- 1Chair of Food Packaging Technology, TUM School of Life Sciences Weihenstephan, Technical University of Munich, Freising, Germany
- 2Fraunhofer Institute for Process Engineering and Packaging IVV, Freising, Germany
- 3Department of Food Engineering, Istanbul Technical University, Istanbul, Turkey
Oxygen scavengers based on gallic acid (GA) usually contain a base that establishes the alkaline conditions necessary for the humidity-induced scavenger reaction. Here we measured the effect of sodium carbonate (Na2CO3) and sodium hydroxide (NaOH) on the reaction kinetics of such scavengers. The time-dependent oxygen absorption of aqueous GA solutions (pH 2.9–13.8) was determined and the results were described using a second-order kinetic model. We calculated the degree of deprotonation (DoD) of GA in the solutions from the species distribution curves, and assessed the effect of the DoD on the reaction kinetics. This revealed that both the reaction rate coefficient k and the scavenger capacity n were significantly affected by the DoD. If the DoD fell below 0.25, there was no significant reaction. Although k increased with the DoD, n reached a maximum at DoD = 0.6–0.7. In principle, target DoD values can be achieved using any base, but a strong base is more efficient because lower quantities are required. In our experiments, the amount of Na2CO3 required to reach the maximum DoD was more than twice that of NaOH. Our results provide the basis for the functional design of active packaging systems incorporating optimized GA-based oxygen scavengers.
1. Introduction
Gallic acid (GA), also known as 3,4,5-trihydroxybenzoic acid, is a natural polyphenol found in many plants either as a free acid or as a component of tannins (Shahidi and Naczk, 2003; Belitz and Grosch, 2013). When combined with a base, GA can be used as an oxygen (O2) scavenger for packaging applications, preventing the oxidation of sensitive food products (Langowski and Wanner, 2005). O2 scavengers based on GA (e.g., a mixture of GA and sodium carbonate) have a high O2 absorption capacity and can be integrated into packaging films (Ahn et al., 2016; Pant et al., 2017). Depending on the film structure, the scavenger can either improve the barrier function of the packaging material or absorb residual O2 from the headspace. The scavenger function is activated by humidity derived from the product or in the environment (Wanner, 2010).
Chemically, the O2 scavenging function of GA-based O2 scavengers relies on the autoxidation of GA in alkaline solution. GA is a weak polyprotic acid (Figure 1) with four acidic protons that can be transferred to an acceptor base.
The solution pH determines the species distribution of GA, i.e., the proportions of GA and the different gallate anions present in the solution, and therefore also the type of GA radicals formed during autoxidation. For autoxidation at high pH Yoshioka et al. (2003) described radical formation by single electron transfer (SET) from the gallate anion to a dissolved O2 molecule (Figure 1). The by-product hydrogen peroxide (H2O2) undergoes homolysis to form two hydroxyl radicals when exposed to UV radiation from ambient sunlight (Holleman, 2007). Alternatively, metal ions such as Fe2+, which could be present as impurities, can be oxidized by H2O2 to form a hydroxyl radical and a hydroxyl anion (Walling, 1975). The hydroxyl radicals may in turn directly withdraw a hydrogen atom from GA in aqueous solution by hydrogen atom transfer (HAT) (Milenkovic et al., 2018). A HAT reaction at pH 9 is shown as an example in Figure 2.
Both SET and HAT can lead to the formation of gallate radicals, which has been confirmed by electron spin resonance spectroscopy and shown in theoretical models (Yoshioka et al., 2003; Severino et al., 2009; Eslami et al., 2010). These gallate radicals can take part in further reactions. Tulyathan et al. (1989) proposed a multi-step oxidation mechanism including a ring-opening step, but did not report any qualitative or quantitative analysis of the reaction products. Hotta et al. (2001) proposed an oxidative polymerization mechanism in an alkaline environment in order to explain the unusually high number of transferred electrons compared to the number of hydroxyl groups. More recently some of the intermediates in this radical-induced polymerization pathway have been identified: C-O dimers (Oniki and Takahama, 2004), C-C dimers (Caregnato et al., 2008) and ellagic acid (Nikolic et al., 2011) (Figure 3).
