- 1College of Health Science and Environmental Engineering, Shenzhen Technology University, Shenzhen, China
- 2State Key Laboratory for Modification of Chemical Fibers and Polymer Materials, College of Materials Science and Engineering, Donghua University, Shanghai, China
- 3College of Materials Science and Engineering, Changsha University of Science & Technology, Changsha, China
Hollow Co3O4@MnO2 cubic nanomaterials are synthesized by ZIF-67@Mn-ZIF sacrificial precursor through a facile thermal treatment. As a kind of supercapacitor electrode material, it demonstrates high performances, such as specific capacitance of 413 F g−1 at the current density of 0.5 A g−1; as the current densities raised from 0.5 to 10 A g−1 (20 times increasing), there is still ~41% retention of its initial capacitance. These satisfactory electrochemical properties should be put down to the hollow and porous structure and the relative higher BET surface area, which supplies more reactive sites for charge and discharge processes.
Introduction
As a new and efficient energy storage device, supercapacitors qualified the benefits of high-power density, high security, long service life, and fast short time storage and release (El-Kady et al., 2016; Shao et al., 2018; Xu et al., 2018). As a result, supercapacitors attracted wide attention in the application on portable consumer electrical products and electric automobiles, and so on (Qu et al., 2016; Li et al., 2018). The performance of the used electrode materials is the main factor affecting the performance of supercapacitors. Currently, the most studied materials are carbon-based materials (Zhang and Zhao, 2009; Zhang et al., 2017), transition metal oxides (TMOs) (Liu et al., 2011; Li et al., 2014; Yu and Lou, 2018; Xu et al., 2019), and conductive polymer materials (Snook et al., 2011; Du et al., 2017). In recent years, metal-organic frameworks (MOFs) are developed as a new type of porous materials ascribed to their great specific surface area, porosity and regulatory pores, functional and special optical and electrical properties (Yue et al., 2015; Salunkhe et al., 2017). So they have great potential in the high-performance supercapacitor after thermal treatment as TMOs' sacrificial precursor.
Up to the present, numerous TMO nanomaterials have been synthesized as supercapacitor electrodes from many kinds of MOF precursors. For instance, high surface area Co3O4 nanoparticles have been obtained from the pyrolysis of ZIF-67 with an appreciable 190 F g−1 specific capacitance value at 5 A g−1 (Saraf et al., 2019), NiO architecture with porous structure was constructed by thermal treatment Ni-MOF under the air flow and demonstrated 324 F g−1 at 1 A g−1 (Han et al., 2017), and porous hollow α-Fe2O3 microboxes synthesized by using MOF as precursor and self-template can reach 380 F g−1 at 0.1 A g−1 as supercapacitor electrode (Yu et al., 2019). Except for these single metal oxides, some mixed metal oxides, and metal oxide composites can also be obtained by MOF precursors. Chen and coworkers have fabricated porous small size ZnCo2O4 nanoparticles (<20 nm) from a mixed zinc and cobalt-MOF, which exhibited an unexpected specific capacitance of 451 F g−1 at 0.5 mV s−1 (Chen et al., 2015). Hierarchical NiO/ZnO double-shell hollow spheres are obtained by Li and coworkers through calcining the bimetallic organic frameworks, which delivered 497 F g−1 at current density of 1.3 A g−1 (Li et al., 2016). Xu and coworkers developed a Co3O4/ZnO nano-heterostructure via a solid-solid conversion process, the synthesized core-shell MOFs@MOFs were used as a template with cobalt and zinc as metal sources, which demonstrated 415 F g−1 specific capacitance value at 0.5 A g−1 (Xu et al., 2016). The mixed metal oxides and the metal oxide composites as electrodes exhibit superior electrochemical performance compared with single ones. Despite these achievements, there are still large spaces to explore other metal oxide composites based on MOF precursors.
