in an argon-filled glovebox using the as-prepared hierarchical nanotube arrays as the anode and circular lithium-metal foil as the cathode. A solution of 1 M LiPF6 in ethylene carbonate and diethyl carbonate at a volume ratio of 1:1 was used as the electrolyte. The cells were galvanostatically charged and discharged using a multichannel battery tester (Neware-CT3008). With lithium-metal foil as the counter and reference electrodes, Cyclic voltammetry (CV) of the coin half-cells was performed on a PARSTAT 4000 electrochemical workstation at a scan rate of 0.2 mV s−1 in the range of 3.0–0.01 V vs. Li/Li+. Electrochemical impedance spectroscopy (EIS) was performed in a wide frequency range of 100 kHz to 0.01 Hz at an AC perturbation voltage of 5 mV.
Results and Discussion
Synthesis and Characterization
Scheme 1 shows the synthesis of the 3D hierarchical charantia-like CC/TiO2/Fe2O3 nanotube arrays. First, the TiO2 nanotube arrays were fabricated on a CC substrate by a facile seed-free hydrothermal method. After annealing the obtained product in air at 350°C for 1 h, the amorphous TiO2 cores crystallized into one-dimensional (1D) CC/TiO2 nanotube arrays. Subsequently, ultrathin FeOOH nanosheets were grown on the surface of the CC/TiO2 nanotube arrays and then spontaneously transformed into hollow nanospheres by the forced hydrolysis of FeCl3, without the use of any structure-directing agent. FeOOH hollow nanospheres grown on the CC/TiO2 nanotube arrays were further transformed into α-Fe2O3 hollow nanospheres through thermal dehydroxylation and lattice shrinkage at a high temperature, leading to the formation of the charantia-like CC/TiO2/Fe2O3 nanotube arrays.
XRD measurements were performed to confirm the crystalline structure of the obtained products. Figure 1A shows the corresponding XRD patterns of TiO2 and TiO2/Fe2O3 products scratched from CC substrates. All the diffraction peaks of the TiO2 products matched well with those of typical tetragonal rutile TiO2 (JCPDS No. 21-1272). The lattice parameters of the obtained tetragonal rutile TiO2 nanotube are a = b = 4.516 Å and c = 5.205 Å. These are consistent with those reported by Luo et al. (2012). Three additional peaks were observed in the XRD pattern of the TiO2/Fe2O3 composite. These peaks located at 33.1, 35.6, and 54.1° correspond to the (104), (110), and (116) planes of α-Fe2O3 (JCPDS No. 33-0664), respectively, indicating the successful preparation of an α-Fe2O3 shell on the TiO2 nanotube core (Larcher et al., 2003; Qi et al., 2018).
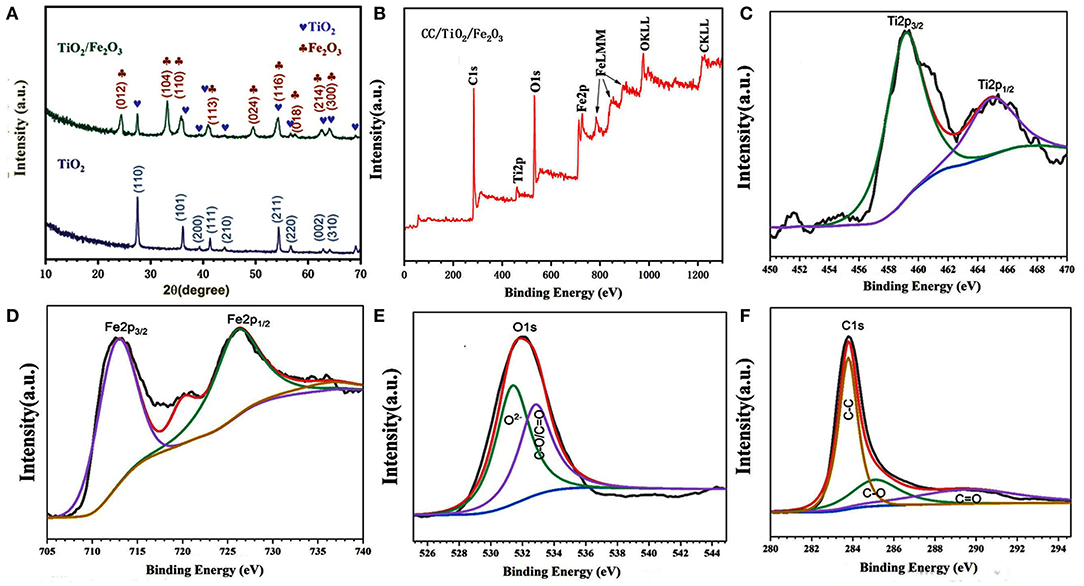
Figure 1. (A) XRD pattern of CC/TiO2 and CC/TiO2/Fe2O3 hollow nano-arrays. XPS spectra of CC/TiO2/Fe2O3 specimens. (B) Survey spectra. (C) Ti2p core-level spectra, (D) Fe2p core-level spectra. (E) O1s core-level spectra, and (F) C1s core-level spectra.
