- 1College of Chemistry and Chemical Engineering, Henan Polytechnic University, Jiaozuo, China
- 2School of Materials Science & Engineering, Henan Polytechnic University, Jiaozuo, China
Flexible, water-resistant, and air-stable hydrogen storage material (named PMMA-LiBH4/GMF), consisting of LiBH4 nanoparticles confined by poly (methylmethacrylate) (PMMA) and reduced graphene oxide (rGO) modified melamine foam (GMF), were prepared by a facile method. PMMA-LiBH4/GMF can recover original shape after compression at the strain of 50% and exhibits highly hydrophobic property (water contact angle of 123°). Owing to the highly hydrophobic property and protection of PMMA, PMMA-LiBH4/GMF demonstrates outstanding water-resistance and air-stability. Significantly, the onset dehydrogenation temperature of PMMA-LiBH4/GMF at first step is reduced to 94°C, which is 149°C less than that of LiBH4/GMF, and the PMMA-LiBH4/GMF desorbs 2.9 wt% hydrogen within 25 min at 250°C, which is obviously more than the dehydrogenation amount of LiBH4/GMF under the same conditions. It's our belief that the flexible, water-resistant and air-stable PMMA-LiBH4/GMF with a simple preparation route will provide a new avenue to the research of hydrogen storage materials.
Introduction
Hydrogen has been considered as a promising energy carrier owing to its higher power density, green and abundant resources (Nielsen et al., 2010; Liu et al., 2016). However, the safe and efficient hydrogen storage technique is one of the key technical obstacles to limit the commercial application of hydrogen-fueled vehicles (Schlapbach and Züttel, 2001). Compared to traditional high-pressure and liquid hydrogen storage systems (Zhang et al., 2014), the solid-state metal hydrides are prospective to realize future hydrogen storage goals due to their safety, compactness and efficiency (Sakintuna et al., 2007). Lithium borohydride (LiBH4) is well-known as one of the most promising metal complex hydrides, which has high gravimetric and volumetric hydrogen densities of 18.5 wt% H2 and 121 kg H2/m3, respectively (Zhang et al., 2017; Wu et al., 2019). Unfortunately, the practical applications of LiBH4 are restricted because of the challenging thermodynamics, slow kinetics and the sensitivity to water and oxygen. Moreover, the reversibility of LiBH4 is also limited by the harsh rehydrogenation conditions (high temperature up to 600°C and high pressure up to 35 MPa H2) (Zhao et al., 2014; Zang et al., 2018).
To overcome the drawbacks of LiBH4, several approaches, including doping catalysts, nanoconfinement and partial cation substitution, have been developed to lower dehydrogenation temperature, to increase the hydrogen desorption capacity and promote the rehydrogenation reaction under mild conditions (Zhou et al., 2012; Cai et al., 2013). Nanoconfinement is also a viable way to improve the dehydrogenation performance by increasing surface areas and decreasing the diffusion lengths (Huang et al., 2015). Therefore, many efforts have been applied to confine LiBH4 in carbon, SiO2 and metal-organic frameworks (MOFs) (Gross et al., 2008; Liu et al., 2010; Ngene et al., 2010; Sun et al., 2011; Vajo, 2011). LiBH4 confined in nanoporous carbon materials exhibits lower temperatures of phase transformation, melting, and dehydrogenation temperature as compared with bulk material, and reduced the formation of B2H6 (Gross et al., 2008; Liu et al., 2011). Cho et al. (2016) reported an environmentally stable hydrogen storage material of Mg nanocrystals encapsulated by atomically thin and gas-selective reduced graphene oxide (rGO) sheets, exhibits distinguishingly dense hydrogen storage (6.5 wt% and 0.105 Kg H2 per liter in the total composite) (Hecchetto et al., 2009). Gosalawit-Utke et al. (2014) reported that LiBH4 was confined by poly(methyl methacrylate)-co-butyl methacrylate (P(MMA-co-BMA)), and the onset dehydrogenation temperature was reduced to ~80°C. Zhao et al. (2010) reported a water-resistant system of ammonia borane (AB) confined by poly(methyl acrylate) (PMA), and found the dehydrogenation performance of AB was improved. An air-stable crystalline Mg/PMMA nanocomposites rapidly uptaked hydrogen (<30 min at 200°C) with high capacity (~6 wt% in Mg, ~4% overall) in the absence of heavy-metal catalysts (Jeon et al., 2011). PMMA, owning to the high permeability ratio of H2/O2, is better than other similar polymers in terms of gas selectivity. Therefore, PMMA can be used in hydrogen storage to keep away the water and oxygen but let the hydrogen get in or out freely (Wang et al., 2008; Liang et al., 2011). Huang et al. (2014) reported that LiBH4 nanoconfined in PMMA exhibits excellent hydrophobic ability and dehydrogenation. However, LiBH4/PMMA may be stiff and rigid due to the glass state of PMMA at room temperature, which may hinder the application of LiBH4/PMMA composites in the flexibility demanded fields.
