- College of Chemistry and Environmental Engineering, Yangtze University, Jingzhou, China
Tin and tin compounds are perceived as promising next-generation lithium (sodium)-ion batteries anodes because of their high theoretical capacity, low cost and proper working potentials. However, their practical applications are severely hampered by huge volume changes during Li+ (Na+) insertion and extraction processes, which could lead to a vast irreversible capacity loss and short cycle life. The significance of morphology design and synergic effects-through combining compatible compounds and/or metals together-on electrochemical properties are analyzed to circumvent these problems. In this review, recent progress and understanding of tin and tin compounds used in lithium (sodium)-ion batteries have been summarized and related approaches to optimize electrochemical performance are also pointed out. Superiorities and intrinsic flaws of the above-mentioned materials that can affect electrochemical performance are discussed, aiming to provide a comprehensive understanding of tin and tin compounds in lithium(sodium)-ion batteries.
Introduction
Since the commercialization of lithium-ion batteries (LIBs) by the Sony Corporation in 1991, LIBs are widely used in portable devices, electric vehicles and energy storage equipment for their benefits of having no memory effect, long cycle life and high energy density (Tarascon and Armand, 2010; Kim et al., 2012; Wang et al., 2019). With largely depleting lithium resources, the existing limited and unevenly distributed lithium reserves cannot meet the increasing demands of LIBs (there is an estimated 17 ppm in the earth's crust; Grosjean et al., 2012). Due to abundant sodium reserves (there is an estimated 23,000 ppm in the earth's crust), sodium-based batteries can be an attractive alternative. Traditional Na-S batteries require operating temperatures between 300 and 350°C to allow sufficient Na+ conductivity of NaAl11O17, but safety issues and energy loss from maintaining the operating temperature are inevitable (Wen et al., 2008; Xin et al., 2014; Kou et al., 2019). Motived by the similar chemical properties of sodium and lithium, researchers have shifted their attention to ambient temperature sodium-ion batteries (SIBs), but lots of problems need to be addressed for the practical application of SIBs (Yabuuchi et al., 2014; Li et al., 2018; Wu L. et al., 2018; Liu Y. et al., 2019). The main issue is the larger radius size of Na+ (1.09 Å) compared with Li+ (0.74 Å), which brings about sluggish reaction kinetics with low capacity, poor rate capability, and short cycling life (Chevrier and Ceder, 2011; Xu et al., 2013; Li et al., 2018). Extensive studies have been carried out to understand the requirements of commercial SIBs, which are great choices for low cost and large-scale energy storage equipment required for intermittent renewable energy and smart grids (Palomares et al., 2012; Pan et al., 2013). Comparitively, the energy density of LIBs cannot fully satisfy an increasingly growing need for electronic energy storage devices (Xiao et al., 2018; Fang et al., 2020). The present conventional anode in LIBs is graphite, which follows a intercalation/de-intercalation reaction pathway with a low theoretical capacity (378 mAh/g) and is electrochemically unfavorable for SIBs owing to the larger size of Na+ (Qian et al., 2014). Therefore, not all successful experiences from LIBs are viable to be applied in SIBs. Usually, graphene and non-graphitic carbon (like hard carbon and carbon black) are conventional anodes in SIBs. Additionally, TiO2, Na2Ti3O7, Sn, SnO2, SnS2, Sb, and P, etc. are potential anode materials for Na+ storage in SIB systems (Slater et al., 2013; Li et al., 2018; Guan et al., 2020). Thanks to a similar charging-discharging mechanism, tin-based anodes' alloying/dealloying reactions have attracted considerable attention because they are applicable to both LIBs and SIBs with a high theoretical capacity (Stevens and Dahn, 2000; Zhu et al., 2013). Environmental benignity, low costs, and lower operating potentials than graphite are also attractive features for tin and tin compounds, but they contain the following intrinsic defects (Fu et al., 2016). Tin and tin compounds as anodes in LIBs (SIBs) sustain colossal volume changes during Li+ (Na+) insertion and extraction processes, which leads to pulverization of the active materials as well as losing electrical contact with the collector (Zhang, 2011; Liu D. et al., 2019). Moreover, a continuously regenerated solid electrolyte interphase (SEI) layer between the electrode and electrolyte interface will consume extra lithium (sodium) ions, causing large irreversible capacity loss and poor cycle stability (Beaulieu et al., 2001). Last but not least, the electronic conductivity of SnO2 (0.1 S/m) and SnS2 (1 S/m) is much inferior to Sn (9.1 × 106 S/m) (Thangaraju and Kaliannan, 2000; Saadeddin et al., 2006; Nie et al., 2020). To cope with these problems, many measures have been taken and summarized as follows.
Firstly, according to comprehensive investigations nano-scale tin and tin compounds can alleviate the inter stress brought on by volume changes, to some extent, and can shorten the transfer paths of lithium (sodium) ions and electrons. Additionally, more reactive sites on the interface between electrodes and electrolytes are generated (Uchiyama et al., 2008; Park and Park, 2015; Park et al., 2018). The second method is to incorporate tin and tin compounds with one or more stress-accommodating phases that have can assure electronic conductivity, such as carbonaceous materials, metals and some transitional metal compounds (Kepler et al., 1999; Takamura et al., 1999). In 2005, Sony commercialized the first tin-based amorphous anode with the trademark “Nexelion” and this anode is composed of Sn, Co and C, where Co and C are identified as conductive and stress-releasing phases. According to Sony, Nexelion has a capacity of 900 mAh, which is 28 % higher than conventional graphite (700 mAh) at 0.2°C. Extensive investigations have been made to find a feasible and low-cost way to synthesize tin- and tin compound-based anodes with satisfactory physicochemical and electrochemical properties for both LIBs and SIBs at the same time. In this review, we focus on the recent progress of Sn, SnO2, and SnS2 as anodes in LIBs and SIBs. This comprehensive review provides an in-depth account of the similarities and differences between Sn, SnO2, and SnS2 as used in LIBs (SIBs) as well as clear directions for the structure design and fabrication procedures regarding anode material syntheses in LIBs and SIBs.
Tin and Tin Compounds in LIBs
Sn-Based Composites
Sn has a high theoretical specific capacity of 993.4 mAh/g, according to the reversible reaction Sn+xLi++xe−↔LixSn (0≤x≤4.4) (Lee et al., 2003). However, huge volume changes and aggregation of Sn particles during the alloying/dealloying process are the main obstacles for practical applications (Beaulieu et al., 2001). Generally, carbonaceous materials and Sn-based intermetallics are believed to address these issues efficiently and largely improve the battery performance of Sn-based anode materials (Zhao et al., 2015; Ying and Han, 2017). Carbon materials, either acting as the support or coating, can effectively ease volume changes and aggregation of Sn particles and increase the overall conductivity, especially with graphene (Wen et al., 2016). Zhou et al. have reported a high-performance anode where tin nanoparticles are impregnated into nitrogen-doped graphene (Zhou et al., 2013a). The graphene coating can facilitate electron transport and prevent aggregation of tin particles. Add void spaces between graphene and tin nanoparticles avail the accommodation of volume changes. As a result, the final composite delivers a reversible capacity of 481 mAh/g at a current density of 100 mA/g.
Some Sn-based intermetallics have also been considered as a promising choice, such as Sn-Cu, Sn-Co, Sn-Sb, Sn-Bi, Sn-Se, Sn-Fe and Sn-Ni etc (Yang et al., 1999; Yoon et al., 2009; Xue et al., 2010; Dang et al., 2015; Qin et al., 2017). Among all these types of intermetallics, Sony's Nexelion-consisting of Sn, Co, and C-is the first commercialized tin-based anode, but the composition is not fully revealed. Hence, it is important to further investigate the role and mechanism of cobalt in the Sn-Co intermetallic system. In principle, cobalt is considered an inactive component used to buffer the volume changes. However, according to the systematic study of Sn1−xCox (0<x<0.6) and [Sn0.55Co0.45]1−yCy (0<y<0.5) conducted by Dahn et al., the Sn1−xCox system is amorphous when 0.28 <x<0.43 and an amorphous structure can hold part of the capacity in place of alloying anodes in LIBs. In addition, cobalt does not form intermetallic Co-carbides which avoids the exclusion of crystalline tin, improving the cycle stability of the composite (Tamura et al., 2004; Dahn et al., 2006; Todd et al., 2007; Li et al., 2011).
Sn-Cu alloy is another extensively explored anode in LIBs, especially in the stable Cu6Sn5 intermetallic phase. According to the detailed in-situ X-ray study of Cu6Sn5 by Larcher and his coworker, the two reverse phase transitions of Cu6Sn5 reacting with Li+ are listed as follows (Larcher et al., 2000):
As the Cu content in the Cu-Sn alloy increases, the final obtained product will significantly improve in cyclability, because Cu is used as an inactive buffering matrix to relieve the volume expansion. However it also results in a relatively lower discharge capacity, for example, the theoretical discharge specific capacity of Cu6Sn5 in LIBs is 584 mAh/g (Trahey et al., 2009). Core/shell Cu6Sn5@SnO2-C anode materials are prepared by boiling Sn and Cu powders in a sucrose solution with air, as reported by Hu's group, in which Cu6Sn5 as an inert foundation replaces the electrochemically inactive Cu, SiC and Ni (Hu et al., 2015). As a consequence, the composite exhibits a high discharge specific capacity of 619 mAh/g at 1.0°C after 500 cycles, and SEM images before and after the first cycle show that the maximum volume change ratio decreases to 12.7%.
On the other hand, some Sn-based intermetallics with electrochemically active metals, like Sb, Bi, and Ge, have shown higher initial capacities and better electrochemical properties than the individual active materials, which is due to the different potentials vs. Li+/Li of these active metals. The temporarily separated charge-discharge process of these active materials guarantees that Sn and the electrochemically active metals can operate as volume-releasing phases for each other alternately (Trifonova et al., 2002; Zhang, 2011). He and his co-workers have reported a colloidal synthesis of monodisperse SnSb nanocrystals that deliver high specific capacities of 700 and 600 mAh/g at 0.5 and 4.0°C after 100 cycles, respectively (He et al., 2015).