XPS was performed to identify the chemical states and surface composition of the final composite. The spectra are shown in Figures 1B–F. Only the peaks of Ti, O, Fe, and C are found in the survey spectrum of the CC/TiO2/Fe2O3 composite. The XPS spectrum of the C 1s core-level could be divided into three peaks (Figure 1C). The peak at 283.7 eV indicates the non-oxygenated carbon. The other two peaks at 284.9 and 289.1 eV correspond to the carbon ramifications generated in the hydrothermal reaction and annealing process. The deconvoluted peaks of the O 1s spectrum could also be decomposed into two components centered at 531.3 and 533.8 eV, respectively. The low binding energy component is attributed to the attached to Ti and Fe, the other component corresponds to the oxygen in C–O and O–C=O bonds. The Ti 2p XPS spectrum exhibits bimodal characteristics. The peaks located at 460 and 466 eV correspond to Ti 2p3/2 and Ti 2p1/2, respectively (Wang et al., 2016). The binding energy difference of 5.8 eV between the Ti 2p1/2 and Ti 2p3/2 core levels indicated that the oxidation state of Ti is mainly Ti (4+). Similarly, the typical bimodal characteristics of the Fe 2p XPS spectrum also confirmed the existence of surface Fe as a trivalent oxide (Luo et al., 2012; Yang et al., 2017). Consistent with the results of XRD, the XPS analysis revealed that the obtained composites are composed of α-Fe2O3 and TiO2.
The morphology and microstructure of the as-obtained composite were characterized by SEM and TEM. Figures 2a–c show the SEM images of pure CC/TiO2 nanotube arrays at different magnifications. It is obvious that the 1D TiO2 nanotube arrays grew uniformly and vertically on the CC substrate in a carambola-like configuration. The high-magnification SEM images clearly show that the 1D carambola-like TiO2 nanotube arrays have a mean bottom diameter of 500 nm. Their walls were assembled from several small nanosheets, leading to the roughening of the surface of the TiO2 nanotube arrays. A rough surface facilitates the heterogeneous nucleation of Fe2O3 nanoparticles. Figures 2d–f present the SEM images of CC/TiO2/Fe2O3 nanocomposite arrays at different magnifications. As seen, a large number of nanospheres adhered to the surface of the carambola-like TiO2 nanotube arrays, forming a 3D hierarchical charantia-like structure. This association rendered the final nanotube arrays more compact and spatially dense, thus guaranteeing a high mass loading and high specific capacity. The mass loading of CC/TiO2/Fe2O3 nanocomposite arrays was ~11.6 mg cm–2, higher than that of the pure TiO2/CC nano-arrays (~5.1 mg cm−2). Owing to these features, the final nanotube arrays can remain ordered without undergoing any apparent structural collapse or breakage. Compared with the bare TiO2 nanotube arrays, the bottom diameters of the 3D hierarchical CC/TiO2/Fe2O3 nano-composite arrays range up to 700 nm, with negligible variation in the length. The surface of the charantia-like CC/TiO2/Fe2O3 nanotube arrays is rougher and looser than that of the 1D bare CC/carambola-like TiO2 nanotube arrays. These characteristics improved the effective contact area with the electrolyte. Furthermore, the robust bottom of the charantia-like nano-composite arrays increased their mechanical strength, thus reducing the fracture risk triggered by volume variations during lithiation/delithiation. This unique structure may lead to a large specific capacity and high cycling stability of the final product (Joshi et al., 2016; Zhang et al., 2019). Figure 2g displays the EDX mapping analysis of the charantia-like CC/TiO2/Fe2O3 nanotube arrays. Fe, Ti, and O are evenly distributed on the surface of carbon fibers, further implying that the nanospheres coated on the surface of 1D TiO2 nanotube arrays are iron oxides.