In the present work, we reported a facile method to prepare flexible, water-resistant and air-stable PMMA-LiBH4/GMF for hydrogen storage and release, in which LiBH4 was confined by PMMA and GMF. The GMF was prepared by simple dipped coating and high temperature reduction. And then, we simply dipped GMF into LiBH4/PMMA solution (tetrahydrofuran, THF, as solvent), and dried the foam in the glove box under Ar atmosphere at room temperature for 72 h. Owing to the super-absorbent property, GMF can adsorb a large amount of THF solution as well as the solutes of LiBH4 and PMMA in the THF solution. After drying, PMMA-LiBH4/GMF, consisting of PMMA confined LiBH4 nanoparticles, can be successfully prepared. It is found the prepared PMMA-LiBH4/GMF is flexible, which can be compressive and maintains the elasticity for many times. Moreover, PMMA-LiBH4/GMF is also found to be highly hydrophobic, and the contact angle reached 123°, indicating there is water-resistant property for the new foam. The hydrophobic surface of the foam prevents water from permeating into the pores of the foam and PMMA shell on the LiBH4 nanoparticles, which also provides additional protection of LiBH4 nanoparticles to keep away from contacting with O2. Most importantly, the onset decomposing temperature of LiBH4 in the nanocomposite foam is reduced from 285 to 94°C and the main dehydrogenation temperature also decreased from 470 to 340–380°C. Unlike the common LiBH4 and LiBH4/PMMA nanocomposites, our PMMA-LiBH4/GMF exhibits flexibility, water-resistance, air-stability, and improved hydrogen releasing properties.
Experimental
Materials
Lithium borohydride solution (2.0 M LiBH4 in tetrahydrofuran) was purchased from Aladdin, PMMA was provided by TCI. All the reagents were used without any further purification and were stored and handled in a glove box (Etelux Lab 2000) equipped with an Ar recirculation system, and the water and hydrogen were kept below 0.1 ppm to prevent the oxidation.
Preparation of PMMA-LiBH4/GMF
A two-step process was employed to prepare the PMMA-LiBH4/GMF materials. Firstly, GMF was prepared as the literature reported by Zhu et al. (2015). Briefly, melamine foam (MF) was cut into blocks and then ultrasonically cleaned using ethanol and deionized water, successively. The blocks were dried at room temperature. Then, the obtained clean MF was dipped into a graphene oxide (GO) suspension (5 mg/mL), and squeezed/released process in GO suspension was repeated for three times. Subsequently, GMF was obtained by heating the foam at a fixed temperature of 160°C for 6 h at an atmospheric environment to reduce GO into reduced graphene oxide (rGO). Secondly, PMMA-LiBH4/GMF was prepared by impregnating GMF into a PMMA-LiBH4 solution (tetrahydrofuran, THF, as solvent) with PMMA concentration of 70 mg/mL, then the modified foam was dried in the glove box at room temperature for 72 h. There was only an organic gas adsorption device in the glove box. An operation to change the atmosphere in glove box by Ar every 18 h to reduce the concentration of THF until the THF was completely dried.
Characterizations
The microstructure was observed by scanning electron microscope (SEM, Zeiss Supa 50VP, Germany) with 15 kV. The phase structure of samples was characterized by X-ray diffraction (XRD) using a Smart-lab, CuKα radiation as a light source. All the XRD measurements were conducted at room temperature, and the scanning range of 5–70°. Contact angle was measured by contact angle meter (JC2000C, Shanghai). The compressibility of the foams was tested by the universal testing machine (WSM-10KN, Changchun).
Hydrogen Storage Measurements
The dehydrogenation behavior was measured by thermogravimetry (TG, SDT, Q600) connected directly with quadrupole mass spectroscopy (MS, HPR 20, QIC). The samples were heated at a temperature range from room temperature to 500°C at a heating rate of 4°C/min with an argon purge rate of 90 mL/min. A Sievert's apparatus purchased from Zhejiang University was used to determine the temperature dependence of the hydrogen desorption and absorption behavior. For each test, ~0.1 g of the as-prepared samples were loaded into a stainless steel tube reactor within a glovebox, the reactor was then connected to the Sieverts-type apparatus. For the isothermal experiment, the sample was quickly heated to the desired temperature, and then the temperature was maintained during the following test.
Results and Discussion
Preparation of PMMA-LiBH4/GMF
A two steps process was used to prepare PMMA-LiBH4/GMF: (1) Graphene-coated melamine foam (GMF) was firstly prepared by dipping clean MF into a graphene oxide solution, and then the foam was heated to make the GO be reduced into reduced graphene oxide (rGO), (2) GMF was immersed into the LiBH4/PMMA THF solution, and then the foam was allowed to evaporate THF and form PMMA confined LiBH4 nanoparticles in the G-Melamine matrix.
As described above, PMMA-LiBH4/GMF was prepared by a facile two-step process. The detailed preparation route is shown in Figure 1. As shown in Figure 1, the MF shows the color change from white to black after thermal reduction of GO. The PMMA and LiBH4 remain in the GMF as the evaporation of THF solvent. Moreover, the size of LiBH4 nanoparticles can be controlled owing to the confinement effect of PMMA. In conclusion, PMMA-LiBH4/GMF can be facile prepared by the simple dipping-evaporation process.