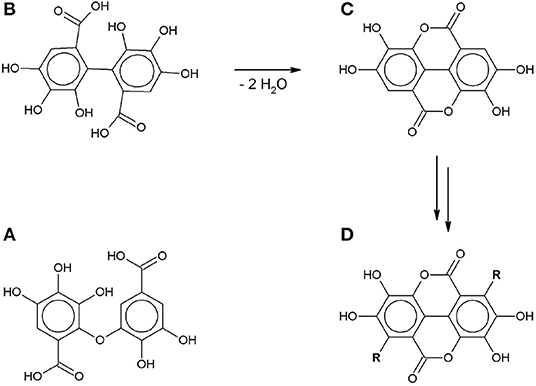
Figure 3. Gallic acid autoxidation intermediates: (A) C-O dimer (Oniki and Takahama, 2004). (B) C-C dimer (Caregnato et al., 2008). (C) Ellagic acid (Nikolic et al., 2011). (D) gallate-based polymer (R: gallate-derived group).
An autoxidation that generates dark-colored reaction products has been described for several phenolic compounds (Cilliers and Singleton, 1989; Friedman and Juergens, 2000). Although the complete reaction pathway and the oxidation products have not yet been identified, the type and quantity of oxidation products changes with pH (Cilliers and Singleton, 1989; Friedman and Juergens, 2000). Wanner (2010) reported that a pH higher than 8 is necessary to enable the GA scavenger reaction and that the type of base used determined the reaction kinetics. Tulyathan et al. (1989) investigated the O2 absorption capacity of GA in the pH range 10–14 and observed a maximum first-order reaction rate constant at pH 12. For the polyphenol caffeic acid Cilliers and Singleton (1989) studied O2 absorption in the pH range 4–8 and reported increasing reaction rate constants at higher pH values.
Considering these results, the pH-dependent deprotonation of GA is likely to play a key role in selecting the autoxidation reaction and therefore the scavenger performance.
We previously used a 2:1 mass ratio GA:sodium carbonate as an O2 scavenger for packaging applications and investigated the effect of temperature and relative humidity on the second-order reaction rate constant and absorption capacity (Pant et al., 2018). However, it remains unclear which type of base and which mixing ratio achieves optimal oxygen scavenger activity.
Therefore, the aim of this work was to study the effect of the type of base on the oxygen scavenger kinetics of GA. The autoxidation of GA was studied in aqueous solutions adjusted to different pH values by adding either NaOH or Na2CO3. The second-order reaction rate coefficent and the absorption capacity were determined as well as their dependence on the deprotonation of GA.
2. Materials and Methods
2.1. Materials
Gallic acid monohydrate (GA, 99%) was obtained from ABCR (Karlsruhe, Germany). Water-free sodium carbonate (Na2CO3, 99.8%) and sodium hydroxide (NaOH, 98.8%) were obtained from Th.Geyer (Renningen, Germany).
To prepare aqueous GA solutions at different pH values, samples of 0.040 g GA powder and the appropriate amount of base were mixed with 5 mL destilled water in a Petri dish at 23 °C. Solutions were prepared with NaOH at pH 5.7, 7.9, 9.1, 11.1, 13.0, 13.8. Solutions were prepared with Na2CO3 at pH 5.1, 7.7, 8.3, 9.3, 10.4. The pH meter was calibrated with standard solutions at pH 7.0, 9.0, and 11.0. A GA solution without added base (pH = 2.9) was used as a reference.
2.2. Modeling the Acid-Base System
An aqueous solution of GA includes several acid-base equilibria, each characterized by its corresponding equilibrium constant K, as shown in Equations (1)–(5).
where H4A, , , HA3−, and A4− denote the GA species with 4, 3, 2, 1, and 0 acidic protons, respectively. Equations (1)–(4) describe the GA deprotonation equilibria characterized by the equilibrium constants pKa1 = 4.39 which is related to the carboxylic proton and pKa2 = 8.50, pKa3 = 10.38, pKa4 = 13 (Eslami et al., 2010; Jabbari, 2015), which are related to the phenolic hydroxy groups. Equation (5) shows the ion product of water, .