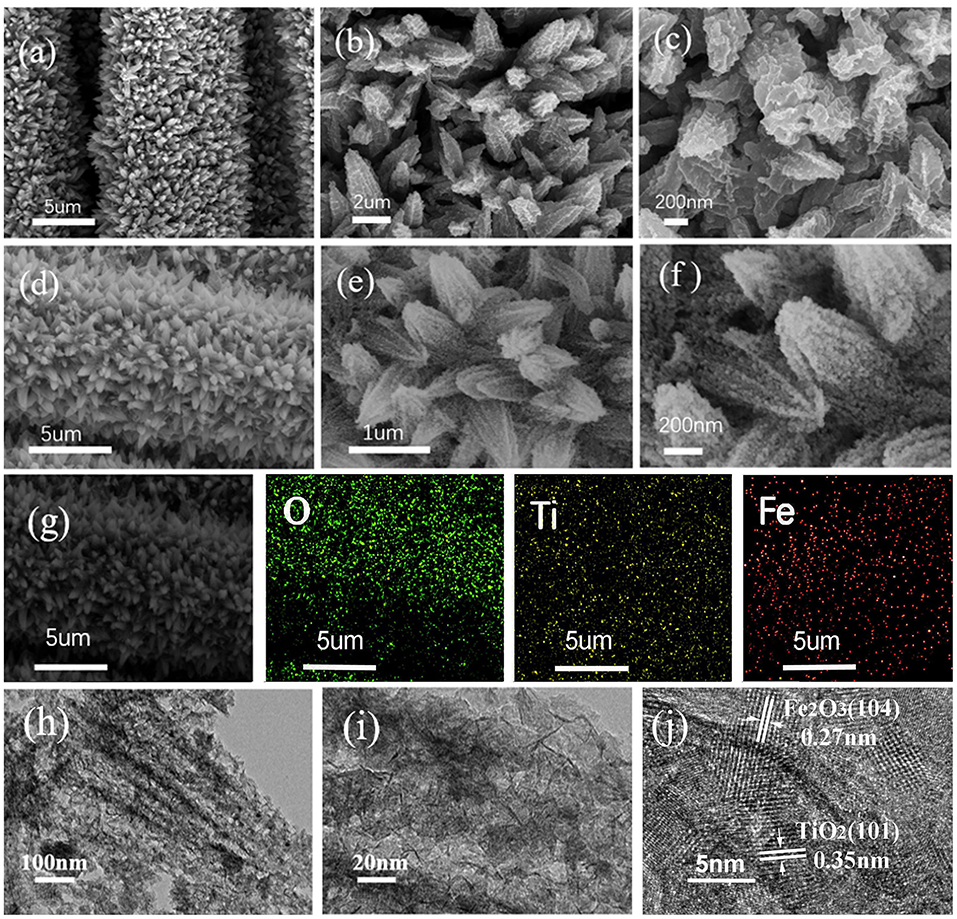
Figure 2. SEM images of the prepared specimens at different magnifications. (a–c) CC/TiO2 specimens. (d–f) CC/TiO2/Fe2O3 specimens. (g) EDX elemental mapping of the CC/TiO2/Fe2O3 specimens. (h–j) TEM and HRTEM images of CC/TiO2/Fe2O3 specimens.
To investigate the microstructure of the charantia-like nanotube arrays, further TEM observations were made. Figures 2h–j show the TEM images of the nanotube arrays scraped off the CC substrate. Figure 2h shows that both the TiO2 cores and Fe2O3 nanospheres have hollow interiors. The hollow Fe2O3 nanospheres are loosely assembled from numerous small nanosheets, indicating that they might have a mesoporous structure. Further, the HRTEM image shows that the lattice spacings of the 3D hierarchical charantia-like CC/TiO2/Fe2O3 nanotube arrays are 0.35 and 0.27 nm, corresponding to the (101) plane of tetragonal rutile TiO2 and the (110) plane of α-Fe2O3, respectively (Luo et al., 2012; Qi et al., 2018). This result further confirms that the nanotube arrays were composed of tetragonal rutile TiO2 and α-Fe2O3.
BET gas-sorption measurements were performed to further investigate the porous nature and the specific surface area of the nanotube arrays. Figure 3A presents the nitrogen adsorption–desorption isotherm of the nanotube arrays. The typical IV isotherm with a distinct hysteresis loop in the range of 0.7–1.0 P/Po indicates that the charantia-like TiO2/Fe2O3 nanotube arrays have a mesoporous structure (Luo et al., 2013; Zhang et al., 2019). The BET specific surface area of the nanotube arrays was calculated to be 149.3 m2 g−1. The large specific surface area is mainly due to the extremely uneven charantia-like surface resulting from the well-wrapped Fe2O3 hollow nanospheres. The pore size distribution (Figure 3B) demonstrates that the main pore size of the nanotube arrays is approximately 13.4 nm. According to the literature (Luo et al., 2012; Wang et al., 2016; Zhang et al., 2019), such a mesoporous structure can offer enough interface for lithium-ion insertion and extraction.