Herein, we have prepared single ZIF-67 nanocrystals first, combined it with Mn-ZIF to form ZIF-67@Mn-ZIF composite, and finally obtained Co3O4@MnO2 electrode material by thermal treatment. After evaluating the electrochemical performance of Co3O4@MnO2 electrode, we found that it exhibited excellent electrochemical properties. When the current density is 0.5 A g−1, the specific capacitance could achieve 413 F g−1, with 20 times current density increasing, it kept 41% retention of initial capacitance and good long-term cycling stability, which is a very promising electrode for use in a supercapacitor.
Experimental
Preparation of ZIF-67
First, 1.455g Co(NO3)2·6H2O and 1.642g 2-methylimidazole were separately dissolved in 40 ml methanol. Second, the two different solutions were mixed and vigorously stirred for 60 s and reacted for 24 h to complete reaction at room temperature after 24 h. Third, the purple precipitates in the bottom were collected by centrifugation with ethanol as washing solution for several times. The collected purple precipitates were dried at 80°C overnight in a vacuum drying chamber.
Preparation of ZIF-67@Mn-ZIF
First, 0.25 g Mn(NO3)2·6H2O was dissolved in 50 ml ethanol. ZIF-67 obtained in the first step was well-dispersed in the above solution. Then, the mixture was transferred into a beaker flask after 20 min of continuous stirring, and the reaction temperature was 50°C and kept for 3 h in an oil bath.
Thermal Treatment of ZIF-67@Mn-ZIF Crystals
The obtained ZIF-67@Mn-ZIF crystals could be converted to Co3O4@MnO2 nanomaterials through a thermal treatment in a tube furnace with air flow at 300°C for 0.5 h; the heating rate was controlled at 0.5°C·min−1. As a contrast experiment, the single precursors (ZIF-67) were calcined under the same thermal conditions, and the final product is Co3O4 nanomaterial.
Material Characterizations
X-ray diffraction (XRD) patterns were measured by using monochromator Cu Kα radiation at a scanning rate of 2°•min−1 (PA-Nalytical X′Pert PRO). Binding energies were detected by the X-ray photoelectron spectroscopy (XPS; ESCALab250). The morphologies were obtained by scanning electron microscope (SEM) (Hitachi, SU-8000). The more detailed structures were investigated by transmission electron microscope (TEM) (JEOL, JEM-2100F), and the elements were detected by its equipped energy dispersive X-ray spectrometer (EDS). The BET surface area and pore size distribution are tested on Accelerated Surface Area & Porosimetry System (ASAP 2020, Micromeritics). XS analytical balance (Mettler Toledo; δ = 0.01 mg) is used to weigh the mass of the electrode materials.
Electrochemical Characterizations
The electrochemical performances of the final products were accomplished by the AUTOLAB PGSTAT302N electrochemical workstation in a standard three-electrode test cell at ~25°C with 1.0 M LiOH solution as electrolyte. The Ag/AgCl (3M KCl) electrode and platinum (Pt) plate (2.5 cm × 2.5 cm × 0.2 mm) directly served as the reference electrode and counter electrode, respectively. The fabricating processes of working electrode were as follows: Co3O4@MnO2 materials (active electrode material, 80%) derived from ZIF-67@Mn-ZIF crystals were mixed with acetylene black (5%) and polyvinylidene difluoride (15%), which was mixed with appropriate volume N-methyl pyrrolidone solvent. The mixture was treated by ultrasonication to form a homogeneous slurry and dropped onto the graphite substrate current collector, the covered surface area is ~1 × 1 cm2, and then dried under vacuum condition at 120°C for 4 h to form the electrodes. For comparison, the Co3O4 materials prepared from single ZIF-67 crystals were also fabricated into electrode with the same processes.
The electrochemical performances of the fabricated electrodes were evaluated from the galvanostatic charge-discharge (GCD) and cyclic voltammetry (CV) measurements. The equation of C = [(I × Δt)/(m × ΔV)] is applied to calculate the specific capacitance values of Co3O4@MnO2 and Co3O4 electrodes, where the I (A), Δt(s), ΔV (V), and m (g) represent the discharge current, the discharge time, the potential window, the mass of active materials in the electrodes, respectively.