The acid-base system is further characterized by the mass balance of the GA species (Equation 6) and the charge balance (Equation 7):
where cA is the initial GA concentration and [A4−], [HA3−], , , [H4A], [H3O+], [OH−], [Na+] are the equilibrium concentrations of the different GA species and the hydronium, hydroxide and sodium ions, respectively.
The system of Equations (1)–(7) was solved in MATLAB R2014a (The MathWorks, Inc., Natick, MA, USA) using the fsolve function. The pH (i.e., the concentration of ), was varied systematically to obtain the pH-dependent distribution of the GA species. From this species distribution, the degree of deprotonation (DoD) was calculated according to Equation (8):
where total acidic H+ refers to the total amount of acidic H+ available from the GA molecules in the solution, and donated H+ refers to the total amount of acidic H+ transferred to the solution at a certain pH.
If Na2CO3, a biprotic base, is added to the solution, additional equilibria have to be considered as shown in Equations (9) and (10).
where and denote the bicarbonate and carbonate ions, respectively. accounts for both carbonic acid and dissolved CO2. The equilibrium constants we used were pKb1 = 10.3 and pKb2 = 6.3 (Lower, 1999).
2.3. Measurement of Oxygen Absorption
The absorption of O2 by the aqueous GA solutions was measured in hermetically closed stainless-steel cells with a free headspace volume of 88 cm3 (Figure 4). The Petri dishes containing the GA solutions were placed in the cells and their O2 uptake from the headspace (initial gas atmosphere: air) was monitored for 30 days at 23°C using Fibox 4 Trace, a luminescence-based O2 detection system (PreSens Precision Sensing GmbH, Regensburg, Germany). To minimize the effect of O2 transport limits in the solution, the Petri dishes were only partially filled.
The pO2 absorption data in hPa were converted to concentrations cO2 using the ideal gas law (Equation 11).
where nO2 is the amount of substance of O2, VHS is the headspace volume of the cell, R = 8.314 J mol−1 K−1 is the ideal gas constant, and T is the temperature in Kelvin.
Each experiment was carried out at least three times and the results are presented as the arithmetic mean with standard deviations.
The mean square error (MSEexp) of the observed O2 concentrations was calculated as shown in Equation (12):
where N is the total number of observations, m is the number of parallel experiments, q the number of observations in one experiment, [O2]exp the observed O2 concentration and [O2]mean the arithmetic mean of all jth observations of the m experiments.
2.4. Modeling the Oxidation Kinetics
The autoxidation of gallic acid can be described by the simplified net reaction shown in Equation (13) (Pant et al., 2018):
where GA represents all non-oxidized GA species, n is a stoichiometric factor and GAox accounts for the various reaction products. This simplified, application-oriented approach is based on the following assumptions: (i) we do not differentiate between the different GA species; (ii) the oxygen uptake is irreversible; and (iii) all oxidation products are summarized as GAox.
The kinetic law of this reaction was approximated as a second-order elementary reaction and the reaction rate r was expressed as shown in Equation (14):
where [GA] and [O2] denote the concentrations of GA and O2, respectively [mol m−3] and k is the reaction rate coefficient [m3 mol−1 s−1]. Based on this kinetic law, the net consumption rates of GA and O2 were described with the system of ordinary differential equations (ODEs) shown as Equations (15) and (16)
The ODE system was solved in MATLAB R2014a using the multistep solver ode15s. The model was fitted to the experimental data to determine the kinetic parameters k and n. The fit was optimized using a downhill simplex method as described by Pant and Reinelt (2018).
3. Results and Discussion
3.1. Deprotonation of Gallic Acid in Aqueous Solution
The acid-base system studied in the oxidation experiments (i.e., an aqueous solution of GA) was described using Equations (1)–(10). GA is present as a range of deprotonated GA species that depend on the pH of the solution. The equilibrium concentrations of the different GA species at a given pH are determined by the acidity constants of the acidic protons. Figure 5 shows the simulated pH-dependent species distribution for aqueous GA solutions. When the pH is low, H4A is the dominant species, representing a GA molecule with four protons. As the pH increases, the relative proportions of the species change until almost all protons are donated at pH 14. The species distribution was used to calculate the DoD as a measure of the average deprotonation of GA (Figure 5). The DoD increases in line with higher pH values and almost complete deprotonation is achieved at pH~14. At pH 9.5 the DoD is 0.5, indicating that the GA molecules have donated an average of two acidic protons.