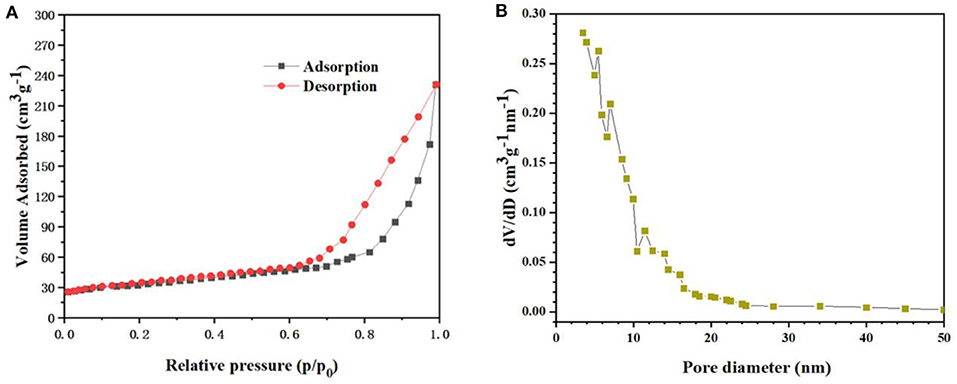
Figure 3. (A) The N2 adsorption/desorption isotherms and (B) pore size distribution of CC/TiO2/Fe2O3 hollow nano-arrays.
Electrochemical Properties
The electrochemical properties of the 3D hierarchical charantia-like CC/TiO2/Fe2O3 nanotube arrays were evaluated to further confirm the performance of the prepared nanotube arrays. Figure 4A shows the first three CV cycles of a single redox couple in the voltage window of 0–3.8 V at a scanning rate of 0.5 mV/s. The first CV curve shows two broad cathodic peaks at 1.05 and 0.42 V, which mainly correspond to the generation of Li2O as well as the formation of a solid-electrolyte interface (SEI) (Luo et al., 2012; Yang et al., 2017). In the anodic process, the peak at 1.4 V indicates the oxidation of zero-valent iron to ferric iron, while the weak peak at 2.3 V is ascribed to the delithiation of TiO2. Except for the slight variation in the two cathodic peaks, the subsequent curves exhibit good reproducibility. The slight shift in the two cathodic peaks to higher potential reveal the occurrence of some irreversible processes during the first cycle. This result is consistent with the previous findings reported in literature (Luo et al., 2012; Qi et al., 2018; Zhang et al., 2019).
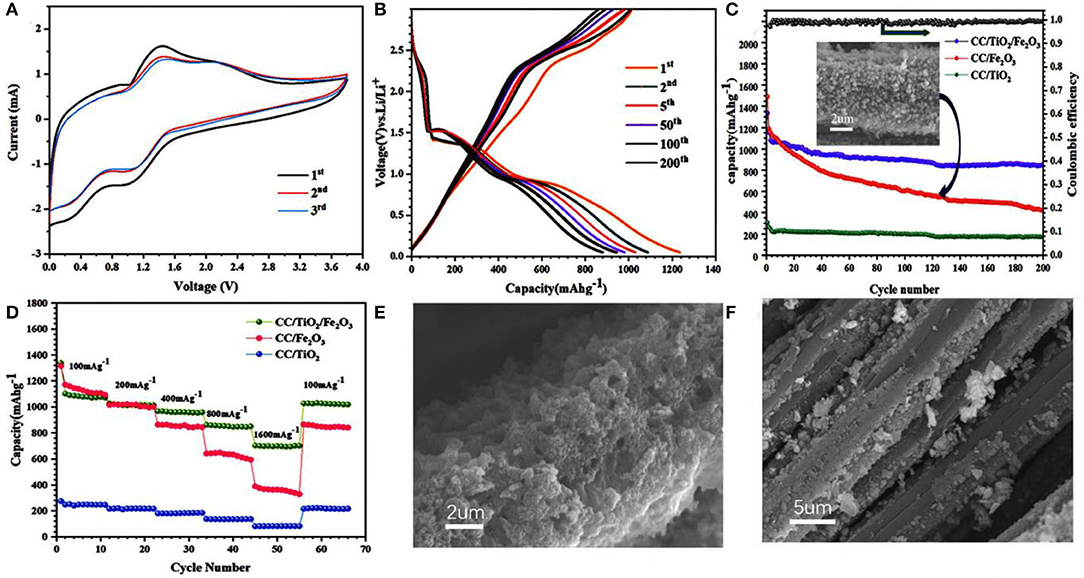
Figure 4. (A) CV curves of CC/TiO2/Fe2O3 specimens between 5 mV and 3.8 V at a scan rate of 0.5 mVs−1. (B) Charge-discharge voltage profiles of CC/TiO2/Fe2O3 specimens at a constant current rate of 200 mAg−1. (C) Cycling performances of CC/TiO2/Fe2O3, CC/TiO2, and CC/Fe2O3 specimens at a constant current rate of 200 mA g−1. (D) Rate performances of CC/TiO2/Fe2O3, CC/TiO2, and CC/Fe2O3 specimens. SEM image of (E) CC/TiO2/Fe2O3 and (F) CC/Fe2O3 specimens after 200 cycles.