Morphology of PMMA-LiBH4/GMF
The morphologies of MF and GMF were observed by SEM as shown in Figures 2A–D. The SEM images of MF show that the pure MF is a 3D porous structure with pore diameters ranging from tens to hundreds of micrometers. Figure 2B exhibits the smooth sponge skeletons. After the introduction of graphene, the GMF keeps the 3D porous structure (Figure 2C), indicating that the network structures of MF are not damaged when the thermal reduction process of GO. However, the sponge skeletons of GMF are rougher than those of MF, this could be related to the presence of reduced graphene oxide (rGO) on the surface of sponge skeletons.
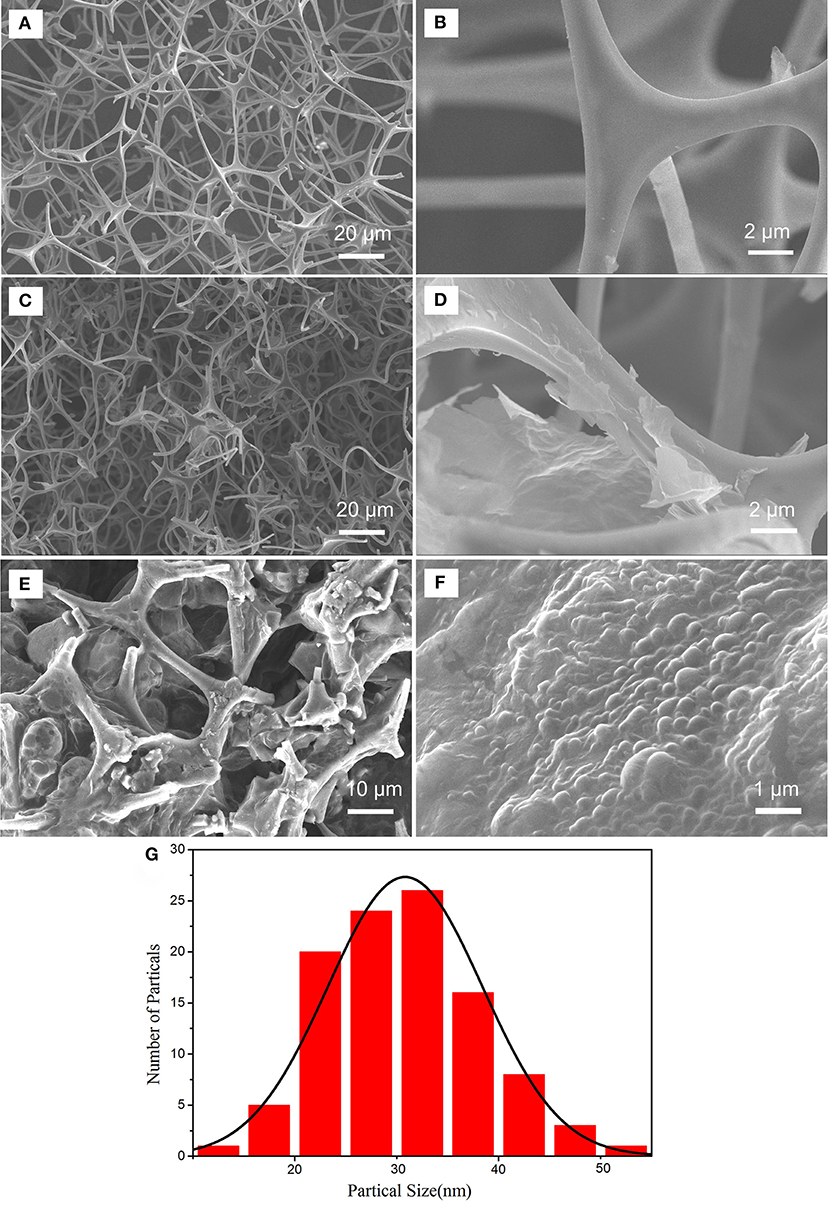
Figure 2. SEM images of MF (A,B), GMF (C,D), PMMA-LiBH4/GMF (E,F) and particle distribution of LiBH4 nanoparticles in PMMA-LiBH4/GMF (G).
The magnified image further demonstrates that rGO with folding edge is stacked on the surface of sponge skeletons (Figure 2D). The results suggest that the GMF is successfully prepared via the thermal reduction of GO over sponge skeletons. Figures 2E,F show that PMMA-LiBH4 nanoparticles can fill into the pore of GMF. The particle distribution of PMMA-LiBH4 nanoparticles is shown in Figure 2G. The average particles size is about 30 nm. The size decrease of LiBH4 nanoparticles as wrapped by PMMA was also reported by Li et al. (2014).