Results and Discussions
The synthesized products were analyzed by X-ray diffraction (XRD) first. The result is shown in Figure 1a. As can be seen from the obtained pattern, there are some strong diffraction peaks that appeared in 2θ = 7.3°, 10.4°, 12.8°, 14.8°, 16.5°, 18.1°, which can be confirmed with the sample ZIF-67 and highly consistent with reported literature (Qin et al., 2017). Figures 1b,c are the low to high magnification SEM images. The particles' morphology is uniform rhombic dodecahedral nanocrystals which were clearly monodispersed, with a diameter of about 300–500 nm. Figure 1d shows a single ZIF-67 nanocrystal with dodecahedron and solid construction. After thermal treatment, the structure collapsed (Figure S1).
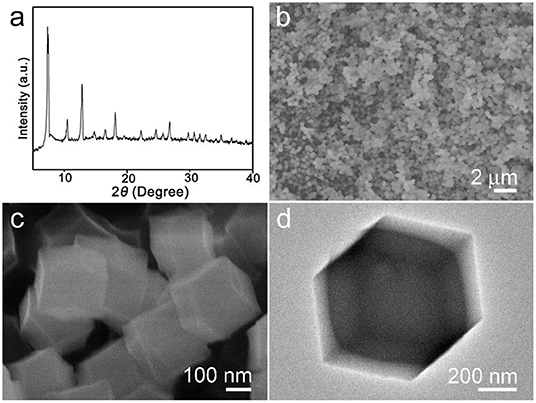
Figure 1. (a) X-ray diffraction (XRD) pattern, (b,c) scanning electron microscope (SEM), and (d) transmission electron microscope (TEM) images of synthesized ZIF-67 sample.
The calcined ZIF-67@Mn-ZIF products were detected by XRD, and the result is shown in Figure 2a. The main diffraction peaks consisted of cubic phase Co3O4 (JCPDS card No. 074-2120), which is obtained by calcinating the ZIF-67 nanocrystal (Figures S2, S3). In addition, there are some other small peaks that also appeared in the pattern (marked with blue star), which could be MnO2 formed by Mn-ZIF (the exact components were detected by XPS, which is detailed later). Figure 2b is a low-resolution SEM image of the obtained Co3O4@MnO2 products, which indicated that the products can be synthesized in large scale. In the enlarged SEM image of Figure 2c, the diameter of obtained Co3O4@MnO2 products increased to about 800 nm; interestingly, the obtained Co3O4@MnO2 products are with hollow structure (Figure 2d), and the corresponding EDS result in Figure 2e is consistent with our designed concept. Co, Mn, and O elements are from Co3O4@MnO2 products, the existence of Cu and C signals is because the TEM grid is made of Cu substrate and carbon membrane, while the peak of Si could be an impurity that brings in the sample preparation process. Inset shows the HRTEM image of the Co3O4@MnO2, the d-spacing of 0.24 nm corresponding to the (311) lattice plane of the Co3O4 crystal, and the d-spacing of 0.22 nm corresponding to the (200) lattice plane of the MnO2 crystal (JCPDS No. 12-0716).