Figure 4B shows different cycle charge–discharge voltage profiles of the 3D hierarchical charantia-like CC/TiO2/Fe2O3 nanotube arrays at a constant current density of 200 mA g−1. The plateau voltages in the first discharge curve are in good agreement with the oxidation peaks in the CV curves. The initial discharge and charge capacities are ~1,280 and 1,089 mAh g−1, respectively, corresponding to a low irreversible capacity loss (~15%). Figure 4C shows the cycling performances of the CC/TiO2/Fe2O3 nanotube arrays as along with those of CC/TiO2 nanotube arrays and CC/Fe2O3 composites. The CC/Fe2O3 composites were synthesized from clean CC using the same Fe ion hydrothermal system mentioned in the experimental section. Although the bare CC/TiO2 nanotube arrays presented a perfect cycle performance, they had a low capacity. On the contrary, despite having a high capacity in the initial stage, the CC/Fe2O3 composite showed a rapid decay of the capacity with cycling. The apparent decline in the capacity is mainly due to the drastic volume changes of Fe2O3 itself during lithiation, which led to the removal of the active material from the CC substrate. By contrast, the combination of low volume expansion of the TiO2 hollow cores and the strong adhesion between the two types of metallic oxides rendered the structure of the 3D nanotube arrays stable during lithium-ion insertion/extraction processes. This feature further led to high cyclic capacity retention of the nanotube arrays. Even after 200 cycles, the discharge capacity of the CC/TiO2/Fe2O3 nanotube arrays was maintained at approximately 896 mAh g−1, which is much higher than that of the bare CC/TiO2 nanotube arrays and CC/Fe2O3 composites. At the 200th cycle, the Coulombic efficiency of the nanotube array electrodes was approximately 98.7%. Figure 4D displays the rate performance of the tested electrodes in the rate range of 100–1,600 mA g−1. The CC/TiO2/Fe2O3 nanotube array electrode maintained a discharge capacity of approximately 742 mAh g−1 even when the discharge rate was increased to 1,600 mA g−1. Meanwhile, the discharge capacity of the CC/Fe2O3 composite electrode was only approximately 397.5 mA g−1 at 1,600 mA g−1. Compared the previous literature (Luo et al., 2012, 2013; Xia et al., 2014), these charantia-like nanotube arrays exhibit superior capacity and cyclability due to their unique double hollow structure (Table 1).
Figures 4E,F illustrate the SEM images of the CC/TiO2/Fe2O3 nanotube array and the CC/Fe2O3 composite electrodes after 200 cycles. The former maintained an integral framework and was tightly adsorbed to the surface of the CC substrate after 200 cycles. Under the same condition, the volume of Fe2O3 itself changed unprecedentedly upon cycling, resulting in a large alternate stress. As a result, the CC/Fe2O3 composite severely fragmented and extensively separated from the CC substrate. Consistent with the charge–discharge test results, the electrical contact loss significantly reduced the cycling life of the CC/Fe2O3 composite electrode.
To gain further insight into the electrochemical performance of the test specimens, EIS measurements were carried out at room temperature. Figures 5A,B show the Nyquist plots of the test specimens at their open-circuit voltage. All the test specimens exhibited semi-circular Nyquist plots in the high-frequency region and a straight line in the low-frequency region. The diameter of the semicircle represents the charge-transfer resistance (RCT), while the slope of the line corresponds to the mass transfer of lithium ions (W) (Shen et al., 2019; Zhang et al., 2019). By adding the Ohmic resistance (Rs) and double-layer capacitance (CPE), the electrochemical system can be fitted to the Randles circuit shown in Figure 5A. As shown in Figure 5, the diameters of the semicircle of the 3D hierarchical charantia-like CC/TiO2/Fe2O3 nanotube arrays and bare CC/TiO2 nano-arrays are smaller than that of the CC/Fe2O3 composite, indicating that the conductivity of the hollow CC/TiO2/Fe2O3 nano-arrays can be effectively improved by their TiO2 nanotube cores. After 200 cycles, the charge-transfer resistance of the CC/TiO2/Fe2O3 nanotube arrays and bare CC/TiO2 nanotube arrays increased marginally, while that of the CC/Fe2O3 composites increased significantly.