Graphene with its excellent electrical conductivity, flexibility, and high specific surface area can be an ideal buffering matrix for tin-based anodes (Li and Kaner, 2008). In 2015, Luo et al. synthesized a novel anode where tin nanoparticles were encapsulated into graphene backboned carbon foam (Luo B. et al., 2016). Graphene and the outermost carbon coating serve as a physical boundary to prevent the aggregation of well-distributed tin nanoparticles and alleviate the huge volume changes of tin particles. The unique structure is prepared by uniformly growing SnO2 on the surface of graphene oxide and coating with porous carbon through a hydrothermal processes, finally calcinating in a reducing atmosphere. The resulting composite shows excellent cycle stability and exceptional rate performance in LIBs as well as in SIBs. A reversible specific capacity of 506 mAh g−1 can be achieved at a current density of 400 mAh/g and retained at 270 mAh/g, and even at 3,200 mA/g after 500 cycles (Figure 1). A summary of anode materials, synthetic methods, and electrochemical performance in tin-based anode composites is shown in Table 1 for comparison.
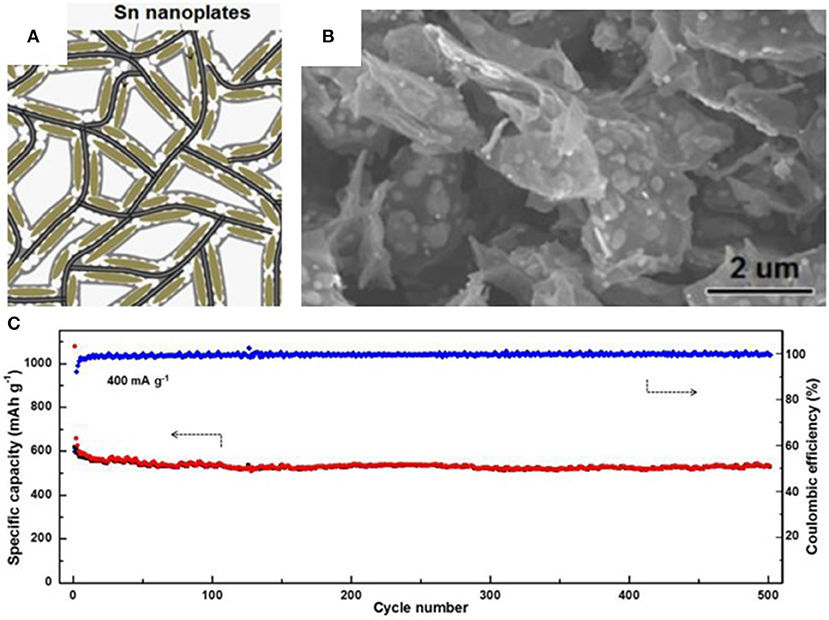
Figure 1. Schematic illustration (A) and SEM image (B) of tin nanoplates encapsulated in foam like graphene backboned carbonaceous carbon matrix (F-G/Sn@C), cycling performance (C) of F-G/Sn@C at 400 mA/g from 0.01 to 2.00 V. Reproduced from Luo B. et al. (2016) with permission from Copyright (2016) Elsevier.
SnO2-Based Composites
Tin oxide materials were first discovered and applied in LIBs with a high specific capacity by Idato et al. from Fuji Photo Film in 1997 (Idota et al., 1997). From then on, SnO2-based anodes in LIBs have drawn considerable attention because of their high theoretical capacity, resource availability, environmental benignity, and low operating potentials (0.3 and 0.5 V vs. Li+/Li in charge and discharge processes; Li R. et al., 2019). The chemical reactions of SnO2 with lithium electrodes involve the following two steps (Courtney and Dahn, 1997; Chen and Lou, 2013; Zhou et al., 2013b):
The theoretical specific capacity for bulk SnO2 electrodes is 780 mAh/g, which includes conversion reactions and further alloying/dealloying reactions. It is worth noting that the conversion reactions of bulk SnO2 to Sn are irreversible but can be partly reversible for nanosized SnO2 and the theoretical specific capacity can be up to 1,484 mAh/g (Kim et al., 2005; Zhang et al., 2009). Like Sn, the as-formed Sn from SnO2 suffers from huge volume changes (250%) in alloying/dealloying processes and what's worse, the inner stress originating from volume changes causes pulverization of the SnO2 electrodes. The conversion reaction and pulverization of the SnO2 electrode brings about a severe capacity decrease in the SnO2. Another issue that needs to be mentioned is that the Sn particles from conversion reactions tend to agglomerate into Sn clusters that will weaken the electrochemical activity (Park et al., 2007; Deng et al., 2016). These flaws are the main limitations for the commercialization of SnO2-based anodes in LIBs.
To deal with the defects of SnO2-based electrodes, the adopted strategies are summarized as follows. The first strategy is to convert bulk SnO2 particles into nanosized particles and simultaneously design nanostructures such as nanospheres, nanotubes, and nanofilms (Liu et al., 2016). The nanostructures can accommodate volume changes and shorten the diffusion length for electrons and lithium ions, but the accompanying negative effect for nanostructure materials is that the high surface energy will lead to the agglomeration of nanoparticles, which is electrochemically unfavorable (Chen and Lou, 2013). Additionally, structure design alone cannot compensate for the whole volume change whilst producing the desired electrochemical performance. Hence, another strategy is proposed, which is to combine the designed architecture with carbonaceous materials including carbon nanotubes, amorphous carbon, hard carbon, and graphene (Read et al., 2001; Yang et al., 2013; Zhou et al., 2016). Carbonaceous materials not only prevent nano SnO2 and as-formed Sn grains from agglomeration by creating a physical barrier, but they also improve the overall electronic conductivity of the SnO2-based composite.
When it comes to size control of SnO2 in LIBs, it is not found that as the SnO2 particles get smaller, the better the electrochemical performance becomes. As the size of SnO2 particles decreases, the SEI layer becomes larger, which hinders SnO2 from reacting with lithium ions (Kim et al., 2013). According to Ahn et al., the optimum size of colloidal synthesis of SnO2 particles is ~11 nm during Li+ insertion/extraction processes (Ahn et al., 2004). A series of sizes of SnO2 hollow spheres as investigated by Kim et al. demonstrated that SnO2 hollow spheres with a size of 25 nm showed the best electrochemical performance (750 mAh/g after 50 cycles at a current density of 100 mA/g; Kim et al., 2013). Moreover, SnO2 nanoparticles synthesized via the hydrothermal method with a size of 3 nm deliver the best reversible capacity (740 mAh/g after 60 cycles at 1,800 mA/g) compared to the ones at 4 and 8 nm (Kim et al., 2005). As a consequence, the optimum size for SnO2 nanoparticles varies for different fabrication processes.
Recently, Jiang et al. have shown that well-designed cob-like SnO2 nanoparticles coated with polydopamine and prepared by a hydrothermal processes exhibit an excellent rate capability and a long cycle life at around 1,400 mAh/g at a current density of 160 mA/g after 300 cycles (Jiang B. et al., 2017). Bush-like hydroxypropyl cellulose-graft-poly(acrylic acid) (HPC-g-PAA) and Na2SnO3·3H2O were used as the template and SnO2 precursor, respectively. SnO2 particles with an average size of 5 nm were uniformly grown on the graft of HPC-g-PAA template, and gaps of 3–5 nm among SnO2 particles could be observed, which allowed it to accommodate for volume changes of SnO2 particles in the electrode. Moreover, the final carbonized polydopamine coating was shown to help form stable SEI layers, which is helpful to enhance the cycle stability (Figure 2).
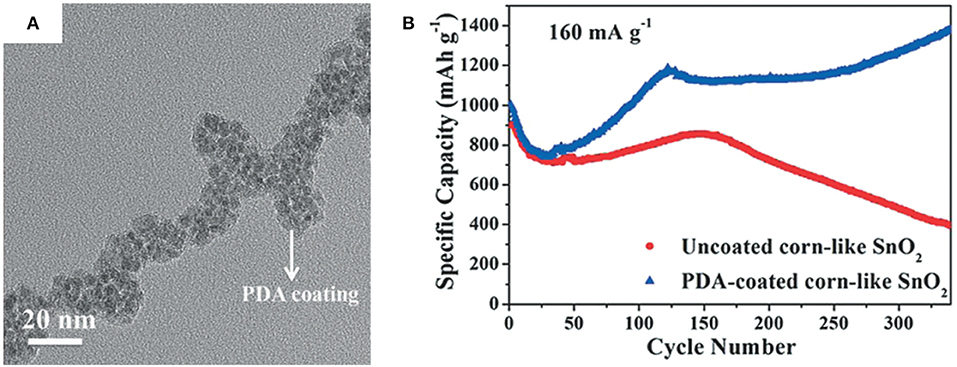
Figure 2. TEM image (A) of PDA-coated SnO2 and cycling performance (B) of PDA-coated corn-like SnO2 and uncoated corn-like SnO2 at 160 mA/g. Reproduced from Jiang B. et al. (2017) with permission from Copyright (2017) WILEY-VCH.
Beyond the use of carbon, transition metal compounds are also regarded as an effective component to be introduced into SnO2 electrodes with syngeneic effects of combined materials. TiO2, for example, is a very stable LIB anode material because of its outstanding electrochemical stability with only a slight volume change (3–4%) even in a high current density (Wang et al., 2012). However, TiO2 is restricted by a low theoretical capacity (178 mAh/g), so TiO2 is often used as a supporting backbone or a protective layer for unstable active materials like SnO2 (Liu H. et al., 2015). Tian et al. have proposed a well-designed nanostructure where SnO2 particles are encapsulated in TiO2 hollow nanowires (Tian et al., 2014). The composite employs SnO2 embedded carbon nanowires as a template after being coated with TiO2 and calcinated in air. Void spaces between SnO2 particles and TiO2 shells have been demonstrated through TEM analysis. The voids offer space to accommodate volume changes of SnO2 nanoparticles during the charge/discharge process. With this unique yolk-shell structure and the role of TiO2 in the composite, the final SnO2@TiO2 composite exhibits a great cycle stability (445 mAh/g at a current density of 800 mA/g after 500 cycles). A summary of anode materials, synthetic methods, and electrochemical performances upon some SnO2-based anodes are pointed out in Table 2.