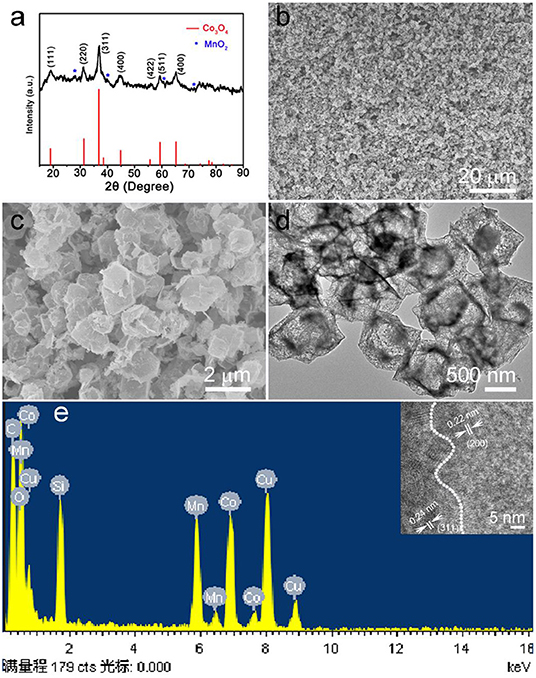
Figure 2. (a) X-ray diffraction (XRD) pattern of the products after calcinating the ZIF-67@Mn-ZIF. (b,c) Low magnification and enlarged scanning electron microscope (SEM) images of the Co3O4@MnO2. (d) Transmission electron microscope (TEM) image and corresponding (e) energy dispersive X-ray spectrometer (EDS) pattern of the Co3O4@MnO2 products. Inset shows the HRTEM image of Co3O4@MnO2.
The obtained calcinated products were further detected by XPS to confirm the metal oxidation states and the chemical compositions. Figure 3A is the survey spectrum of the products, which shows the core levels of Co 2p, Mn 2p, and O 1s, respectively. To get clearer information, the high-resolution XPS spectra analysis was carried out. The Co 2p's high-resolution XPS spectrum is shown in Figure 3B. The main two peaks centered at 780.3 and 796.2 eV can be appointed to the binding energies of 2p3/2 and 2p1/2 of Co(II), whereas the other two lower peaks centered at 786.1 and 802.5 eV can be appointed to the binding energies of 2p3/2 and 2p1/2 of Co(III). These results imply the Co3O4 phase in our sample and agreement with the XRD result (Yan et al., 2012; Li et al., 2013). Figure 3C is the high-resolution XPS spectrum extracted from Mn 2p. The main two peaks are centered at 641.1 and 652.7 eV; therefore, the spin-orbital splitting calculated is 11.6 eV. These results well refer to the electronic orbits of Mn 2p3/2 and 2p1/2, pointing to Mn(IV) state of the products (Sui et al., 2009). As can be seen from the high-resolution spectrum of O 1s in Figure 3D, there are two distinct components, except for the binding energy of 531.2 eV assigned to the oxygen atoms in the hydroxyl groups, the strong peak of 529.6 eV should belong to the oxygen atoms in the chemical compositions of Co3O4 and MnO2 (Wei et al., 2008; Xia et al., 2010). These results further proved that the chemical component of as-fabricated products is Co3O4@MnO2.
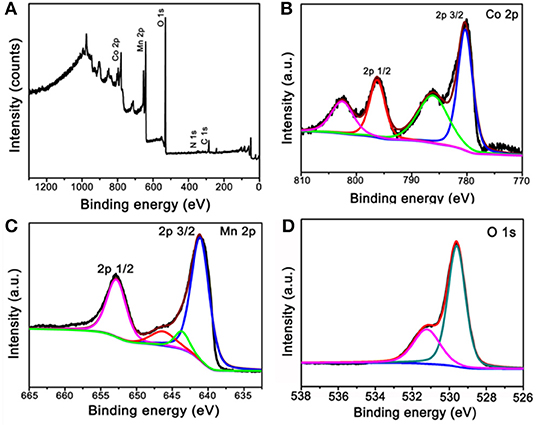
Figure 3. X-ray photoelectron spectroscopy (XPS) spectra of the products after calcinating the ZIF-67@Mn-ZIF. (A) Survey XPS spectrum and (B–D) high-resolution XPS spectra of Co 2p, Mn 2p, and O 1s.
A typical IV type adsorption behavior was observed in the prepared Co3O4 and Co3O4@MnO2 products by the N2 adsorption-desorption isotherms (Figures 4A,B), which exhibit a mesoporous structure with slit type pores. The BET surface areas for the Co3O4 and Co3O4@MnO2 are 72.214 and 148.407 m2 g−1, a high BET surface area might be beneficial for the electrons and ions' storage and shuttle in the electrode because it provides more active sites, hence could lead to enhanced electrochemical capacity (Jiang et al., 2012). From the corresponding pore size distributions of the inset image, it can be found that the pore sizes are concentrated in 3–10 nm for Co3O4 sample and 3–6 nm for Co3O4@MnO2 sample. The porous structure is facilitating the electrolyte ion diffusion and transference in the course of charge and discharge processes.