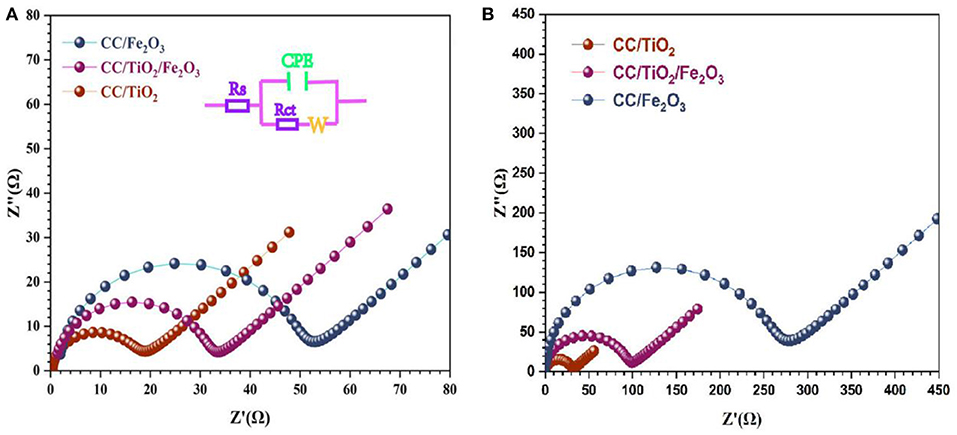
Figure 5. Impedance analysis of CC/TiO2/Fe2O3, s CC/TiO2, and CC/Fe2O3 specimens for (A) fresh cell and (B) 50th cycled cell.
Conclusions
3D hierarchical charantia-like TiO2/Fe2O3 nanotube arrays were fabricated on CC by a simple, two-step, seed-free hydrothermal method. The well-wrapped mesoporous Fe2O3 nanospheres on TiO2 nanotubes endowed the final nanotube arrays with a larger specific surface area. The hollow framework and its direct contact with the CC current collector led to the good conductivity of the electrode. The low volume expansion of the hollow TiO2 cores combined with the strong adhesion between the two types of metal oxides stabilized the structure of the final nanotube arrays during cycling. The electrochemical results revealed that the charantia-like TiO2/Fe2O3 nanotube arrays have high reversible capacity, improved cycling stability, and excellent rate capability. After 200 cycles at a current density of 200 mA g−1, the capacity of the nanotube arrays remained at 875 mAh g−1. A rate capability of ~742 mAh g−1 was achieved even when the discharge rate was increased to 1,600 mA g−1.
Data Availability Statement
The datasets generated for this study are available on request to the corresponding author.
Author Contributions
PX, HZ, AS, and YZha conducted the synthesis. PX, YZha, and YW carried out the characterization and the electrochemical measurements. PX and ZZ co-wrote the manuscript. All authors discussed the data and commented on the manuscript.
Funding
This work was supported by National Natural Science Foundation of China (grant no. 71401106), Natural Science Foundation of Shanghai (grant no. 14ZR1418700), and Shanghai University of Engineering Science Innovation Fund (grant no. 18KY0504).
Conflict of Interest
The authors declare that the research was conducted in the absence of any commercial or financial relationships that could be construed as a potential conflict of interest.
Acknowledgments
The authors would like to thank Minjie Yan for his help with SEM/EDX analysis.