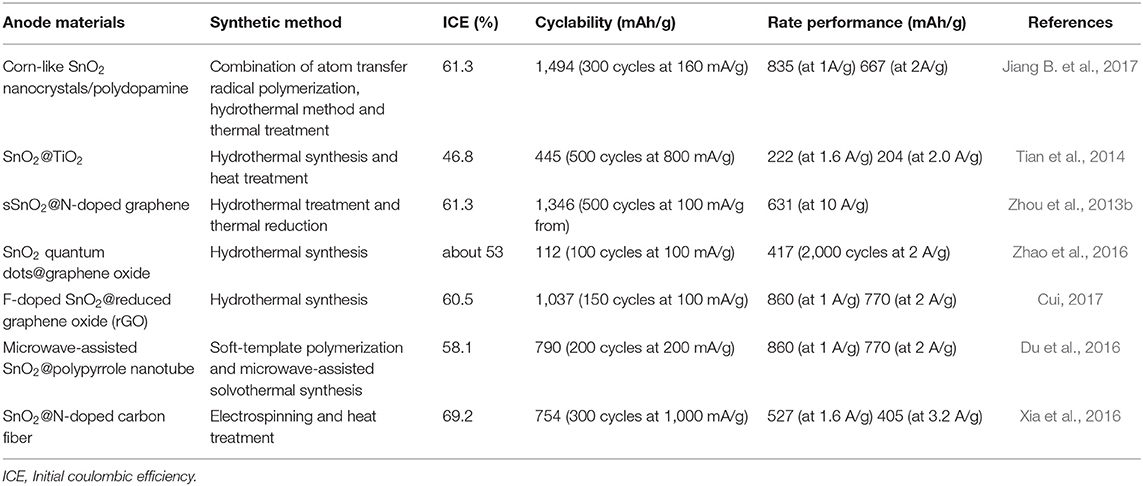
Table 2. Anode materials, synthetic methods and electrochemical performance of SnO2-based composites in LIBs.
SnS2-Based Composites
Momma et al. and Brousse et al. have revealed that tin sulfides could also be used as novel anode materials in LIBs (Brousse et al., 1998; Momma et al., 2001). SnS2 materials possess superior physicochemical properties with a theoretical specific capacity of 645 mAh/g and a unique layered hexagonal CdI2-type crystal structure that is composed of tin cations sandwiched between two layers of close-packed sulfur anions in octahedral coordination, in which adjacent sulfur layers are linked with weak Van der Waals interactions and the interlayer intervals are about 0.59 nm (Morales et al., 1992; Lefebvre et al., 1997; Song et al., 2013; Deng et al., 2014; Li R. et al., 2019). Layer voids in this unique configuration are beneficial for the Li+ insertion process and can partially accommodate the volume change (Chen et al., 2017). However, integral volume changes and poor electronic conductivity of SnS2 are inevitable, which needs to be improved and one set of adopted electrochemical reactions have been put forward, which are the following (Momma et al., 2001; Kim et al., 2007):
It can be obviously observed from the above equations that the reaction mechanism of SnS2 with lithium is very similar to the lithiation and delithiation of SnO2. In the first discharge cycle, metallic tin and amorphous Li2S are formed during the irreversible conversion of SnS2, where active Sn can be coated with the inactive Li2S, mitigating the volume changes of the electrode to some extent (Kim et al., 2009). With further charge and discharge processes, alloying/dealloying reactions of tin with lithium ions are reversible, but the capacity reduces rapidly due to the irreversible conversion and severe pulverization of SnS2 electrodes. Analogously, morphology design and the introduction of a conductive phase that accommodates volume changes, like amorphous carbon and graphene, can largely alleviate the volume changes of SnS2 in charge and discharge processes (Zhuo et al., 2012).
Since the microstructure of layered SnS2 materials has some resemblance to 2D graphene, the combination of them is more compatible than other dissimilar materials like SnO2, Sn, and Si (Bin et al., 2019). Few-layer SnS2/graphene hybrid materials synthesized using L-cysteine as a ligand in the solution-phase method have been reported by Chang et al. which can deliver a reversible specific capacity of 920 mAh/g at a current density of 100 mA/g (Chang et al., 2012). Additionally, graphene can be functionalized by doping with nitrogen, fluorine, or sulfur elements, and the doped graphene generates more defects and active sites which significantly enhances the electrochemical activity and conductivity (Guo et al., 2011). Zheng et al. have reported a large-scale and facile synthetic route for SnS2 nanoparticles coated with S-doped graphene (SnS2/S-rGO). The electrochemical stability of SnS2/S-rGO particles is much better than that of the undoped SnS2/rGO, in which the SnS2/S-rGO can possess a discharge specific capacity of 947 mAh/g whereas the SnS2/rGO is about 700 mAh/g after 200 cycles at 1A/g (Zheng et al., 2017). This result can be mainly ascribed to the stronger interaction of S-doped graphene with SnS2 particles.
Wu et al. have presented a well-designed stable H-TiO2@SnS2@PPy composite by growing SnS2 sheets on hydrogen treated TiO2 (H-TiO2) nanowires and coating with carbonized polypyrrole (PPy), in which H-TiO2 provides some advantages over untreated TiO2. The key reason is that H-TiO2 structurally possesses more defects than the untreated TiO2, which provides increased conductivity and stronger chemical interactions with SnS2 (Ti-S) (Wu et al., 2019). Furthermore, the outermost carbonized PPy layer can accommodate the volume change to some degree as well as boosting the electronic conductivity. With the synergistic effects of the mentioned materials, the final H-TiO2@SnS2@PPy composite can deliver an outstanding electrochemical stability with a high discharge specific capacity of 508.7 mAh/g at 2.0 A/g after 2,000 cycles (Figure 3). A summary of anode materials, synthetic methods, and electrochemical performance of SnS2-based composites in LIBs have been displayed in Table 3.
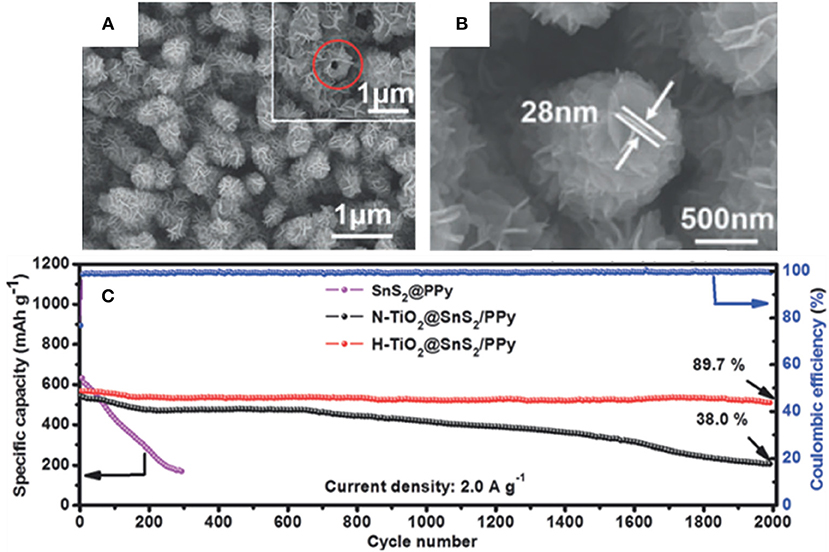
Figure 3. SEM images of H-TiO2@SnS2 (A) and H-TiO2@SnS2@PPy (B), cycling performance (C) of SnS2@PPy, H-TiO2@SnS2@PPy, and N-TiO2@SnS2@PPy at 2.0 A/g. Reproduced from Wu et al. (2019) with permission from Copyright (2019) WILEY-VCH.
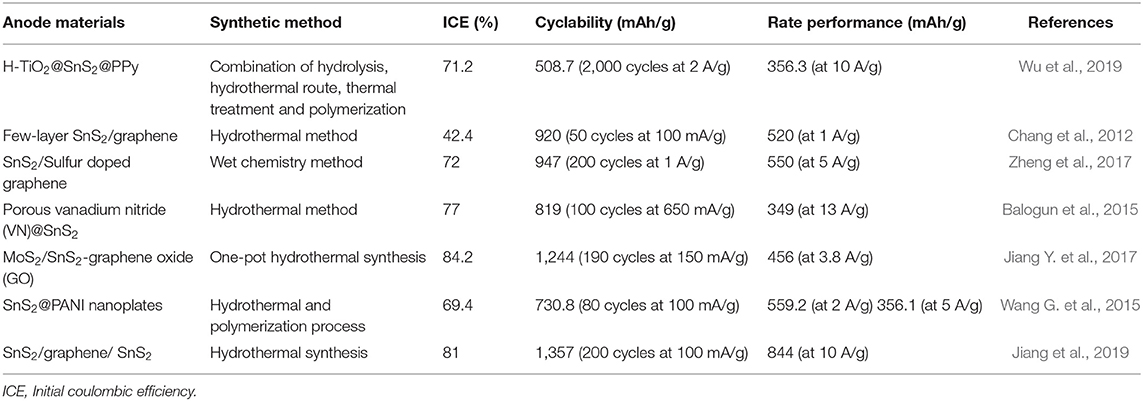
Table 3. Anode materials, synthetic methods and electrochemical performance of SnS2-based composites in LIBs.
Tin and Tin Compounds in SIBs
The revival of sodium-ion batteries (SIBs) owes mainly to the low cost and abundance of sodium on earth. Although the intercalation mechanism of sodium and lithium are similar when used as electrodes in secondary alkali metal batteries, the larger radius size of Na+ (1.09 Å) compared to Li+ (0.74 Å) makes it challenging to find a suitable Na+ host with both excellent cycle stability and a relatively high capacity (Luo W. et al., 2016; Wu L. et al., 2018). Graphite is the most used anode in commercial LIBs but cannot insert Na+ effectively, which is due to the mismatching of graphite's interlayer interval (0.334 nm) with the larger radius of Na+ (Chevrier and Ceder, 2011). Moreover, Si is a very promising anode material for LIBs as it has a theoretical discharge specific capacity of 3,579 mAh/g, and some Si-based materials have been commercialized, but it cannot react with Na+ in the same manner as LIBs. This is because Na-induced lattice disturbance are remarkable in Si materials as they become endowed with small interstitial space and high stiffness (Chou et al., 2015; Fang et al., 2019). Interestingly, Sn, SnO2, and SnS2 can be applied in SIBs with relatively high capacity, low cost, and proper low charge/discharge potentials vs. Na/Na+, due to the minor Na-induced lattice disturbance in Sn-based materials (Guo et al., 2011; Zhu et al., 2013; Li et al., 2015). However, these active materials still go through huge volume changes, and the volume change is even severer in SIBs, which leads to serious pulverization of these brittle active materials ending up with rapid capacity decay and poor cycle stability (Ellis et al., 2012). The coping strategies of Sn, SnO2, and SnS2 in SIBs are analogous to ones in LIBs, which are the nanostructure design of these active materials and the process of simultaneously introducing a second phase that buffers the volume change (Nayak et al., 2018). Major improvements for Sn, SnO2, and SnS2 in SIBs have been separately detailed in the following sections and the anode materials, synthetic methods and electrochemical performance of Sn, SnO2, SnS2-based anode composites in SIBs are summarized in Table 4.