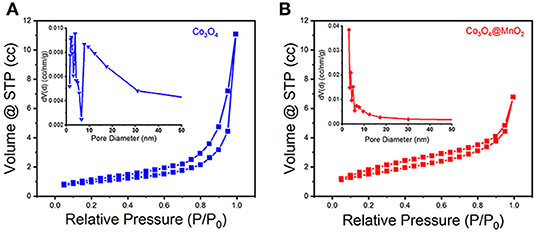
Figure 4. N2 adsorption-desorption isotherms of (A) Co3O4 and (B) Co3O4@MnO2 products. Inset shows their corresponding pore size distributions.
The electrochemical properties of the Co3O4@MnO2 electrode materials were evaluated, and the results are summarized and shown in Figure 5. Enclosed loops in Figure 5A show the electrode's CV performance at increasing scan rates from 1 to 100 mV s−1, unlike the pseudocapacitance behavior of single Co3O4 nanocrystals (Figure S4). The shapes of the CV curves of Co3O4@MnO2 indicate a typical electrical double layer capacitance (EDLC) behavior, and it retains well as the scan rate upscales to 20 mV s−1, demonstrating its good rate capability (Wu et al., 2015). The EDLC behavior of Co3O4@MnO2 ascribes to the MnO2 outer layer (Li et al., 2012). The slight shape deformation was observed when the scan rate achieves 50 and 100 mV s−1; this could be ascribed to the polarization phenomenon at high scan rate (Salanne et al., 2016). The GCD properties were evaluated at current densities from 0.5 A g−1 and extended to 10 A g−1 in the voltage range from 0 to 0.6 V vs. Ag/AgCl (3M KCl). In Figure 5B, it is clear to observe a series of good symmetric triangle shape GCD curves, revealing its good EDLC behavior; this result is consistent with CV performance. Under a series of current densities, that is, 0.5, 1, 2, 4, 6, 8, and 10 A g−1, the specific capacitances were calculated to be 413, 370, 324, 273, 233, 200, and 168 F g−1, respectively. On the contrary, single Co3O4 nanocrystal only delivers 187, 155, 108, 76, 57, and 45 F g−1 at 1, 2, 4, 6, 8, and 10 A g−1, respectively (Figure S5). Obviously, the Co3O4@MnO2 electrode presents better capacitance values than single Co3O4 electrode, the reason could be owing to the multicomponent and higher BET surface of Co3O4@MnO2 electrode that endows the more charge storage (Jiang et al., 2012). Under the extending current densities from 0.5 to 10 A g−1, the specific capacitance decreased from 413 to 168 F g−1, retaining ~41% of its initial capacitance (shown in Figure 5C). While for single Co3O4 nanocrystal, the rate capability is only 25% from 1 to 10 A g−1 (187 vs. 45 F g−1, Figure S6). After 2,000 times cycles of CV test at 20 mV s−1, the capacitance retention remains at 110% (shown in Figure 5D), while only 80% of single Co3O4 nanocrystal (Figure S7), indicating a good stability of Co3O4@MnO2 electrode. It is clear to conclude that the performance of Co3O4@MnO2 in connection with capacitance retention and cycling ability is much improved compared with single Co3O4.