References
Deng, S., Zhang, Y, Xie, D., Yang, L., Wang, G., Zheng, X. S., et al. (2019). Oxygen vacancy modulated Ti2Nb10O29−x embedded onto porous bacterial cellulose carbon for highly efficient lithium ion storage. Nano Energy 58, 355–364. doi: 10.1016/j.nanoen.2019.01.051
Gao, G., Yu, L., Wu, H. B., and Lou, X. W. (2014). Hierarchical tubular structures constructed by carbon-coated α-Fe2O3 nanorods for highly reversible lithium storage. Small 10, 1741–1745. doi: 10.1002/smll.201303818
Han, H., Song, T., Bae, Y. J., Nazar, L. F., Kim, H., and Paik, U. (2011). Nitridated TiO2 hollow nanofibers as an anode material for high power lithium ion batteries. Energy Environ. Sci. 4, 4532–4536. doi: 10.1039/c1ee02333k
He, J., Chen, Y., and Manthiram, A. (2018). Vertical Co9S8 hollow nanowall arrays grown on a Celgard separator as a multifunctional polysulfide barrier for high-performance Li-S batteries. Energy Environ. Sci. 67611, 2560–2568. doi: 10.1039/C8EE00893K
Jiang, T., Bu, F., Feng, X., Shakir, I., Hao, G., and Xu, Y. (2017). Porous Fe2O3 nanoframeworks encapsulated within three-dimensional graphene as high-performance flexible anode for lithium-ion battery. ACS Nano 11, 5140–5147. doi: 10.1021/acsnano.7b02198
Joshi, B. N., An, S., Jo, H. S., Song, K. Y., Park, H. G., Hwang, S., et al. (2016). Flexible, freestanding, and binder-free SnOx-ZnO/carbon nanofiber composites for lithium ion battery anodes. ACS Appl. Mater. Interfaces 814, 9446–9453. doi: 10.1021/acsami.6b01093
Larcher, D., Bonnin, D., Cortes, R., Rivals, I., Personnaz, L., and Tarascon, J. M. (2003). Combined XRD, EXAFS, and mossbauer studies of the reduction by lithium of alpha-Fe2O3 with various particle size. J. Electrochem. Soc. 150, A1643–A1650. doi: 10.1149/1.1622959
Lee, G., Kim, S., Kim, S., and Choi, J. (2017). SiO2/TiO2 composite film for high capacity and excellent cycling stability in lithium-ion battery anodes. Adv. Funct. Mater. 27:1703538. doi: 10.1002/adfm.201703538
Li, Y. Y., Cai, Q. F., Wang, L., Li, Q. W., Peng, X., Gao, B., et al. (2016). Mesoporous TiO2 nanocrystals/graphene as an efficient sulfur host material for high-performance lithium-sulfur batteries. ACS Appl. Mater. Interfaces 8, 23784–23792. doi: 10.1021/acsami.6b09479
Liu, B., Zhang, Y., Pan, G., Ai, C., Deng, S., Liu, S., et al. (2019). Ordered lithiophilic sites to regulate Li plating/stripping behavior for superior lithium metal anodes. J. Mater. Chem. A 7, 21794–21801. doi: 10.1039/C9TA09502K
Luo, J. S., Xia, X. H., Luo, Y. S., Guan, C., Liu, J. L., Qi, X. Y., et al. (2013). Rationally designed hierarchical TiO2@Fe2O3 hollow nanostructures for improved lithium ion storage. Adv. Energy Mater. 3, 737–743. doi: 10.1002/aenm.201200953
Luo, Y., Luo, J., Jiang, J., and Zhou, W. (2012). Seed-assisted synthesis of highly ordered TiO2@α-Fe2O3 core/shell arrays on carbon textiles for lithium-ion battery applications. Energy Environ. Sci. 5, 6559–6566. doi: 10.1039/c2ee03396h
Moretti, A., Kim, G. T., Bresser, D., Renger, K., Paillard, E., Marassi, R., et al. (2013). Investigation of different binding agents for nanocrystalline anatase TiO2 anodes and its application in a novel, green lithium-ion battery. J. Power Sources 221, 419–426. doi: 10.1016/j.jpowsour.2012.07.142
Partheeban, T., and Sasidharan, M. (2019). Template-free synthesis of LiV3O8 hollow microspheres as positive electrode for Li-ion batteries. J. Mater Sci. 55, 2155–2165. doi: 10.1007/s10853-019-04086-3
Qi, X., Zhang, H., Zhang, Z., Bian, Y., Shen, A., Xu, P., et al. (2018). Subunits controlled synthesis of three-dimensional hierarchical flower-like α-Fe2O3 hollow spheres as high-performance anodes for lithium ion batteries. Appl. Surf. Sci. 452, 174–180. doi: 10.1016/j.apsusc.2018.04.253
Shen, S., Guo, W., Xie, D., Wang, Y., Deng, S., Zhong, Y., et al. (2018). A synergistic vertical graphene skeleton and S–C shell to construct high-performance TiNb2O7-based core/shell arrays. J. Mater. Chem. A 6, 20195–20204. doi: 10.1039/C8TA06858E