XRD Analysis of PMMA-LiBH4/GMF
The XRD technique was used to study the structure of different foams. As shown in Figure 3A, MF and GMF show similar XRD patterns, indicating the coating of graphene nanosheet do not affect the primary structure of MF. Moreover, the characteristic XRD peaks of layered graphene cannot be detected. However, the Raman spectra confirm the presence of graphene (Figure 3B). The Raman peaks attributed to the graphene can not be observed for MF, while the D and G graphene peaks of GMF associated with sp2 and sp3 bonds are observed in 1,370 and 1,580 cm−1, respectively. These results illustrate that GMF is obtained successfully in this experiment. As known from the literature (Zhao et al., 2010; Gosalawit-Utke et al., 2014), the crystalline of LiBH4 disappeared when LiBH4 was confined by PMMA, due to form amorphous and/or nanocrystalline structure for LiBH4. The XRD patterns of LiBH4/GMF and PMMA-LiBH4/GMF are shown in Figure 4. The XRD peaks corresponding to LiBH4 can be detected, and the LiBH4 phase is still dominant phase in both PMMA-LiBH4/GMF and LiBH4/GMF, illustrating that LiBH4 is successfully confined in PMMA and foam. However, the intensity of LiBH4 peaks in PMMA-iLiBH4/GMF is much lower than that of LiBH4/GMF, this can be related to the nano size that resulted by the confinement effect of PMMA. Meanwhile, for LiBH4/GMF, the peaks of BN, Li3N, and B can be detected, indicating LiBH4 and GMF reacted partially during the preparation. Besides LiBH4, only XRD peaks ascribed to the BN phase can be observed for PMMA-LiBH4/GMF, indicating the PMMA can effectively restrict the reaction between LiBH4 and GMF.
Flexibility and Water-Resistance of PMMA-LiBH4/GMF
In order to demonstrate the flexibility and compressibility of PMMA-LiBH4/GMF, the folding and compressive tests have been conducted as shown in Figure 5. After folding, the PMMA-LiBH4/GMF can completely recover to its original shape and size immediately (Figures 5A–C). Similarly, as the compressive force released, the foam also can rapidly recover to its original shape (Figures 5D,E). The results indicate our PMMA-LiBH4/GMF exhibits excellent flexibility though there is glassy PMMA on or in the foam.
To further evaluate the flexibility of the PMMA-LiBH4/GMF, three compressive loading-unloading cycle tests were measured. As shown in Figure 6A, the foam shows obvious hysteresis loops for three compressive loading cycles. It is found the elastic modulus (E), the stress at 50% strain and the dissipated energies are 344/159/157 Pa, 19/18/17 kPa, and 200/134/123 kJ/m3 for the first, second, and third compression, respectively (Figures 6B–D). Herein, the mechanical properties of PMMA-LiBH4/GMF decrease after the first loading. However, the loading-unloading loops of second and third compression are almost overlapped, indicating the mechanical properties of the foam become stable after the first loading.
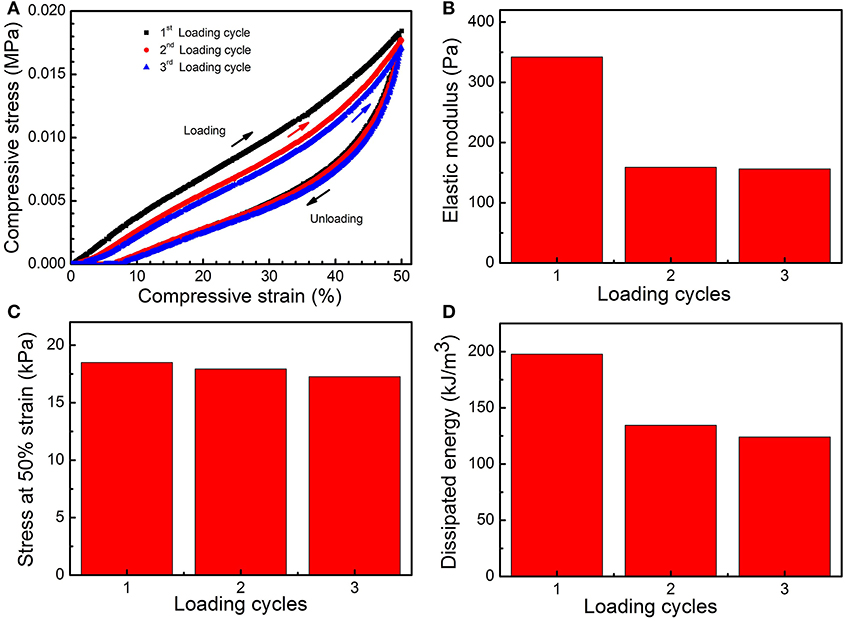
Figure 6. Hysteresis loops (A), elastic modulus (E) (B), stress at 50% strain (C), and dissipated energies (D) of PMMA-LiBH4/GMF for the first, second, and third compression.
It's well-known that graphene modified MF is superhydrophobic, and it's expected our PMMA-LiBH4/GMF will be also highly hydrophobic to resist water diffused into the foam, and subsequently reacted with LiBH4 nanoparticles. In order to determine the hydrophobicity of PMMA-LiBH4/GMF, the contact angle was measured. As shown in Figure 7, the water contact angle of GMF, PMMA/GMF and PMMA-LiBH4/GMF is 145, 142, 123°, respectively. As reported in literature (Li et al., 2014), GMF exhibited the best hydrophobicity. Although the contact angle slightly decreased, GMF still possessed high hydrophobicity after adding PMMA. Most interestingly, our PMMA-LiBH4/GMF also preserves high hydrophobicity of 123° (Figure 7C). For more visible, a water droplet is dropped on the surface of various foams. Consistently, water droplet was quickly permeated into the original MF, whereas water droplet on the GMF and PMMA-LiBH4/GMF shows semicircle shape. Therefore, our PMMA-LiBH4/GMF is highly hydrophobic, which will protect the LiBH4 nanoparticles away from the contact with moisture in the air.