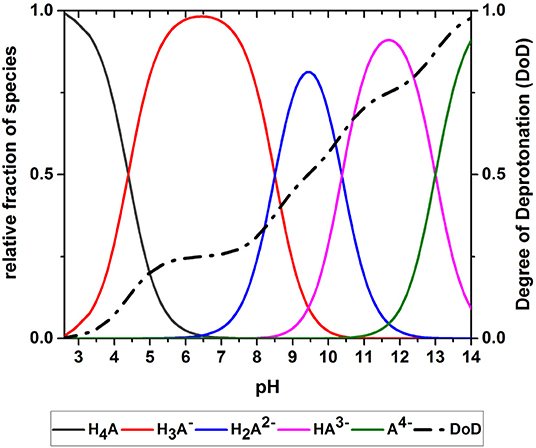
Figure 5. Species distribution of an aqueous gallic acid solution allowing the calculation of the degree of deprotonation (DoD).
Figure 6 shows how much NaOH or Na2CO3 is necessary to achieve a certain DoD according to the simulation. Due to the different strength of the two bases, the amounts differ significantly and the difference in the quantity of each base required becomes larger as the DoD increases. For example, to achieve a DoD of 0.75 using Na2CO3, base:GA weight ratio is 2, but this falls to only 0.8 when using NaOH.
3.2. Effect of pH on Oxygen Absorption
In packaging materials, GA-based O2 scavengers can absorb O2 if water is present, i.e., in aqueous solutions comprising GA and a base. We assessed the effect of the base on the ability of GA to absorb O2 in solutions at different pH values, prepared by adding either NaOH (Figure 7) or Na2CO3 (Figure 8). Progress was monitored over 30 days of storage at 23°C.
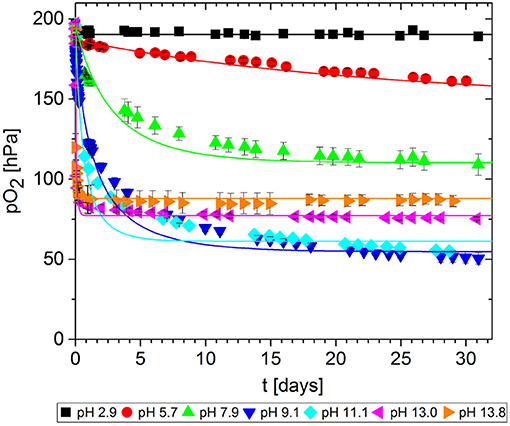
Figure 7. Oxygen absorption by aqueous solutions of gallic acid and NaOH (5 mL) at 23°C. Symbols shows the experimental data and lines show the simulation results.
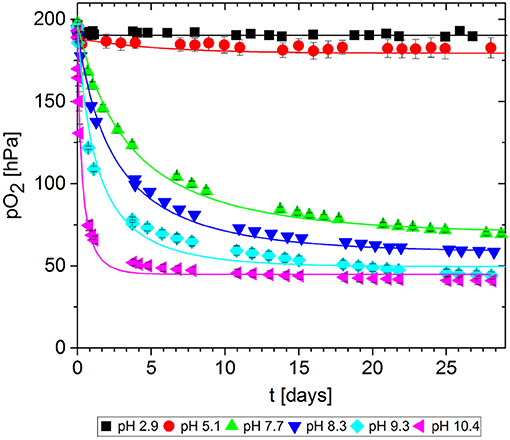
Figure 8. Oxygen absorption by aqueous solutions of gallic acid and Na2CO3 (5 mL) at 23°C. Symbols show the experimental data and lines show the simulation results.