Shen, S., Xia, X., Zhong, Y., Deng, S., Xie, D., Liu, B., et al. (2019). Implanting niobium carbide into trichoderma spore carbon: a new advanced host for sulfur cathodes. Adv. Mater. 31:1900009. doi: 10.1002/adma.201900009
Smith, K. A., Savva, A. I., Mao, K. S., Wang, Y., Tenne, D. A., Chen, D., et al. (2019). Effect of proton irradiation on anatase TiO2 nanotube anodes for lithium-ion batteries. J Mater Sci. 54, 13221–13235. doi: 10.1007/s10853-019-03825-w
Wang, C., Wu, L., Wang, H., Zuo, W., Li, Y., and Liu, J. (2015). Fabrication and shell optimization of synergistic TiO2-MoO3 core-shell nanowire array anode for high energy and power density lithium-ion batteries. Adv. Funct. Mater. 25, 3524–3533. doi: 10.1002/adfm.201500634
Wang, X., Fan, L., Gong, D., Zhu, J., Zhang, Q., and Lu, B. (2016). Core-shell Ge@Graphene@TiO2 nanofibers as a high-capacity and cycle-stable anode for lithium and sodium Ion battery. Adv. Funct. Mater. 26, 1104–1111. doi: 10.1002/adfm.201504589
Wang, Z., Luan, D., Madhavi, S., Hu, Y., and Lou, X. (2012). Assembling carbon-coated α-Fe2O3 hollow nanohorns on the CNT backbone for superior lithium storage capability. Energy Environ. Sci. 5, 5252–5256. doi: 10.1039/C1EE02831F
Xia, H., Xiong, W., Lim, C. K., Yao, Q. F., Wang, Y. D., and Xie, J. P. (2014). hierarchical TiO2-B nanowire@α-Fe2O3 nanothorn core-branch arrays as superior electrodes for lithium ion microbatteries. Nano Res. 7, 1797–1808. doi: 10.1007/s12274-014-0539-3
Yang, J., Wu, Q., Yang, X., He, S., Khan, J., Meng, Y., et al. (2017). Chestnut-like TiO2@α-Fe2O3 core-shell nanostructures with abundant interfaces for efficient and ultralong life lithium-ion storage. ACS Appl. Mater. Inter. 9, 354–361. doi: 10.1021/acsami.6b12150
Yang, Y., Wang, S., Lin, S., Li, Y., Zhang, W., Chao, Y., et al. (2018). Rational design of hierarchical TiO2/epitaxially aligned MoS2-carbon coupled interface nanosheets core/shell architecture for ultrastable sodium-ion and lithium-sulfur batteries. Small Methods 2:1800119. doi: 10.1002/smtd.201800119
Ying, W., Yu, J., Shi, J., Gu, L., and Yu, Y. (2017). Multichannel porous TiO2 hollow nanofibers with rich oxygen vacancies and high grain boundary density enabling superior sodium storage performance. Small 13:1700129. doi: 10.1002/smll.201700129
Zhang, Z., Xu, P., Zhang, H., Shen, A., and Zhao, Y. (2019). Flexible three-dimensional titanium-dioxide-based hollow nanoflower arrays for advanced lithium-ion battery anodes. ACS Appl. Energy Mater. 2, 5744–5752. doi: 10.1021/acsaem.9b00869
Zhao, Q., Liu, J., Li, X., Xia, Z., Zhang, Q., Zhou, M., et al. (2019). Graphene oxide-induced synthesis of button-shaped amorphous Fe2O3/rGO/CNFs films as flexible anode for high-performance lithium-ion batteries. Chem. Eng. J. 369, 215–222. doi: 10.1016/j.cej.2019.03.076
Zhao, Y., Li, X., Yan, B., Xiong, D., Li, D., Lawes, S., et al. (2016). Recent developments and understanding of novel mixed transition-metal oxides as anodes in lithium ion batteries. Adv. Energy Mater. 6:1502175. doi: 10.1002/aenm.201502175
Keywords: carbon fabric, TiO2 nanotube arrays, α-Fe2O3 hollow nanospheres, lithium-ion batteries, anodes
Citation: Xu P, Zhang Z, Zhang H, Shen A, Zhao Y, Zhou Y and Weng Y (2020) Binder-Free Charantia-Like Metal-Oxide Core/Shell Nanotube Arrays for High-Performance Lithium-Ion Anodes. Front. Chem. 8:159. doi: 10.3389/fchem.2020.00159
Received: 03 December 2019; Accepted: 21 February 2020;
Published: 06 March 2020.
Edited by:
James Rohan, University College Cork, IrelandReviewed by:
Maksudul Hasan, Newcastle University, United KingdomXinhui Xia, Zhejiang University, China
Copyright © 2020 Xu, Zhang, Zhang, Shen, Zhao, Zhou and Weng. This is an open-access article distributed under the terms of the Creative Commons Attribution License (CC BY). The use, distribution or reproduction in other forums is permitted, provided the original author(s) and the copyright owner(s) are credited and that the original publication in this journal is cited, in accordance with accepted academic practice. No use, distribution or reproduction is permitted which does not comply with these terms.
*Correspondence: Ziying Zhang, enp5aW5nJiN4MDAwNDA7c3Vlcy5lZHUuY24=