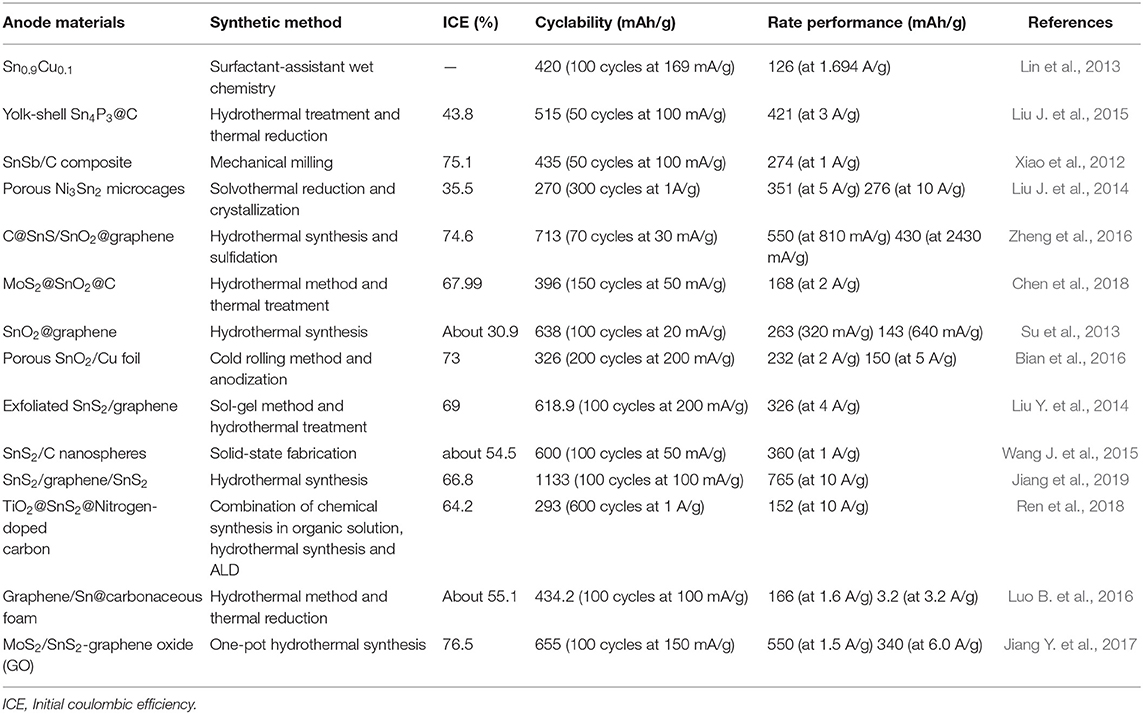
Table 4. Anode materials, synthetic methods and electrochemical performance of Sn, SnO2, and SnS2-based composite anodes in SIBs.
Sn-Based Composites
The theoretical capacity of Sn as anode materials in SIBs (Na15Sn4) is about 847 mAh/g, but volume changes of Sn electrodes during charge-discharge processes are as high as 525 %, which is much higher than Sn in LIBs (Qian et al., 2014). As reported by Qian et al., the capacity of pure Sn electrodes in SIBs falls to zero in only five cycles, which can be explained by the pulverization of active materials during Na+ insertion/extraction processes (Ellis et al., 2013). Sn-based intermetallic alloy anodes have been demonstrated to be a reasonable solution to address the short cycle life of Sn (Li J. et al., 2019). A Sn-Cu alloy is a stable active/inactive alloy with a relatively high capacity in LIBs where the addition of Cu significantly increases the stability of the alloy. As mentioned in the LIBs section, the Cu6Sn5 alloy is more stable than other Sn-Cu intermetallics, but the application of Cu6Sn5 in SIBs is hampered by the short diffusion depth owing to the larger size of Na+. Regarding this, Lin et al. have reported using a Sn0.9Cu0.1 alloy in SIBs (Lin et al., 2013). In spite of a low initial discharge specific capacity of 250 mAh/g, the capacity gradually increased to 440 mAh/g in 20 cycles without capacity loss after 100 cycles.
Sn-P intermetal is an emerging SIB anode material with balanced properties (Luo W. et al., 2016). Although the theoretical specific capacity of Sn4P3 (1,132 mAh/g) is significantly inferior to the pure P (2,560 mAh/g), electronic conductivity and theoretical volumetric capacity are much better than pure P in SIBs (Kim et al., 2014; Lan et al., 2017). Liu et al. have synthesized uniform yolk-shell Sn4P3@C nanoparticles for SIBs where Sn4P3 nanoparticles are encapsulated in hollow carbon spheres rendering some void space for the volume change of Sn4P3 whilst maintaining an intact microstructure (Liu J. et al., 2015). The carbon shell helps to form a stable SEI layer and strengthen the overall electronic conductivity of the composite. An initial discharge specific capacity of 790 mAh/g for yolk-shell Sn4P3@C nanospheres was determined and retained a high reversible specific capacity of 515 mAh/g after 50 cycles at 100 mA/g (Figure 4).
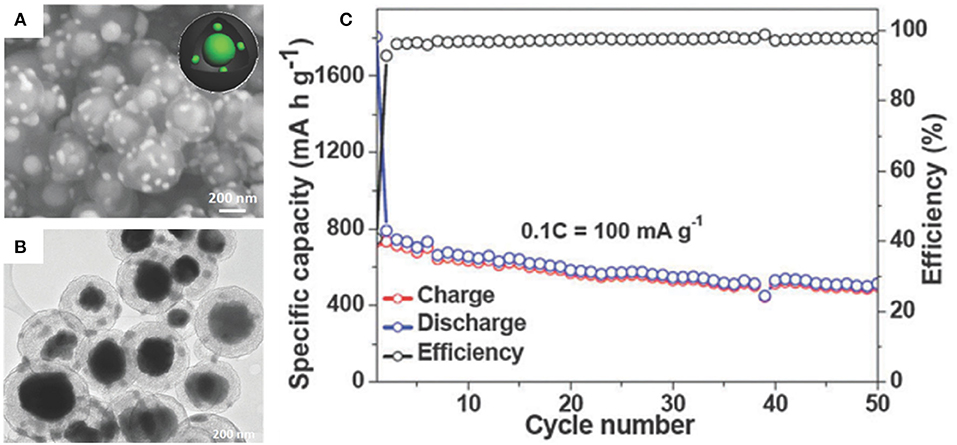
Figure 4. SEM (A) and TEM images (B) of yolk-shell Sn4P3@C. Cycling performance (C) of yolk-shell Sn4P3@C at 100 mA/g. Reproduced from Liu J. et al. (2015) with permission from Copyright (2015) Royal Society of Chemistry.
SnO2-Based Composites
Sodiation/desodiation reactions of the SnO2 electrode are very similar to the lithiation/delithiation process, which include the conversion of SnO2 and reversible alloying/dealloying reactions contributing to the total theoretical specific capacity of 1,378 mAh/g (Su et al., 2013). SnO2 is one of the most extensively investigated anode materials in LIBs and nowadays, some of the SnO2-based composites have reached the theoretical capacity of SnO2 with an excellent cycle life. Herein, successful strategies in LIBs to address volume changes are advised for employment in SIBs as well (Chen and Lou, 2013).
Huang et al. have reported a facile in situ synthesis of 3D porous carbon encapsulated SnO2 nanoparticles (SnO2-PC) that exhibits a great cycle stability with a discharge specific capacity of 208.1 mAh/g at 100 mA/g after 250 cycles and SnO2-PC with a SnO2 weight percentage of 74.47 % demonstrated an extraordinary rate capability with a discharge specific capacity of 100 mAh/g at 1,600 mA/g after 1,000 cycles (Huang et al., 2016). The greatly improved electrochemical performance of the as-prepared SnO2-PC composite owes to the porous carbon matrix that can alleviate volume changes of SnO2 in the sodiation/desodiation process and improve the electronic conductivity of the composite.
Heterostructure has the advantage of high-speed electron transfer because of the interface effect. The heterojunction of nanocrystals with different band-gaps has been proven to enhance surface reaction kinetics and to provide increased charge transport. Zheng et al. have employed SnS in a C@SnO2@graphene composite in SIBs. The C@SnS/SnO2@graphene composite exhibits a high rate capability and long cycle life with a high capacity, which can be ascribed to the heterostructure of SnS/SnO2 which further improves the electronic conductivity and diffusion of Na+ in the electrode (Zheng et al., 2016). C@SnS/SnO2@graphene achieves a reversible discharge specific capacity of 713 mAh/g at 30 mA/g after 70 cycles, which is higher than C@SnS@graphene (around 600 mAh/g) and C@SnO2@graphene (around 400 mAh/g). By increasing the current density to 810 and 2,430 mA/g, the discharge specific capacity can be retained at 520 and 430 mAh/g, respectively (Figure 5).
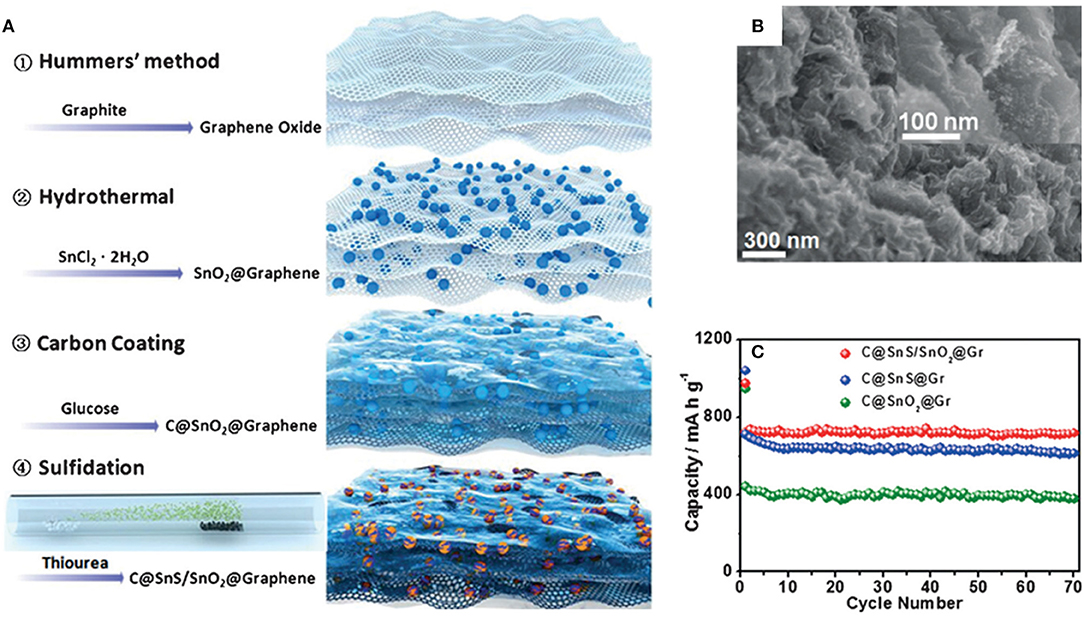
Figure 5. Schematic illustration (A) of the synthetic procedure of C@SnS/SnO2@graphene. SEM image (B) of C@SnS/SnO2@graphene. Cycling performance (C) of C@SnS/SnO2@graphene, C@SnS@graphene and C@SnO2@graphene at 30 mA/g. Reproduced from Zheng et al. (2016) with permission from Copyright (2016) WILEY-VCH.