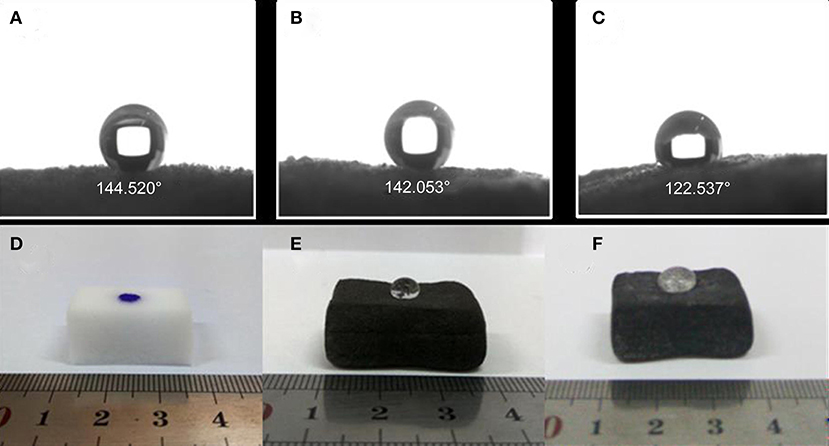
Figure 7. The contact angle of (A) GMF, (B) PMMA/GMF and (C) PMMA-LiBH4/GMF and the picture of water droplet (stain with methylene blue) on the surface of (D) MF, (E) GMF, and (F) PMMA-LiBH4/GMF.
Dehydrogenation Properties of PMMA-LiBH4/GMF
Figure 8 shows the TPD curves of PMMA-LiBH4/GMF. It is obvious that the dehydrogenation temperature of LiBH4 is significantly reduced by combining with PMMA and GMF. Pure LiBH4 starts to decompose at 290°C and rapidly release hydrogen at around 400°C. The dehydrogenation of LiBH4 can be enhanced by the introduction of GFM. For LiBH4/GMF composite, the onset dehydrogenation temperature is reduced to 243°C, which is 47°C lower than that of pure LiBH4, moreover, three dehydrogenation stages can be observed, and the total dehydrogenation is inferior to the pure LiBH4 under the studied temperature region. More interestingly, the majority of dehydrogenation temperature significantly shifts to lower temperature after the introduction of PMMA. The onset dehydrogenation temperature of PMMA-LiBH4/GMF at first step is reduced to 94°C, which is 149°C less than that of LiBH4/GMF, and the second step is also lowered by 56°C, from 265 to 209°C. Moreover, the dehydrogenation amounts of PMMA-LiBH4/GMF at the three steps are more than that of LiBH4/GMF. More importantly, about 7 wt% hydrogen can be fast released at the temperature range from 350 to 400°C. The total dehydrogenation amounts of PMMA-LiBH4/GMF are about 11 wt%, which are obviously higher than those of pure LiBH4 and LiBH4/GMF when the temperature was lower than 500°C. Therefore, the co-existence of GMF and PMMA can greatly improve dehydrogenation capacity of LiBH4.
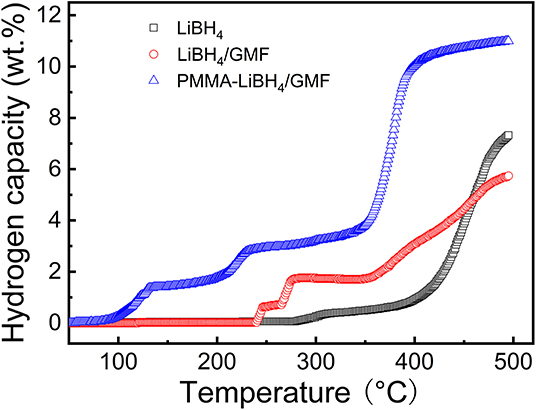
Figure 8. Temperature programmed dehydrogenation (TPD) curves of pure LiBH4, LiBH4/GMF, and PMMA-LiBH4/GMF.