The reference solution with no base added (pH = 2.9) did not absorb any O2. Regardless of which base was added, the more alkaline solutions generally absorbed greater quantities of O2 by promoting faster O2 uptake and thus more O2 absorption in total after 30 days. However, for NaOH solutions, the O2 absorption capacity peaked at ~10 and dropped again. Accordingly, the absorption capacity of GA at pH 11.1. was almost equal to that at pH 9.1, and the capacity was lower at pH 13.0 and 13.8. Tulyathan et al. (1989) also reported an O2 absorption peak but at slightly higher pH. In the case of Na2CO3, it was not possible to prepare solutions with a pH higher than 10.4 due to the buffer effect of the - system (pKb1 = 10.3): The necessary quantities to achieve higher pH values would not dissolve in the GA solution.
The experiment was stopped after 30 days, but not all samples reached their maximum absorption capacity during this period because the scavenging reaction was too slow. The observed differences in O2 absorption must reflect a pH-dependent effect on the mechanism of the oxidation reaction, leading to different ways of incorporating O2 and thereby to different oxidation products. This was confirmed by the color development of the samples. Figure 9 shows samples from the solutions at pH 7.2, pH 11.1 and pH 13.0 after 1 day and after 30 days of storage. Initially (t = 0), the solutions were almost colorless with a slight yellow tint. Between days 1 and 30, the solutions at pH 7.2 and 11.1 changed from green to brown, whereas the solution at pH 13.0 change from organge to yellow. For autoxidation experiments in the pH range 2.6–11, Tóth et al. (2014) reported a similar profile of long-term color development. This qualitative observation supports previous reports that different polymerization products are formed during the autoxidation of GA in alkaline solutions (Cilliers and Singleton, 1989; Tulyathan et al., 1989; Hotta et al., 2001; Tóth et al., 2014). Further studies should be carried out to investigate the oxidation mechanism in more detail, including the effect of traces of metal ions on the reaction.
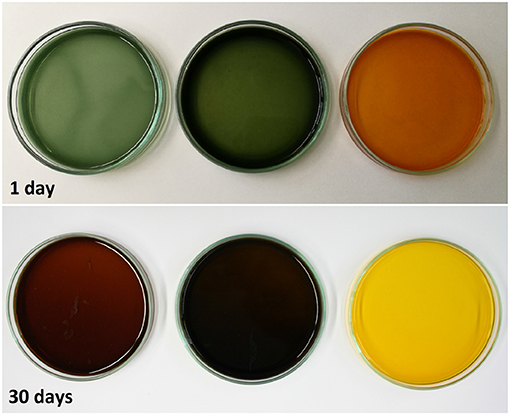
Figure 9. Color evolution of gallic acid solutions with pH 7.2, pH 11.1, and pH 13.0 (left to right).
The O2 absorption measurements clearly demonstrated that pH has an effect on scavenger kinetics. However, the pH only provides information about conditions external to the reaction and, strictly speaking, the concept of pH can only be used to describe dilute aqueous solutions. For the design of O2 scavenger mixtures for packaging applications, it therefore seems more reasonable to describe the autoxidation process as a function of an intrinsic property of GA, the DoD.
The kinetic parameters k and n were determined for each experimental dataset by fitting a second-order kinetic model (Table 1). For most experiments, the simulation results were in good agreement with the empirical data: the root-mean-square error (RMSE) was in the same order of magnitude as the experimental error MSEexp. The fitted curves are shown in Figures 7, 8. These results show that the simple second-order model can be used to describe the O2 uptake by GA under diverse conditions.
Figure 10 shows the effect of the DoD on the reaction rate constant k and the absorption capacity n in the presence of each base. We found that k increased in line with the DoD, but that n reached a maximum at a DoD value of 0.6–0.7 equating to a pH of 10–11 under our experimental conditions. When the DoD fell below 0.2–0.3 there was no significant O2 absorption. At a DoD value of 0.6–0.7 the predominant species in the solution are HA3− and (Figure 5). These species can participate in an autoxidation reaction involving an SET step as described in Figure 1. Our results indicate that this pathway may be preferred in terms of achieving a maximum absorption capacity. In contrast, autoxidation based on A4− is likely to involve a different reaction pathway leading to a lower absorption capacity.