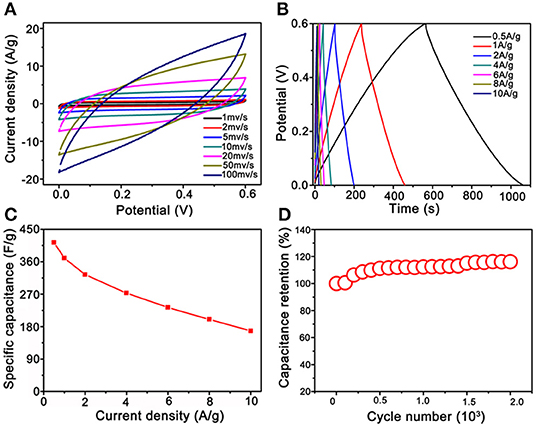
Figure 5. Electrochemical test results of Co3O4@MnO2 electrode. (A) Cyclic voltammetry (CV) curves at extending scan rates from 1 to 100 mV s−1. (B) CD curves under extending current densities from 0.5 to 10 A g−1. (C) Plot of the specific capacitance against different current densities. (D) Long-term cycling stability.
Conclusions
In conclusion, hollow Co3O4@MnO2 cubic nanomaterials were synthesized by sacrificing the ZIF-67@Mn-ZIF precursor through an uncomplicated controlled thermal treatment. The porous structure and high BET surface area endow its excellent properties as supercapacitor electrode, it presented a high specific capacitance of 413 F g−1 (0.5 A g−1) and showed a rate capability of 41% at the current density enhanced to 20 times with excellent stability, giving the impression that this hollow cubic nanomaterial possesses considerable potential as a supercapacitor electrode material.
Data Availability Statement
The XRD datasets generated for this study can be found in the repository of ICDD, with the accession numbers of 74-2120 and 12-0716 in JCPDS card.
Author Contributions
JW and JH conceived and designed the experiments. JX, YZ, and CX performed the experiments and analyzed the data. All authors revised and checked the draft.
Funding
This research was supported by the National Natural Science Foundation of China (51701022, 51972055), the Shenzhen Science and Technology Research Project (Grant No. JCYJ20180508152903208), and the Shenzhen Pengcheng Scholar Program.
Conflict of Interest
The authors declare that the research was conducted in the absence of any commercial or financial relationships that could be construed as a potential conflict of interest.
Supplementary Material
The Supplementary Material for this article can be found online at: https://www.frontiersin.org/articles/10.3389/fchem.2019.00831/full#supplementary-material
References
Chen, S., Xue, M., Li, Y. Q., Pan, Y., Zhu, L. K., Zhang, D. L., et al. (2015). Porous ZnCo2O4 nanoparticles derived from a new mixed-metal organic framework for supercapacitors. Inorg. Chem. Front. 2, 177–183. doi: 10.1039/C4QI00167B
Du, X., Zhang, Z., Liu, W., and Deng, Y. L. (2017). Nanocellulose-based conductive materials and their emerging applications in energy devices - A review. Nano Energy 35, 299–320. doi: 10.1016/j.nanoen.2017.04.001
El-Kady, M. F., Shao, Y., and Kaner, R. B. (2016). Graphene for batteries, supercapacitors and beyond. Nat. Rev. Mater. 1:16033. doi: 10.1038/natrevmats.2016.33
Han, Y., Zhang, S., Shen, N., Li, D. J., and Li, X. F. (2017). MOF-derived porous NiO nanoparticle architecture for high performance supercapacitors. Mater. Lett. 188, 1–4. doi: 10.1016/j.matlet.2016.09.051
Jiang, H., Sun, T., Li, C., and Ma, J. (2012). Hierarchical porous nanostructures assembled from ultrathin MnO2 nanoflakes with enhanced supercapacitive performances. J. Mater. Chem. 22, 2751- 2756. doi: 10.1039/C1JM14732C
Li, G. C., Liu, P. F., Liu, R., Liu, M. M., Tao, K., Zhu, S. R., et al. (2016). MOF-derived hierarchical double-shelled NiO/ZnO hollow spheres for high-performance supercapacitors. Dalton Trans. 45, 13311–13316. doi: 10.1039/C6DT01791F