To understand the underlying reason for the excellent dehydrogenation performances of PMMA-LiBH4/GMF, XRD patterns and FTIR spectra of PMMA-LiBH4/GMF at different dehydrogenation states during hydrogen desorption process were conducted as shown in Figures 9A,B. Seen from the XRD in Figure 4, there is a partial reaction between LiBH4 and GMF during the preparation of PMMA-LiBH4/GMF. After dehydrogenation at 180°C, the XRD peaks at 2θ = 43.1, 50.4, 74.4° can be detected and was assigned to BN phase besides the peaks of LiBH4 (Figure 9A), which maintains a similar structure with fresh PMMA-LiBH4/GMF. In the FTIR spectra, B-H bond has a relatively weak intensity comparing with the fresh PMMA-LiBH4/GMF, which may be ascribed to the rise in temperature stimulating the reaction between LiBH4 and GMF. And synchronously no B-O bond is detected at 180°C (Figure 9B). Thus, the possible mechanism before 180°C conjectured is that GMF as a N source has a chemical reaction with LiBH4. When the dehydrogenation temperature increase to 270°C, the new XRD peak at about 30° appears, indicating Li3BO3 is formed. Here, LiBH4, BN are still the main components. The B-O bond is observed in the FTIR of the sample dehydrogenated at 270°C (Figure 9B). Based on these results, we come to the conclusion that the formation of Li3BO3 may be due to the occurred chemical reaction between LiBH4 and PMMA (O source) in the second dehydrogenation step. The dehydrogenation amount is relatively poorer under this temperature phase, which illustrates not too strong chemical reaction between PMMA and LiBH4 with increasing the temperature to 400°C, Li3BO3 and BN become the main peaks in XRD pattern of PMMA-LiBH4/GMF, and no LiBH4 can be detected. Additionally, seen from the FTIR spectra, B–O bond is existed, and the B–H bond gradually weakens and almost can not be resolved at 400°C. This phase presented an optimal dehydrogenation amount. This phenomenon attests that a strong chemical reaction occurs between PMMA and LiBH4. Based on XRD and FTIR results, the hydrogen release of PMMA-LiBH4/GMF foam mainly depends on the reaction between PMMA and LiBH4. Potentially, due to the formation of Li3BO3, the new reaction pathway of LiBH4 lead to the low dehydrogenation temperature and fast decomposition kinetics of PMMA-LiBH4/GMF. Additionally, the possible electrostatic interaction between B and O atom weakens the B-H bonds and lowers the hydrogen desorption temperature. Summarily, the existence of PMMA and GMF has an accelerated effect toward the dehydrogenation property of LiBH4.
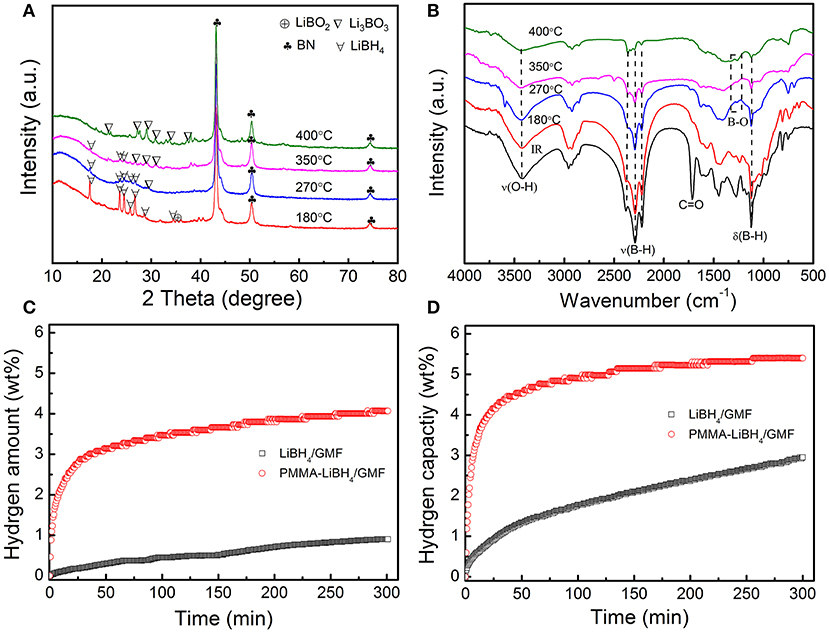
Figure 9. XRD (A) and FTIR (B) patterns of dehydrogenated of PMMA-LiBH4/GMF at 180, 270, 350, and 400°C and the isothermal hydrogen desorption curve of LiBH4/GMF, PMMA-LiBH4/GMF (C,D).
In order to further study the effects of GMF and PMMA on the dehydrogenation properties of LiBH4, the isothermal hydrogen desorption experiments were also conducted (Figures 9C,D). PMMA-LiBH4/GMF desorbs 2.9 wt% hydrogen within 25 min at 250°C, which is obviously more than the dehydrogenation amount of LiBH4/GMF at the same conditions. Moreover, 4.2% hydrogen can be released from PMMA-LiBH4/GMF within 25 min at 350°C. Our PMMA-LiBH4/GMF exhibits excellent dehydrogenation performance, which is better than LiBH4/GMF and pure LiBH4 (Zhu et al., 2015). Combining the above experimental results, the initial onset dehydrogenation temperature values of 290, 243, and 94°C obtained from TPD curves can be ascribed to LiBH4, LiBH4/GMF, and PMMA-LiBH4/GMF, respectively. When compared with the initial onset dehydrogenation temperature of these materials, PMMA-LiBH4/GMF presents an excellent dehydrogenation property in LiBH4. And coincidentally, PMMA-LiBH4/GMF reveals an optimal total dehydrogenation capacity under uniform reaction system.
Conclusion
In the present work, we provided a convenient route to synthesize flexible, water-resistant and air-stable PMMA-LiBH4/GMF with improved hydrogen releasing properties. GMF and PMMA can not only protect LiBH4 from water and oxygen, but also hinder growth and agglomeration of the particles Significantly, our PMMA-LiBH4/GMF can maintain the original flexible characteristics and hydrophobicity of GMF. More importantly, our PMMA-LiBH4/GMF exhibits excellent hydrogen desorption property, which is better than those of LiBH4/GMF and pure LiBH4. The improvement of dehydrogenation property of PMMA-LiBH4/GMF may be due to chemical effect between PMMA (or GMF) and LiBH4. We hope our PMMA-LiBH4/GMF provides a new avenue to the next generation hydrogen storage materials with novel functionalities.