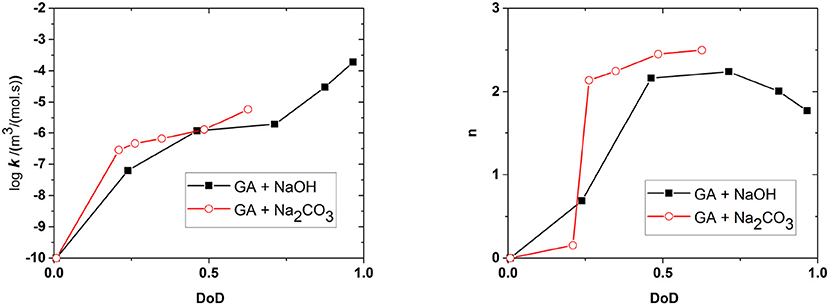
Figure 10. Effect of the degree of deprotonation (DoD) on the reaction rate coefficient k and absorption capacity n. Connecting lines are shown for clarity.
Figure 6 shows the amounts of NaOH and Na2CO3 required to achieve a certain DoD in the solution and this relationship is the basis for the development of an optimized scavenger composition. For maximum O2 scavenger capacity, the DoD should be 0.6–0.7. This requires a base:GA mass ration of ~1.8:1 for Na2CO3 and ~0.8:1 for NaOH. As expected, a much smaller amount of the strong base NaOH is required to reach any given DoD compared to the weaker base Na2CO3. In terms of the design and processing of packaging materials incorporating scavengers based on GA, the amount of base required is important in terms of the precessability of the packaging material and the cost efficiency of the process.
4. Conclusion
In this study, we found that the amount and type of base added to an aqueous GA solution changes the O2 absorption properties of the system in terms of the reaction rate constant and also the maximum absorption capacity. The addition of a base changes the acid-base equilibrium of the solution and therefore the type and amount of GA species that are present, which in turn influences the autoxidation reaction and ultimately the absorption of O2. The key property that must be targeted when designing O2 scavengers based on GA is therefore the DoD. We found that no reaction occurred at DoD values below 0.25. The absorption capacity n reaches its maximum value when the DoD is 0.6–0.7 whereas the reaction rate constant k increases with the DoD. Accordingly, the optimum DoD can be achieved by adding either NaOH or Na2CO3, but a strong base is more efficient because a lower quantity is required to reach the desired DoD value. These results, together with the models describing the GA solution and the autoxidation reaction, provide guidance for the development of packaging systems incorporating O2 scavengers based on GA.
Data Availability Statement
The datasets generated for this study are available on request to the corresponding author.
Author Contributions
AP conceived and designed the experiments, performed some of the oxygen absorption measurements, conducted the simulations and parameter estimations, and wrote the paper. DÖ contributed to the experimental design and performed some of the oxygen absorption measurements. SF contributed to the analysis and interpretation of the results and helped to write and revise the paper. MR contributed to the experimental design as well as modeling and simulation techniques and revised the paper.
Funding
This work was supported by the German Research Foundation (DFG) and the Technical University of Munich (TUM) in the framework of the Open Access Publishing Program.
Conflict of Interest
The authors declare that the research was conducted in the absence of any commercial or financial relationships that could be construed as a potential conflict of interest.
Acknowledgments
The authors thank Dr. Richard M. Twyman for manuscript editing.
References
Ahn, B. J., Gaikwad, K. K., and Lee, Y. S. (2016). Characterization and properties of LDPE film with gallic-acid-based oxygen scavenging system useful as a functional packaging material. J. Appl. Polym. Sci. 133:44138. doi: 10.1002/app.44138
Belitz, H.-D., and Grosch, W. (2013). Lehrbuch der Lebensmittelchemie. Berlin; Heidelberg: Springer-Verlag.