SnS2-Based Composites in SIBs
As mentioned, SnS2 has a special layered structure where tin cations are sandwiched between two layers of sulfur anions. The spacing between two adjoining two layers (d002 = 5.90 Å) is larger than the radius of Na+ (d002 = 1.09 Å), which allows the intercalation and diffusion of Na+ throughout the electrode effectively (Zheng et al., 2016). However, a pure SnS2 electrode contends with poor conductivity and severe pulverization. It has been demonstrated from previous studies that combining SnS2 with conductive materials will notably strengthen the electrochemical performance (Ren et al., 2017; Wu Y. et al., 2018). The unique 2D layer structure of SnS2 means it is highly compatible with graphene and can provide an increase in electronic conductivity. In 2014, Liu et al. discovered that exfoliated SnS2 restacked on graphene showed a remarkable electrochemical performance with a discharge specific capacity of 650 mAh/g at 200 mA/g after 100 cycles (Liu Y. et al., 2014). The excellent performance can be ascribed to the ultrasmall exfoliated-SnS2 layers being utilized fully when used as the electrode.
Jiang et al. have reported a sandwich-like SnS2/graphene/SnS2 composite with expanded interlayers produced by a one-step hydrothermal synthesis, where both sides of the reduced graphene oxide sheets is covalently decorated with ultrathin SnS2 nanosheets (Jiang et al., 2019). The enlarged interlayer distance of SnS2 is about 8.03 Å, which assists the insertion/extraction of Li+/Na+ with rapid transport kinetics. As a result, SnS2/graphene/SnS2 composites have excellent electrochemical properties both in LIBs (see also in the LIBs section) and SIBs. To be specific for SIBs, the reversible discharge specific capacities of 1,295 mAh/g and 765 mAh/g are delivered at a current density of 0.1 and 10 A/g, respectively (Figure 6). Additionally, according to the structural characterizations of SnS2/graphene/SnS2 electrodes after 200 cycles, morphology changes and significant particle agglomeration cannot be clearly detected. Some reasons for the superiority of the SnS2/graphene/SnS2 composite are that the graphene sheet is sandwiched between SnS2 layers with enhanced conductivity and it has a strong structural integrity.
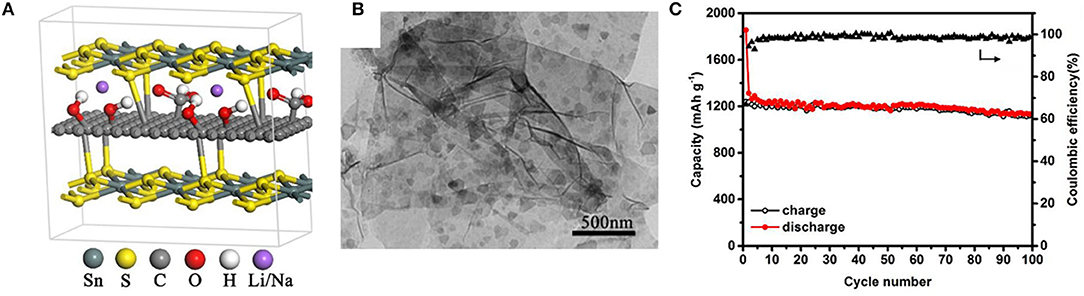
Figure 6. Molecular model (A) of sandwich like SnS2/graphene/SnS2; TEM image (B) of SnS2/graphene/SnS2; Cycling performance (C) of SnS2/graphene/SnS2 at 100 mA/g. Reproduced from Jiang et al. (2019) with permission from Copyright (2019) American Chemical Society.
Summary and Outlook
Sn, SnO2, and SnS2 have been extensively studied as substitutes for graphite in LIBs and for potential application in SIBs. Either in LIBs or SIBs, the ultimate problem that needs to be addressed is the huge volume change of Sn with Li+ or Na+ during the alloying/dealloying processes. This problem has been largely addressed by introducing one or more metals and/or compounds into the system and at least one additive which can act as an inactive buffering matrix. Also, the use of reasonable nanostructure design can tactfully mitigate the volume change and facilitate the diffusion of Li+ (Na+) and electrons. Due to these efforts, some of these tin-based anode materials have reached their maximum theoretical capacity. So far, the real practical uses of tin-based anodes is still very scarce in both LIBs and SIBs, which is mainly due to the tedious synthetic procedures, high costs and low yields. Recently, much work has focused on large-scale synthetic methods. We believe that a cost-effective and facile fabrication process which takes morphology into consideration can promote the application of tin-based anodes in commercial LIBs and large-scale energy storage equipment in SIBs.
Author Contributions
HM and WX contributed conception and design of the study. CM, RL, and LY organized the database. HM wrote the first draft of the manuscript. WX revised the whole manuscript.
Funding
This work was financially supported by the National Natural Science Foundation of China (Nos. 51874046 and 51404038).
Conflict of Interest
The authors declare that the research was conducted in the absence of any commercial or financial relationships that could be construed as a potential conflict of interest.
References
Ahn, H.-J., Choi, H.-C., Park, K.-W., Kim, S.-B., and Sung, Y.-E. (2004). Investigation of the structural and electrochemical properties of size-controlled SnO2 nanoparticles. J. Phys. Chem. B. 108, 9815–9820. doi: 10.1021/jp035769n
Balogun, M.-S., Qiu, W., Jian, J., Huang, Y., Luo, Y., Yang, H., et al. (2015). Vanadium nitride nanowire supported SnS2 nanosheets with high reversible capacity as anode material for lithium ion batteries. ACS Appl. Mater. Inter. 7, 23205–23215. doi: 10.1021/acsami.5b07044
Beaulieu, L. Y., Eberman, K. W., Turner, R. L., Krause, L. J., and Dahn, J. R. (2001). Colossal reversible volume changes in lithium alloys. Electrochem. Solid-State Lett. 4, A137–A140. doi: 10.1149/1.1388178
Bian, H., Zhang, J., Yuen, M.-F., Kang, W., Zhan, Y., Yu, D. Y. W., et al. (2016). Anodic nanoporous SnO2 grown on Cu foils as superior binder-free Na-ion battery anodes. J. Power Sources 307, 634–640. doi: 10.1016/j.jpowsour.2015.12.118
Bin, D.-S., Duan, S.-Y., Lin, X.-J., Liu, L., Liu, Y., Xu, Y.-S., et al. (2019). Structural engineering of SnS2/graphene nanocomposite for high-performance K-ion battery anode. Nano Energy 60, 912–918. doi: 10.1016/j.nanoen.2019.04.032
Brousse, T., Lee, S. M., Pasquereau, L., Defives, D., and Schleich, D. M. (1998). Composite negative electrodes for lithium ion cells. Solid State Ion. 113–115, 51–56. doi: 10.1016/S0167-2738(98)00366-X
Chang, K., Wang, Z., Huang, G., Li, H., Chen, W., and Lee, J. Y. (2012). Few-layer SnS2/graphene hybrid with exceptional electrochemical performance as lithium-ion battery anode. J. Power Sources 201, 259–266. doi: 10.1016/j.jpowsour.2011.10.132
Chang, X., Wang, T., Liu, Z., Zheng, X., Zheng, J., and Li, X. (2017). Ultrafine Sn nanocrystals in a hierarchically porous N-doped carbon for lithium ion batteries. Nano Res. 10, 1950–1958. doi: 10.1007/s12274-016-1381-6
Chen, J. S., and Lou, X. W. (2013). SnO2-based nanomaterials: synthesis and application in lithium-ion batteries. Small 9, 1877–1893. doi: 10.1002/smll.201202601
Chen, Q., Lu, F., Xia, Y., Wang, H., and Kuang, X. (2017). Interlayer expansion of few-layered Mo-doped SnS2 nanosheets grown on carbon cloth with excellent lithium storage performance for lithium ion batteries. J. Mater. Chem. A 5, 4075–4083. doi: 10.1039/C7TA00236J
Chen, Z., Yin, D., and Zhang, M. (2018). Sandwich-like MoS2@SnO2@C with high capacity and stability for sodium/potassium ion batteries. Small 14:1703818. doi: 10.1002/smll.201870074
Chevrier, V. L., and Ceder, G. (2011). Challenges for Na-ion negative electrodes. J. Electrochem. Soc. 158, A1011–A1014. doi: 10.1149/1.3607983
Chou, C.-Y., Lee, M., and Hwang, G. S. (2015). A comparative first-principles study on sodiation of silicon, germanium, and tin for sodium-ion batteries. J. Phys. Chem. C 119, 14843–14850. doi: 10.1021/acs.jpcc.5b01099
Courtney, I. A., and Dahn, J. R. (1997). Electrochemical and in situ X-ray diffraction studies of the reaction of lithium with tin oxide composites. J. Electrochem. Soc. 144, 2045–2052. doi: 10.1149/1.1837740
Cui, D. (2017). Fluorine-doped SnO2 nanoparticles anchored on reduced graphene oxide as a high-performance lithium ion battery anode. J. Power Sources 362:20–26. doi: 10.1016/j.jpowsour.2017.07.024
Dahn, J. R., Mar, R. E., and Abouzeid, A. (2006). Combinatorial study of Sn1−xCox (0 < x < 0.6) and [Sn0.55Co0.45]1−yCy (0 < y < 0.5) alloy negative electrode materials for Li-ion batteries. J. Electrochem. Soc. 153, A361–A365. doi: 10.1149/1.2150160
Dang, H. X., Klavetter, K. C., Meyerson, M. L., Heller, A., and Mullins, C. B. (2015). Tin microparticles for a lithium ion battery anode with enhanced cycling stability and efficiency derived from Se-doping. J. Mater. Chem. A 3, 13500–13506. doi: 10.1039/C5TA02131F
Deng, Y., Fang, C., and Chen, G. (2016). The developments of SnO2/graphene nanocomposites as anode materials for high performance lithium ion batteries: a review. J. Power Sources 304, 81–101. doi: 10.1016/j.jpowsour.2015.11.017