Data Availability Statement
All datasets generated for this study are included in the article/supplementary material.
Author Contributions
YF and DC conducted the synthesis experiment, performance test, and paper writing. ZY participated in performance testing. GF, DZ, and QC participated in analysis of the results. QC and BL conducted the design, guidance, analysis of experiment results, and paper revision.
Funding
This research was financially supported by the National Natural Science Foundation of China (51871090, U1804135, and 51671080), Plan for Scientific Innovation Talent of Henan Province (194200510019) and Program for Innovative Research Team (in Science and Technology) in the University of Henan Province (16IRTSTHN005).
Conflict of Interest
The authors declare that the research was conducted in the absence of any commercial or financial relationships that could be construed as a potential conflict of interest.
References
Cai, W. T., Wang, H., Jiao, L. F., Wang, Y. J., and Zhu, M. (2013). Remarkable irreversible and reversible dehydrogenation of LiBH4 by doping with nanosized cobalt metalloid compounds. Int. J. Hydrog. Energy 38, 3304–3312. doi: 10.1016/j.ijhydene.2012.10.032
Cho, E. S., Ruminski, A. M., Aloni, S., Liu, Y. S., Guo, J. H., and Urban, J. J. (2016). Graphene oxide/metal nanocrystal multilaminates as the atomic limit for safe and selective hydrogen storage. Nature 7, 10804–10809. doi: 10.1038/ncomms11145
Gosalawit-Utke, R., Meethom, S., Pistidda, C., Milanese, C., Laipple, D., Saisopa, T., et al. (2014). Destabilization of LiBH4 by nanoconfinement in PMMA-co-BM polymer matrix for reversible hydrogen storage. Int. J. Hydrog. Energy 39, 5019–5029. doi: 10.1016/j.ijhydene.2014.01.078
Gross, A. F., Vajo, J. J., Van Atta, S. L., and Olson, G. L. (2008). Enhanced hydrogen storage kinetics of LiBH4 in nanoporous carbon scaffolds. J. Phys. Chem. C 112, 5651–5657. doi: 10.1021/jp711066t
Hecchetto, R., Bazzanella, N., Miotello, A., Carotenuto, G., and Nicolais, L. (2009). Hydrogen sorption in metal-polymer composites: the role of interfaces. J. Appl. Phys. 105:083513. doi: 10.1063/1.3106583
Huang, J. M., Yan, Y. R., Ouyang, L. Z., Wang, H., Liu, J. W., and Zhu, M. (2014). Increased air stability and decreased dehydrogenation temperature of LiBH4 via modification within poly(methylmethacrylate). Dalton Trans. 43, 410–413. doi: 10.1039/c3dt51989a
Huang, J. M., Yan, Y. R., Ouyang, L. Z., Wang, H., and Zhu, M. (2015). Dehydrogenation mechanism of LiBH4 by Poly(methyl methacrylate). J. Alloys Compd. 645, S100–S102. doi: 10.1016/j.jallcom.2014.12.268
Jeon, K. J., Moon, H. R., Ruminski, A. M., Jiang, B., Kisielowski, C., Bardhan, R., et al. (2011). Air-stable magnesium nanocomposites provide rapid and high-capacity hydrogen storage without using heavy-metal catalysts. Nat. Mater. 10, 286–290. doi: 10.1038/nmat2978
Li, Y. T., Zhang, Q. A., and Fang, F. (2014). Facile self-assembly of light metal borohydrides with controllable nanostructures. RSC Adv. 4, 983–986. doi: 10.1039/C3RA45867A
Liang, C., Liu, Y. F., Fu, H. L., Ding, Y. F., Gao, M. X., and Pan, H. G. (2011). Li-Mg-N-H-based combination systems for hydrogen storage. J. Alloys Compd. 509, 7844–7853. doi: 10.1016/j.jallcom.2011.04.123
Liu, X. F., Peaslee, D., Jost, C. Z., Baumann, T. F., and Majzoub, E. H. (2011). Systematic pore-size effects of nanoconfinement of LiBH4: elimination of diborane release and tunable behavior for hydrogen storage applications. Chem. Mater. 23, 1331–1336. doi: 10.1021/cm103546g
Liu, X. F., Peaslee, D., Jost, C. Z., and Majzoub, E. H. (2010). Controlling the decomposition pathway of LiBH4 via confinement in highly ordered nanoporous carbon. J. Phys. Chem. C 114, 14036–14041. doi: 10.1021/jp1055045
Liu, Y. F., Yang, Y. X., Gao, M. X., and Pan, H. G. (2016). Tailoring thermodynamics and kinetics for hydrogen storage in complex hydrides towards applications. Chem. Rec. 16, 189–204. doi: 10.1002/tcr.201500224
Ngene, P., Adelhelm, P., Beale, A. M., de Jong, K. P., and de Jongh, P. E. (2010). LiBH4/SBA-15 nanocomposites prepared by melt infiltration under hydrogen pressure: synthesis and hydrogen sorption properties. J. Phys. Chem. C 114, 6163–6168. doi: 10.1021/jp9065949
Nielsen, T. K., Bösenberg, U., Gosalawit, R., Dornheim, M., Cerenius, Y., Besenbacher, F., et al. (2010). A reversible nanoconfined chemical reaction. ACS Nano 4, 3903–3908. doi: 10.1021/nn1006946
Sakintuna, B., Darkrim, F. L., and Hirscher, M. (2007). Metal hydride materials for solid hydrogen storage: a review. Int. J. Hydrog. Energy 32, 1121–1140. doi: 10.1016/j.ijhydene.2006.11.022
Schlapbach, L., and Züttel, A. (2001). Hydrogen-storage materials for mobile applications. Nature 414, 353–358. doi: 10.1038/35104634
Sun, W. W., Li, S. F., Mao, J. F., Guo, Z. P., Liu, H. K., Dou, S. X., et al. (2011). Nanoconfinement of lithium borohydride in Cu-MoFs towards low temperature dehydrogenation. Dalton Trans. 40, 5673–5676. doi: 10.1039/c0dt01727b
Vajo, J. J. (2011). Influnce of nano-confinement on the thermodynamics and dehydrogenation kinetics of metal hydrides. Curr. Opin. Solid State Mater. Sci. 15, 52–61. doi: 10.1016/j.cossms.2010.11.001
Wang, Q., Chen, Y. U., Tao, M. D., and Wu, C. L. (2008). Review on hydrogen storage properties and reaction mechanism of metal-N-H systems. Rare Met. Mater. Eng. 37, 382–385.