Li, W., Li, G., Sun, J., Zou, R., Xu, K., Sun, Y., et al. (2013). Hierarchical heterostructures of MnO2 nanosheets or nanorods grown on Au-coated Co3O4 porous nanowalls for high-performance pseudocapacitance. Nanoscale 5, 2901–2908. doi: 10.1039/c3nr34140b
Li, W., Zhang, B., Lin, R., Ho-Kimura, S., He, G., Zhou, X., et al. (2018). A dendritic nickel cobalt sulfide nanostructure for alkaline battery electrodes. Adv. Funct. Mater. 28:1705937. doi: 10.1002/adfm.201705937
Li, W. Y., Liu, Q., Sun, Y. G., Sun, J. Q., Zou, R. J., Li, G., et al. (2012). MnO2 ultralong nanowires with better electrical conductivity and enhanced supercapacitor performances. J. Mater. Chem. 22, 14864–14867. doi: 10.1039/c2jm33368f
Li, W. Y., Xu, K. B., An, L., Jiang, F. R., Zhou, X. Y., Yang, J. M., et al. (2014). Effect of temperature on the performance of ultrafine MnO2 nanobelt supercapacitors. J. Mater. Chem. A 2, 1443–1447. doi: 10.1039/C3TA14182A
Liu, J., Jiang, J., Cheng, C., Li, H., Zhang, J., Gong, H., et al. (2011). Co3O4 Nanowire@MnO2 ultrathin nanosheet core/shell arrays: a new class of high-performance pseudocapacitive materials. Adv. Mater. 23, 2076–2081. doi: 10.1002/adma.201100058
Qin, J. N., Wang, S. B., and Wang, X. C. (2017). Visible-light reduction CO2 with dodecahedral zeolitic imidazolate framework ZIF-67 as an efficient co-catalyst. Appl. Catal. B Environ. 209, 476–482. doi: 10.1016/j.apcatb.2017.03.018
Qu, G., Cheng, J., Li, X., Yuan, D., Chen, P., Chen, X., et al. (2016). A fiber supercapacitor with high energy density based on hollow graphene/conducting polymer fiber electrode. Adv. Mater. 28, 3646–3652. doi: 10.1002/adma.201600689
Salanne, M., Rotenberg, B., Naoi, K., Kaneko, K., Taberna, P. L., Grey, C. P., et al. (2016). Efficient storage mechanisms for building better supercapacitors. Nat. Energy 1:16070. doi: 10.1038/nenergy.2016.70
Salunkhe, R. R., Kaneti, Y. V., and Yamauchi, Y. (2017). Metal-organic framework-derived nanoporous metal oxides toward supercapacitor applications: progress and prospects. ACS Nano 11, 5293–5308. doi: 10.1021/acsnano.7b02796
Saraf, M., Rajak, R., and Mobin, S. M. (2019). MOF derived high surface area enabled porous Co3O4 nanoparticles for supercapacitors. ChemistrySelect 4, 8142–8149. doi: 10.1002/slct.201901652
Shao, Y., El-Kady, M. F., Sun, J., Li, Y., Zhang, Q., Zhu, M., et al. (2018). Design and mechanisms of asymmetric supercapacitors. Chem. Rev. 118, 9233–9280. doi: 10.1021/acs.chemrev.8b00252
Snook, G. A., Kao, P., and Best, A. S. (2011). Conducting-polymer-based supercapacitor devices and electrodes. J. Power Sources 196, 1–12. doi: 10.1016/j.jpowsour.2010.06.084
Sui, N., Duan, Y. Z., Jiao, X. L., and Chen, D. R. (2009). Large-scale preparation and catalytic properties of one-dimensional alpha/beta-MnO2 nanostructures. J. Phys. Chem. C 113, 8560–8565. doi: 10.1021/jp810452k
Wei, W. F., Cui, X. W., Chen, W. X., and Ivey, D. G. (2008). Phase-controlled synthesis of MnO2 nanocrystals by anodic electrodeposition: implications for high-rate capability electrochemical supercapacitors. J. Phys. Chem. C 112, 15075–15083. doi: 10.1021/jp804044s