Deng, Y., Luo, Z., Conrad, N. J., Liu, H., Gong, Y., Najmaei, S., et al. (2014). Black phosphorus-monolayer MoS2 van der Waals heterojunction p-n diode. ACS Nano 8, 8292–8299. doi: 10.1021/nn5027388
Du, X., Yang, T., Lin, J., Feng, T., Zhu, J., Lu, L., et al. (2016). Microwave-assisted synthesis of SnO2@polypyrrole nanotubes and their pyrolyzed composite as anode for lithium-ion batteries. ACS Appl. Mater. Interfaces. 8, 15598–15606. doi: 10.1021/acsami.6b03332
Ellis, L. D., Ferguson, P. P., and Obrovac, M. N. (2013). Sodium insertion into tin cobalt carbon active/inactive nanocomposite. J. Electrochem. Soc. 160, A869–A872. doi: 10.1149/2.103306jes
Ellis, L. D., Hatchard, T. D., and Obrovac, M. N. (2012). Reversible insertion of sodium in tin. J. Electrochem. Soc. 159, A1801–A1805. doi: 10.1149/2.037211jes
Fang, R., Miao, C., Mou, H., and Xiao, W. (2020). Facile synthesis of Si@TiO2@rGO composite with sandwich-like nanostructure as superior performance anodes for lithium ion batteries. J. Alloys Compd. 818:152884. doi: 10.1016/j.jallcom.2019.152884
Fang, R., Xiao, W., Miao, C., Mei, P., Zhang, Y., Yan, X., et al. (2019). Fabrication of Si-SiO2@Fe/NC composite from industrial waste AlSiFe powders as high stability anodes for lithium ion batteries. Electrochim. Acta 324:134860. doi: 10.1016/j.electacta.2019.134860
Fu, S., Ni, J., Xu, Y., Zhang, Q., and Li, L. (2016). Hydrogenation driven conductive Na2Ti3O7 nanoarrays as robust binder-free anodes for sodium-ion batteries. Nano Lett. 16, 4544–4551. doi: 10.1021/acs.nanolett.6b01805
Grosjean, C., Miranda, P. H., Perrin, M., and Poggi, P. (2012). Assessment of world lithium resources and consequences of their geographic distribution on the expected development of the electric vehicle industry. Renew. Sust. Energ. Rev. 16, 1735–1744. doi: 10.1016/j.rser.2011.11.023
Guan, P., Zhou, L., Yu, Z., Sun, Y., Liu, Y., Wu, F., et al. (2020). Recent progress of surface coating on cathode materials for high-performance lithium-ion batteries. J. Energy Chem. 43, 220–235. doi: 10.1016/j.jechem.2019.08.022
Guo, B., Liang, F., Zhang, B., and Gong, J. R. (2011). Graphene doping: a review. Insci. J. 1, 80–89. doi: 10.5640/insc.010280
He, M., Walter, M., Kravchyk, K. V., Erni, R., Widmer, R., and Kovalenko, M. V. (2015). Monodisperse SnSb nanocrystals for Li-ion and Na-ion battery anodes: synergy and dissonance between Sn and Sb. Nanoscale 7, 455–459. doi: 10.1039/C4NR05604C
Hu, R., Waller, G. H., Wang, Y., Chen, Y., Yang, C., Zhou, W., et al. (2015). Cu6Sn5@SnO2-C nanocomposite with stable core/shell structure as a high reversible anode for Li-ion batteries. Nano Energy 18, 232–244. doi: 10.1016/j.nanoen.2015.10.037
Huang, X., Cui, S., Chang, J., Hallac, P. B., Fell, C. R., Luo, Y., et al. (2015). A hierarchical tin/carbon composite as an anode for lithium-ion batteries with a long cycle life. Angewandte. Chemie. 127, 1510–1513. doi: 10.1002/ange.201409530
Huang, Z., Hou, H., Zou, G., Chen, J., Zhang, Y., Liao, H., et al. (2016). 3D porous carbon encapsulated SnO2 nanocomposite for ultrastable sodium ion batteries. Electrochimi. Acta 214, 156–164. doi: 10.1016/j.electacta.2016.08.040
Idota, Y., Kubota, T., Matsufuji, A., Maekawa, Y., and Miyasaka, T. (1997). Tin-based amorphous oxide: a high-capacity lithium-ion-storage material. Science 276, 1395–1397. doi: 10.1126/science.276.5317.1395
Jiang, B., He, Y., Li, B., Zhao, S., Wang, S., He, Y.-B., et al. (2017). Polymer-templated formation of polydopamine-coated SnO2 nanocrystals: anodes for cyclable lithium-ion batteries. Angew. Chem. Inter. Ed. 56, 1869–1872. doi: 10.1002/anie.201611160
Jiang, Y., Guo, Y., Lu, W., Feng, Z., Xi, B., Kai, S., et al. (2017). Rationally incorporated MoS2/SnS2 nanoparticles on graphene sheets for lithium-ion and sodium-ion batteries. ACS Appl. Mater. Interfaces 9, 27697–27706. doi: 10.1021/acsami.7b06572
Jiang, Y., Song, D., Wu, J., Wang, Z., Huang, S., Xu, Y., et al. (2019). Sandwich-like SnS2/graphene/SnS2 with expanded interlayer distance as high-rate lithium/sodium-ion battery anode materials. ACS Nano 13, 9100–9111. doi: 10.1021/acsnano.9b03330
Kepler, K. D., Vaughey, J. T., and Thackeray, M. M. (1999). Copper-tin anodes for rechargeable lithium batteries: an example of the matrix effect in an intermetallic system. J. Power Sources 81–82, 383–387. doi: 10.1016/S0378-7753(99)00111-1
Kim, C., Noh, M., Choi, M., Cho, J., and Park, B. (2005). Critical size of a nano SnO2 electrode for Li-secondary battery. Chem. Mater. 17, 3297–3301. doi: 10.1021/cm048003o
Kim, H. S., Chung, Y. H., Kang, S. H., and Sung, Y.-E. (2009). Electrochemical behavior of carbon-coated SnS2 for use as the anode in lithium-ion batteries. Electrochim. Acta 54, 3606–3610. doi: 10.1016/j.electacta.2009.01.030
Kim, T.-H., Park, J.-S., Chang, S. K., Choi, S., Ryu, J. H., and Song, H.-K. (2012). The current move of lithium ion batteries towards the next phase. Adv. Energy Mater. 2, 860–872. doi: 10.1002/aenm.201200028
Kim, T.-J., Kim, C., Son, D., Choi, M., and Park, B. (2007). Novel SnS2-nanosheet anodes for lithium-ion batteries. J. Power Sources 167, 529–535. doi: 10.1016/j.jpowsour.2007.02.040
Kim, W.-S., Hwa, Y., Jeun, J.-H., Sohn, H.-J., and Hong, S.-H. (2013). Synthesis of SnO2 nano hollow spheres and their size effects in lithium ion battery anode application. J. Power Sources 225, 108–112. doi: 10.1016/j.jpowsour.2012.10.030
Kim, Y., Kim, Y., Choi, A., Woo, S., Mok, D., Choi, N.-S., et al. (2014). Tin phosphide as a promising anode material for Na-ion batteries. Adv. Mater. 26, 4139–4144. doi: 10.1002/adma.201305638
Kou, Z., Miao, C., Mei, P., Zhang, Y., Yan, X., Jiang, Y., et al. (2019). Enhancing the cycling stability of all-solid-state lithium-ion batteries assembled with Li1.3Al0.3Ti1.7(PO4)3 solid electrolytes prepared from precursor solutions with appropriate pH values. Ceram. Int. doi: 10.1016/j.ceramint.2019.12.229
Lan, D., Wang, W., Shi, L., Huang, Y., Hu, L., and Li, Q. (2017). Phase pure Sn4P3 nanotops by solution-liquid-solid growth for anode application in sodium ion batteries. J. Mater. Chem. A 5, 5791–5796. doi: 10.1039/C6TA10685D
Larcher, D., Beaulieu, L. Y., Macneil, D. D., and Dahn, J. R. (2000). In situ X-ray study of the electrochemical reaction of Li with η′-Cu6Sn5. J. Electrochem. Soc. 147, 1658–1662. doi: 10.1149/1.1393413
Lee, K. T., Jung, Y. S., and Oh, S. M. (2003). Synthesis of tin-encapsulated spherical hollow carbon for anode material in lithium secondary batteries. J. Am. Chem. Soc. 125, 5652–5653. doi: 10.1021/ja0345524
Lefebvre, I., Lannoo, M., Moubtassim, M. E., Fourcade, J. O., and Jumas, J. C. (1997). Lithium insertion in three-dimensional tin sulfides. Chem. Mater. 9, 2805–2814. doi: 10.1021/cm970139h
Li, D., and Kaner, R. B. (2008). Graphene-based materials. Science 320, 1170–1171. doi: 10.1126/science.1158180
Li, J., Xu, X., Luo, Z., Zhang, C., Yu, X., Zuo, Y., et al. (2019). Compositionally tuned NixSn alloys as anode materials for lithium-ion and sodium-ion batteries with a high pseudocapacitive contribution. Electrochim. Acta 304, 246–254. doi: 10.1016/j.electacta.2019.02.098
Li, L., Zheng, Y., Zhang, S., Yang, J., Shao, Z., and Guo, Z. (2018). Recent progress on sodium ion batteries: potential high-performance anodes. Energy Environ. Sci. 11, 2310–2340. doi: 10.1039/C8EE01023D
Li, M.-Y., Liu, C.-L., Shi, M.-R., and Dong, W.-S. (2011). Nanostructure Sn-Co-C composite lithium ion battery electrode with unique stability and high electrochemical performance. Electrochim. Acta 56, 3023–3028. doi: 10.1016/j.electacta.2010.12.102
Li, R., Xiao, W., Miao, C., Fang, R., Wang, Z., and Zhang, M. (2019). Sphere-like SnO2/TiO2 composites as high-performance anodes for lithium ion batteries. Ceram. Int. 45, 13530–13535. doi: 10.1016/j.ceramint.2019.04.059
Li, Z., Ding, J., and Mitlin, D. (2015). Tin and tin compounds for sodium ion battery anodes: phase transformations and performance. Acc. Chem. Res. 48, 1657–1665. doi: 10.1021/acs.accounts.5b00114
Lin, Y.-M., Abel, P. R., Gupta, A., Goodenough, J. B., Heller, A., and Mullins, C. B. (2013). Sn-Cu nanocomposite anodes for rechargeable sodium-ion batteries. ACS Appl. Mater. Interfaces 5, 8273–8277. doi: 10.1021/am4023994
Liu, D., Fan, X., Li, Z., Liu, T., Sun, M., Qian, C., et al. (2019). A cation/anion co-doped Li1.12Na0.08Ni0.2Mn0.6O1.95F0.05 cathode for lithium ion batteries. Nano Energy 58, 786–796. doi: 10.1016/j.nanoen.2019.01.080