Wu, R. Y., Ren, Z. H., Zhang, X., Lu, Y. H., Li, H. W., Gao, M. X., et al. (2019). Nanosheet-like lithium borohydride hydrate with 10 wt% hydrogen release at 70°C as a chemical hydrogen storage candidate. J. Phys. Chem. Lett. 10, 1872–1877. doi: 10.1021/acs.jpclett.9b00416
Zang, L., Sun, W. Y., Liu, S., Huang, Y. K., Yuan, H. T., Tao, Z. L., et al. (2018). Enhanced hydrogen storage properties and reversibility of LiBH4 confined in two-dimensional Ti3C2. ACS Appl. Mater. Interfaces 10, 19598–19604. doi: 10.1021/acsami.8b02327
Zhang, L. T., Chen, L. X., Xiao, X. Z., Chen, Z. W., Wang, S. K., Fan, X. L., et al. (2014). Superior dehydrogenation performance of nanoscale lithium borohydride modified with fluorographite. Int. J. Hydrog. Energy 39, 896–904. doi: 10.1016/j.ijhydene.2013.10.109
Zhang, L. T., Zheng, J. G., Xiao, X. Z., Wang, X. C., Huang, X., Liu, M. J., et al. (2017). A new strategy to remarkably improve the low-temperature reversible hydrogen desorption performances of LiBH4 by compositing with fluorographene. Int. J. Hydrog. Energy 42, 20046–20055. doi: 10.1016/j.ijhydene.2017.05.060
Zhao, J. Z., Shi, J. F., Zhang, X. W., Cheng, F. Y., Liang, J., Tao, Z. L., et al. (2010). A soft hydrogen storage material: poly(methylacrylate)-confined ammonia borane with controllable dehydrogenation. Adv. Mater. 22, 394–397. doi: 10.1002/adma.200902174
Zhao, Y. P., Jiao, L. F., Liu, Y. C., Guo, L. J., Li, L., Liu, H. Q., et al. (2014). A synergistic effect between nanoconfinement of carbon aerogels and catalysis of CoNiB nanoparticles on dehydrogenation of LiBH4. Int. J. Hydrog. Energy 39, 917–926. doi: 10.1016/j.ijhydene.2013.10.137
Zhou, Y. F., Liu, Y. F., Wu, W., Zhang, Y., Gao, M. X., and Pan, H. G. (2012). Improved hydrogen storage properties of LiBH4 destabilized by in situ formation of MgH2 and LaH3. J. Phys. Chem. C 116, 1588–1595. doi: 10.1021/jp2101573
Keywords: hydrogen storage materials, LiBH4, melamine foam, flexibility, air-stable
Citation: Fan Y, Chen D, Yuan Z, Chen Q, Fan G, Zhao D and Liu B (2020) Flexible, Water-Resistant and Air-Stable LiBH4 Nanoparticles Loaded Melamine Foam With Improved Dehydrogenation. Front. Chem. 8:45. doi: 10.3389/fchem.2020.00045
Received: 14 November 2019; Accepted: 14 January 2020;
Published: 04 February 2020.
Edited by:
Yongfeng Liu, Zhejiang University, ChinaCopyright © 2020 Fan, Chen, Yuan, Chen, Fan, Zhao and Liu. This is an open-access article distributed under the terms of the Creative Commons Attribution License (CC BY). The use, distribution or reproduction in other forums is permitted, provided the original author(s) and the copyright owner(s) are credited and that the original publication in this journal is cited, in accordance with accepted academic practice. No use, distribution or reproduction is permitted which does not comply with these terms.
*Correspondence: Qiang Chen, Y2hlbnFpYW5nJiN4MDAwNDA7aHB1LmVkdS5jbg==; Baozhong Liu, YnpsaXUmI3gwMDA0MDtocHUuZWR1LmNu