Wu, J., Ouyang, C., Dou, S., and Wang, S. (2015). Hybrid NiS/CoO mesoporous nanosheet arrays on Ni foam for high-rate supercapacitors. Nanotechnology 26:325401. doi: 10.1088/0957-4484/26/32/325401
Xia, H., Feng, J. K., Wang, H. L., Lai, M. O., and Lu, L. (2010). MnO2 nanotube and nanowire arrays by electrochemical deposition for supercapacitors. J. Power Sources 195, 4410–4413. doi: 10.1016/j.jpowsour.2010.01.075
Xu, J., Liu, S. C., and Liu, Y. (2016). Co3O4/ZnO nanoheterostructure derived from core-shell ZIF-8@ZIF-67 for supercapacitors. RSC Adv. 6, 52137–52142. doi: 10.1039/C6RA07773K
Xu, K., Li, S., Yang, J., and Hu, J. (2018). Hierarchical hollow MnO2 nanofibers with enhanced supercapacitor performance. J. Colloid Interface Sci. 513, 448–454. doi: 10.1016/j.jcis.2017.11.052
Xu, K., Shen, Y., Zhang, K., Yang, F., Li, S., and Hu, J. (2019). Hierarchical assembly of manganese dioxide nanosheets on one-dimensional titanium nitride nanofibers for high-performance supercapacitors. J. Colloid Interface Sci. 552, 712–718. doi: 10.1016/j.jcis.2019.05.093
Yan, N., Hu, L., Li, Y., Wang, Y., Zhong, H., Hu, X. Y., et al. (2012). Co3O4 nanocages for high-performance anode material in lithium-ion batteries. J. Phys. Chem. C 116, 7227–7235. doi: 10.1021/jp2126009
Yu, X. Y., and Lou, X. W. (2018). Mixed metal sulfides for electrochemical energy storage and conversion. Adv. Energy Mater. 8:1701592. doi: 10.1002/aenm.201701592
Yu, Z. Y., Zhang, X. Y., Wei, L., and Guo, X. (2019). MOF-derived porous hollow alpha-Fe2O3 microboxes modified by silver nanoclusters for enhanced pseudocapacitive storage. Appl. Surf. Sci. 463, 616–625. doi: 10.1016/j.apsusc.2018.08.262
Yue, Y., Fulvio, P. F., and Dai, S. (2015). Hierarchical metal-organic framework hybrids: perturbation-assisted nanofusion synthesis. Acc. Chem. Res. 48, 3044–3052. doi: 10.1021/acs.accounts.5b00349
Zhang, L. L., and Zhao, X. S. (2009). Carbon-based materials as supercapacitor electrodes. Chem. Soc. Rev. 38, 2520–2531. doi: 10.1039/b813846j
Keywords: metal-organic frameworks, ZIF-67, Mn-ZIF, Co3O4@MnO2, supercapacitors
Citation: Xu J, Xu C, Zhao Y, Wu J and Hu J (2019) Hollow Co3O4@MnO2 Cubic Derived From ZIF-67@Mn-ZIF as Electrode Materials for Supercapacitors. Front. Chem. 7:831. doi: 10.3389/fchem.2019.00831
Received: 27 October 2019; Accepted: 14 November 2019;
Published: 13 December 2019.
Edited by:
Min Zeng, Lanzhou Institute of Chemical Physics (CAS), ChinaReviewed by:
Shijie Li, Zhejiang Ocean University, ChinaHui Yang, Jiangxi University of Science and Technology, China
Copyright © 2019 Xu, Xu, Zhao, Wu and Hu. This is an open-access article distributed under the terms of the Creative Commons Attribution License (CC BY). The use, distribution or reproduction in other forums is permitted, provided the original author(s) and the copyright owner(s) are credited and that the original publication in this journal is cited, in accordance with accepted academic practice. No use, distribution or reproduction is permitted which does not comply with these terms.
*Correspondence: Jianghong Wu, d3VqaWFuZ2hvbmcmI3gwMDA0MDtzenR1LmVkdS5jbg==