Liu, H., Li, W., Shen, D., Zhao, D., and Wang, G. (2015). Graphitic carbon conformal coating of mesoporous TiO2 hollow spheres for high-performance lithium ion battery anodes. J. Am. Chem. Soc. 137, 13161–13166. doi: 10.1021/jacs.5b08743
Liu, J., Kopold, P., Wu, C., Van Aken, P. A., Maier, J., and Yu, Y. (2015). Uniform yolk-shell Sn4P3@C nanospheres as high-capacity and cycle-stable anode materials for sodium-ion batteries. Energy Environ. Sci. 8, 3531–3538. doi: 10.1039/C5EE02074C
Liu, J., Wen, Y., Van Aken, P. A., Maier, J., and Yu, Y. (2014). Facile synthesis of highly porous Ni-Sn intermetallic microcages with excellent electrochemical performance for lithium and sodium storage. Nano Lett. 14, 6387–6392. doi: 10.1021/nl5028606
Liu, L., Xie, F., Lyu, J., Zhao, T., Li, T., and Choi, B. G. (2016). Tin-based anode materials with well-designed architectures for next-generation lithium-ion batteries. J. Power Sources 321, 11–35. doi: 10.1016/j.jpowsour.2016.04.105
Liu, Y., Fan, X., Zhang, Z., Wu, H.-H., Liu, D., Dou, A., et al. (2019). Enhanced electrochemical performance of Li-rich layered cathode materials by combined Cr doping and LiAlO2 coating. ACS Sustain. Chem. Eng. 7, 2225–2235. doi: 10.1021/acssuschemeng.8b04905
Liu, Y., Kang, H., Jiao, L., Chen, C., Cao, K., Wang, Y., et al. (2014). Exfoliated-SnS2 restacked on graphene as high-capacity, high-rate, and long-cycle life anode for sodium ion batteries. Nanoscale 7:1325–1332. doi: 10.1039/C4NR05106H
Luo, B., Qiu, T., Ye, D., Wang, L., and Zhi, L. (2016). Tin nanoparticles encapsulated in graphene backboned carbonaceous foams as high-performance anodes for lithium-ion and sodium-ion storage. Nano Energy 22, 232–240. doi: 10.1016/j.nanoen.2016.02.024
Luo, W., Shen, F., Bommier, C., Zhu, H., Ji, X., and Hu, L. (2016). Na-ion battery anodes: materials and electrochemistry. Acc. Chem. Res. 49, 231–240. doi: 10.1021/acs.accounts.5b00482
Momma, T., Shiraishi, N., Yoshizawa, A., Osaka, T., Gedanken, A., Zhu, J., et al. (2001). SnS2 anode for rechargeable lithium battery. J. Power Sources 97, 198–200. doi: 10.1016/S0378-7753(01)00723-6
Morales, J., Perez-Vicente, C., and Tirado, J. L. (1992). Chemical and electrochemical lithium intercalation and staging in 2H-SnS2. Solid State Ion. 51, 133–138. doi: 10.1016/0167-2738(92)90190-Z
Nayak, P. K., Yang, L., Brehm, W., and Adelhelm, P. (2018). From lithium-ion to sodium-ion batteries: advantages, challenges, and surprises. Angew. Chem. Int. Ed. 57, 102–120. doi: 10.1002/anie.201703772
Nie, Y., Xiao, W., Miao, C., Xu, M., and Wang, C. (2020). Effect of calcining oxygen pressure gradient on properties of LiNi0.8Co0.15Al0.05O2 cathode materials for lithium ion batteries. Electrochim. Acta 334:135654. doi: 10.1016/j.electacta.2020.135654
Palomares, V., Serras, P., Villaluenga, I., Hueso, K. B., Carretero-González, J., and Rojo, T. (2012). Na-ion batteries, recent advances and present challenges to become low cost energy storage systems. Energy Environ. Sci. 5, 5884–5901. doi: 10.1039/c2ee02781j
Pan, H., Hu, Y.-S., and Chen, L. (2013). Room-temperature stationary sodium-ion batteries for large-scale electric energy storage. Energy Environ. Sci. 6, 2338–2360. doi: 10.1039/c3ee40847g
Park, J.-W., and Park, C.-M. (2015). A fundamental understanding of Li insertion/extraction behaviors in SnO and SnO2. J. Electrochem. Soc. 162, A2811–A2816. doi: 10.1149/2.0891514jes
Park, M.-G., Lee, D.-H., Jung, H., Choi, J.-H., and Park, C.-M. (2018). Sn-based nanocomposite for Li-ion battery anode with high energy density, rate capability, and reversibility. ACS Nano 12, 2955–2967. doi: 10.1021/acsnano.8b00586
Park, M.-S., Wang, G.-X., Kang, Y.-M., Wexler, D., Dou, S.-X., and Liu, H.-K. (2007). Preparation and electrochemical properties of SnO2 nanowires for application in lithium-ion batteries. Angew. Chem. Int. Ed. 46, 750–753. doi: 10.1002/anie.200603309
Qian, J., Xiong, Y., Cao, Y., Ai, X., and Yang, H. (2014). Synergistic Na-storage reactions in Sn4P3 as a high-capacity, cycle-stable anode of Na-ion batteries. Nano Lett. 14, 1865–1869. doi: 10.1021/nl404637q
Qin, J., He, C., Zhao, N., Wang, Z., Shi, C., Liu, E.-Z., et al. (2014). Graphene networks anchored with Sn@Graphene as lithium ion battery anode. ACS Nano 8, 1728–1738. doi: 10.1021/nn406105n
Qin, J., Liu, D., Zhang, X., Zhao, N., Shi, C., Liu, E.-Z., et al. (2017). One-step synthesis of SnCo nano confined in hierarchical carbon nanostructures for lithium ion battery anode. Nanoscale 9, 15856–15864. doi: 10.1039/C7NR04786J
Read, J., Foster, D., Wolfenstine, J., and Behl, W. (2001). SnO2-carbon composites for lithium-ion battery anodes. J. Power Sources 96, 277–281. doi: 10.1016/S0378-7753(00)00569-3
Ren, W., Zhang, H., Guan, C., and Cheng, C. (2018). SnS2 nanosheets arrays sandwiched by N-doped carbon and TiO2 for high-performance Na-ion storage. Green Energy Environ. 3, 42–49. doi: 10.1016/j.gee.2017.09.005
Ren, Y., Wang, J., Huang, X., and Ding, J. (2017). Three-dimensional SnS2 flowers/carbon nanotubes network: extraordinary rate capacity for sodium-ion battery. Mater. Lett. 186, 57–61. doi: 10.1016/j.matlet.2016.09.089
Saadeddin, I., Hilal, H. S., Pecquenard, B., Marcus, J., Mansouri, A., Labrugere, C., et al. (2006). Simultaneous doping of Zn and Sb in SnO2 ceramics: enhancement of electrical conductivity. Solid State Sci. 8, 7–13. doi: 10.1016/j.solidstatesciences.2005.09.002
Slater, M. D., Kim, D., Lee, E., and Johnson, C. S. (2013). Sodium-ion batteries. Adv. Funct. Mater. 23, 947–958. doi: 10.1002/adfm.201200691
Song, H. S., Li, S. L., Gao, L., Xu, Y., Ueno, K., Tang, J., et al. (2013). High-performance top-gated monolayer SnS2 field-effect transistors and their integrated logic circuits. Nanoscale 5, 9666–9670. doi: 10.1039/c3nr01899g
Stevens, D. A., and Dahn, J. R. (2000). High capacity anode materials for rechargeable sodium-ion batteries. J. Electrochem. Soc. 147, 1271–1273. doi: 10.1149/1.1393348
Su, D., Ahn, H.-J., and Wang, G. (2013). SnO2@graphene nanocomposites as anode materials for Na-ion batteries with superior electrochemical performance. Chem. Commun. 49, 3131–3133. doi: 10.1039/c3cc40448j
Sun, L., Ma, T., Zhang, J., Guo, X., Yan, C., and Liu, X. (2019). Double-shelled hollow carbon spheres confining tin as high-performance electrodes for lithium ion batteries. Electrochim. Acta 321:134672. doi: 10.1016/j.electacta.2019.134672
Takamura, T., Suzuki, J., Yamada, C., Sumiya, K., and Sekine, K. (1999). Metal film deposition on carbon anodes for high rate charge–discharge of Li–ion batteries. Surf. Eng. 15, 225–229. doi: 10.1179/026708499101516560
Tamura, N., Fujimoto, M., Kamino, M., and Fujitani, S. (2004). Mechanical stability of Sn-Co alloy anodes for lithium secondary batteries. Electrochim. Acta 49, 1949–1956. doi: 10.1016/j.electacta.2003.12.024
Tarascon, J. M., and Armand, M. (2010). “Issues and challenges facing rechargeable lithium batteries,” in Materials For Sustainable Energy, ed D. Vincent (London, UK; Singapore: Macmillan Publishers Ltd; World Scientific), 171–179. doi: 10.1142/9789814317665_0024
Thangaraju, B., and Kaliannan, P. (2000). Spray pyrolytic deposition and characterization of SnS and SnS2 thin films. J. Phys. D Appl. Phys. 33, 1054–1059. doi: 10.1088/0022-3727/33/9/304
Tian, Q., Zhang, Z., Yang, L., and Hirano, S.-I. (2014). Encapsulation of SnO2 nanoparticles into hollow TiO2 nanowires as high performance anode materials for lithium ion batteries. J. Power Sources 253, 9–16. doi: 10.1016/j.jpowsour.2013.12.049
Todd, A. D. W., Dunlap, R. A., and Dahn, J. R. (2007). Mössbauer effect studies of sputter-deposited tin-cobalt and tin-cobalt-carbon alloys. J. Alloys Compd. 443, 114–120. doi: 10.1016/j.jallcom.2007.06.030
Trahey, L., Vaughey, J. T., Kung, H. H., and Thackeray, M. M. (2009). High-capacity, microporous Cu6Sn5-Sn anodes for Li-ion batteries. J. Electrochem. Soc. 156, A385–A389. doi: 10.1149/1.3094033
Trifonova, A., Wachtler, M., Winter, M., and Besenhard, J. O. (2002). Sn-Sb and Sn-Bi alloys as anode materials for lithium-ion batteries. Ionics 8, 321–328. doi: 10.1007/BF02376044
Uchiyama, H., Hosono, E., Honma, I., Zhou, H., and Imai, H. (2008). A nanoscale meshed electrode of single-crystalline SnO for lithium-ion rechargeable batteries. Electrochem. Commun. 10, 52–55. doi: 10.1016/j.elecom.2007.10.018
Wang, G., Peng, J., Zhang, L., Zhang, J., Dai, B., Zhu, M., et al. (2015). Two-dimensional SnS2@PANI nanoplates with high capacity and excellent stability for lithium-ion batteries. J. Mater. Chem. A 3, 3659–3666. doi: 10.1039/C4TA06384H
Wang, J., Luo, C., Mao, J., Zhu, Y., Fan, X., Gao, T., et al. (2015). Solid-state fabrication of SnS2/C nanospheres for high-performance sodium ion battery anode. ACS Appl. Mater. Interfaces 7, 11476–11481. doi: 10.1021/acsami.5b02413
Wang, Y.-Q., Gu, L., Guo, Y.-G., Li, H., He, X.-Q., Tsukimoto, S., et al. (2012). Rutile-TiO2 nanocoating for a high-rate Li4Ti5O12 anode of a lithium-ion battery. J. Am. Chem. Soc. 134, 7874–7879. doi: 10.1021/ja301266w
Wang, Z., Kou, Z., Miao, C., and Xiao, W. (2019). Improved performance all-solid-state electrolytes with high compacted density of monodispersed spherical Li1.3Al0.3Ti1.7(PO4)3 particles. Ceram. Int. 45, 14469–14473. doi: 10.1016/j.ceramint.2019.04.192
Wen, L., Li, F., and Cheng, H.-M. (2016). Carbon nanotubes and graphene for flexible electrochemical energy storage: from materials to devices. Adv. Mater. 28, 4306–4337. doi: 10.1002/adma.201504225
Wen, Z., Cao, J., Gu, Z., Xu, X., Zhang, F., and Lin, Z. (2008). Research on sodium sulfur battery for energy storage. Solid State Ion. 179, 1697–1701. doi: 10.1016/j.ssi.2008.01.070
Wu, L., Shi, S., Zhang, X., Yang, Y., Liu, J., Tang, S., et al. (2018). Room-temperature pre-reduction of spinning solution for the synthesis of Na3V2(PO4)3/C nanofibers as high-performance cathode materials for Na-ion batteries. Electrochim. Acta 274, 233–241. doi: 10.1016/j.electacta.2018.04.122
Wu, L., Zheng, J., Wang, L., Xiong, X., Shao, Y., Wang, G., et al. (2019). PPy-encapsulated SnS2 nanosheets stabilized by defects on a TiO2 support as a durable anode material for lithium-ion batteries. Angew. Chem. Int. Ed. 58, 811–815. doi: 10.1002/anie.201811784
Wu, Y., Nie, P., Wu, L., Dou, H., and Zhang, X. (2018). 2D MXene/SnS2 composites as high-performance anodes for sodium ion batteries. Chem. Eng. J. 334, 932–938. doi: 10.1016/j.cej.2017.10.007
Xia, L., Wang, S., Liu, G., Ding, L., Li, D., Wang, H., et al. (2016). Flexible SnO2/N-doped carbon nanofiber films as integrated electrodes for lithium-ion batteries with superior rate capacity and long cycle life. Small 12, 853–859. doi: 10.1002/smll.201503315
Xiao, L., Cao, Y., Xiao, J., Wang, W., Kovarik, L., Nie, Z., et al. (2012). High capacity, reversible alloying reactions in SnSb/C nanocomposites for Na-ion battery applications. Chem. Commun. 48, 3321–3323. doi: 10.1039/c2cc17129e
Xiao, W., Wang, Z., Zhang, Y., Fang, R., Yuan, Z., Miao, C., et al. (2018). Enhanced performance of P(VDF-HFP)-based composite polymer electrolytes doped with organic-inorganic hybrid particles PMMA-ZrO2 for lithium ion batteries. J. Power Sources 382, 128–134. doi: 10.1016/j.jpowsour.2018.02.012
Xin, S., Yin, Y.-X., Guo, Y.-G., and Wan, L.-J. (2014). A high-energy room-temperature sodium-sulfur battery. Adv. Mater. 26, 1261–1265. doi: 10.1002/adma.201304126
Xu, Y., Zhu, Y., Liu, Y., and Wang, C. (2013). Electrochemical performance of porous carbon/tin composite anodes for sodium-ion and lithium-ion batteries. Adv. Energy Mater. 3, 128–133. doi: 10.1002/aenm.201200346
Xue, L., Fu, Z., Yao, Y., Huang, T., and Yu, A. (2010). Three-dimensional porous Sn-Cu alloy anode for lithium-ion batteries. Electrochim. Acta 55, 7310–7314. doi: 10.1016/j.electacta.2010.07.015
Yabuuchi, N., Kubota, K., Dahbi, M., and Komaba, S. (2014). Research development on sodium-ion batteries. Chem. Rev. 114, 11636–11682. doi: 10.1021/cr500192f
Yang, J., Wachtler, M., Winter, M., and Besenhard, J. O. (1999). Sub-microcrystalline Sn and Sn-SnSb powders as lithium storage materials for lithium-ion batteries. Electrochem. Solid-State Lett. 2, 161–163. doi: 10.1149/1.1390769
Yang, S., Yue, W., Zhu, J., Ren, Y., and Yang, X. (2013). Graphene-based mesoporous SnO2 with enhanced electrochemical performance for lithium-ion batteries. Adv. Funct. Mater. 23, 3570–3576. doi: 10.1002/adfm.201203286
Ying, H., and Han, W.-Q. (2017). Metallic Sn-based anode materials: application in high-performance lithium-ion and sodium-ion batteries. Adv. Sci. 4:1700298. doi: 10.1002/advs.201700298
Yoon, S., Lee, J.-M., Kim, H., Im, D., Doo, S.-G., and Sohn, H.-J. (2009). An Sn-Fe/carbon nanocomposite as an alternative anode material for rechargeable lithium batteries. Electrochim. Acta 54, 2699–2705. doi: 10.1016/j.electacta.2008.11.060
Yuan, Z., Dong, L., Gao, Q., Huang, Z., Wang, L., Wang, G., et al. (2018). SnSb alloy nanoparticles embedded in N-doped porous carbon nanofibers as a high-capacity anode material for lithium-ion batteries. J. Alloys Compd. 777, 775–783. doi: 10.1016/j.jallcom.2018.10.295
Zhang, H.-X., Feng, C., Zhai, Y.-C., Jiang, K., Li, Q., and Fan, S.-S. (2009). Cross-stacked carbon nanotube sheets uniformly loaded with SnO2 nanoparticles: a novel binder-free and high-capacity anode material for lithium-ion batteries. Adv. Mater. 21, 2299–2304. doi: 10.1002/adma.200802290
Zhang, W.-J. (2011). A review of the electrochemical performance of alloy anodes for lithium-ion batteries. J. Power Sources 196, 13–24. doi: 10.1016/j.jpowsour.2010.07.020
Zhao, K., Zhang, L., Xia, R., Dong, Y., Xu, W., Niu, C., et al. (2016). SnO2 quantum dots@graphene oxide as a high-rate and long-life anode material for lithium-ion batteries. Small 12, 588–594. doi: 10.1002/smll.201502183
Zhao, Y., Li, X., Yan, B., Li, D., Lawes, S., and Sun, X. (2015). Significant impact of 2D graphene nanosheets on large volume change tin-based anodes in lithium-ion batteries: a review. J. Power Sources 274, 869–884. doi: 10.1016/j.jpowsour.2014.10.008
Zheng, P., Dai, Z., Zhang, Y., Dinh, K. N., Zheng, Y., Fan, H., et al. (2017). Scalable synthesis of SnS2/S-doped graphene composites for superior Li/Na-ion batteries. Nanoscale 9, 14820–14825. doi: 10.1039/C7NR06044K
Zheng, Y., Zhou, T., Zhang, C., Mao, J., Liu, H., and Guo, Z. (2016). Boosted charge transfer in SnS/SnO2 heterostructures: toward high rate capability for sodium-ion batteries. Angew. Chem. 128, 3469–3474. doi: 10.1002/ange.201510978
Zhou, X., Bao, J., Dai, Z., and Guo, Y.-G. (2013a). Tin nanoparticles impregnated in nitrogen-doped graphene for lithium-ion battery anodes. J. Phys. Chem. C 117, 25367–25373. doi: 10.1021/jp409668m
Zhou, X., Wan, L.-J., and Guo, Y.-G. (2013b). Binding SnO2 nanocrystals in nitrogen-doped graphene sheets as anode materials for lithium-ion batteries. Adv. Mater. 25, 2152–2157. doi: 10.1002/adma.201300071
Zhou, X., Yu, L., and Lou, X. W. (2016). Formation of uniform N-doped carbon-coated SnO2 submicroboxes with enhanced lithium storage properties. Adv. Energy Mater. 6:1600451. doi: 10.1002/aenm.201600451
Zhou, X., Yu, Y., Yang, J., Wang, H., Jia, M., and Tang, J. (2019). Cross-linking tin-based metal-organic frameworks with encapsulated silicon nanoparticles: high-performance anodes for lithium-ion batteries. ChemElectroChem 6, 2056–2063. doi: 10.1002/celc.201900235
Zhu, H., Jia, Z., Chen, Y., Weadock, N., Wan, J., Vaaland, O., et al. (2013). Tin anode for sodium-ion batteries using natural wood fiber as a mechanical buffer and electrolyte reservoir. Nano Lett. 13, 3093–3100. doi: 10.1021/nl400998t
Keywords: tin, tin compound, anode, lithium-ion batteries, sodium-ion batteries
Citation: Mou H, Xiao W, Miao C, Li R and Yu L (2020) Tin and Tin Compound Materials as Anodes in Lithium-Ion and Sodium-Ion Batteries: A Review. Front. Chem. 8:141. doi: 10.3389/fchem.2020.00141
Received: 29 December 2019; Accepted: 14 February 2020;
Published: 19 March 2020.
Edited by:
Xianwen Wu, Jishou University, ChinaReviewed by:
Yunjian Liu, Jiangsu University, ChinaLingjun Li, Changsha University of Science and Technology, China
Copyright © 2020 Mou, Xiao, Miao, Li and Yu. This is an open-access article distributed under the terms of the Creative Commons Attribution License (CC BY). The use, distribution or reproduction in other forums is permitted, provided the original author(s) and the copyright owner(s) are credited and that the original publication in this journal is cited, in accordance with accepted academic practice. No use, distribution or reproduction is permitted which does not comply with these terms.
*Correspondence: Wei Xiao, eHd5bHlxMjAwNkAxMjYuY29t