Caregnato, P., David Gara, P. M., Bosio, G. N., Gonzalez, M. C., Russo, N., Michelini, M. d. C., et al. (2008). Theoretical and experimental investigation on the oxidation of gallic acid by sulfate radical anions. J. Phys. Chem. A 112, 1188–1194. doi: 10.1021/jp075464z
Cilliers, J. J. L., and Singleton, V. L. (1989). Nonenzymic autoxidative phenolic browning reactions in a caffeic acid model system. J. Agricult. Food Chem. 37, 890–896. doi: 10.1021/jf00088a013
Eslami, A. C., Pasanphan, W., Wagner, B. A., and Buettner, G. R. (2010). Free radicals produced by the oxidation of gallic acid: an electron paramagnetic resonance study. Chem. Cent. J. 4, 1–4. doi: 10.1186/1752-153X-4-15
Friedman, M., and Juergens, H. S. (2000). Effect of ph on the stability of plant phenolic compounds. J. Agricult. Food Chem. 48, 2101–2110. doi: 10.1021/jf990489j
Hotta, H., Sakamoto, H., Nagano, S., Osakai, T., and Tsujino, Y. (2001). Unusually large numbers of electrons for the oxidation of polyphenolic antioxidants. Biochim. Biophys. Acta 1526, 159–167. doi: 10.1016/S0304-4165(01)00123-4
Jabbari, M. (2015). Solvent dependence of protonation equilibria for gallic acid in water and different acetonitrile–water cosolvent systems. J. Mol. Liquids 208(Suppl. C):5–10. doi: 10.1016/j.molliq.2015.03.055
Langowski, H.-C., and Wanner, T. (2005). Organic oxygen scavenger/indicator. EP1952140B1 (WO 2007/059901 A1).
Milenkovic, D., Djorovic, J., Petrovic, V., Avdovic, E., and Markovic, Z. (2018). Hydrogen atom transfer versus proton coupled electron transfer mechanism of gallic acid with different peroxy radicals. React. Kinet. Mech. Catal. 123, 215–230. doi: 10.1007/s11144-017-1286-8
Nikolic, G., Veselinovic, A., Mitic, Z., and Zivanovic, S. (2011). HPLC-DAD study of gallic acid autoxidation in alkaline aqueous solutions and the influence of Mg (II) ion. Acta Facult. Med. Naissensis 28, 219–224. Available online at: https://publisher.medfak.ni.ac.rs/AFMN_1/2011/4-2011/4.pdf
Oniki, T., and Takahama, U. (2004). Free radicals produced by the oxidation of gallic acid and catechin derivatives. J. Wood Sci. 50, 545–547. doi: 10.1007/s10086-003-0591-1
Pant, A. F., Dorn, J., and Reinelt, M. (2018). Effect of temperature and relative humidity on the reaction kinetics of an oxygen scavenger based on gallic acid. Front. Chem. 6:587. doi: 10.3389/fchem.2018.00587
Pant, A. F., and Reinelt, M. (2018). “Kinetic modelling of scavenger reactions: parameter estimation for a gallic acid-based oxygen scavenger,” in FOODSIM'2018, Vol. 25 (Ghent), 89–94.
Pant, A. F., Saengerlaub, S., and Mueller, K. (2017). Gallic acid as an oxygen scavenger in bio-based multilayer packaging films. Materials 10:489. doi: 10.3390/ma10050489
Severino, J. F., Goodman, B. A., Kay, C. W., Stolze, K., Tunega, D., Reichenauer, T. G., et al. (2009). Free radicals generated during oxidation of green tea polyphenols: electron paramagnetic resonance spectroscopy combined with density functional theory calculations. Free Radical Biol. Med. 46, 1076–1088. doi: 10.1016/j.freeradbiomed.2009.01.004
Shahidi, F., and Naczk, M. (2003). Phenolics in Food and Nutraceuticals, 2nd Edn. Boca Raton, FL: CRC Press.
Tóth, I. Y., Szekeres, M., Turcu, R., Sáringer, S., Illés, E., Nesztor, D., et al. (2014). Mechanism of in situ surface polymerization of gallic acid in an environmental-inspired preparation of carboxylated core-shell magnetite nanoparticles. Langmuir 30, 15451–15461. doi: 10.1021/la5038102
Tulyathan, V., Boulton, R. B., and Singleton, V. L. (1989). Oxygen uptake by gallic acid as a model for similar reactions in wines. J. Agricult. Food Chem. 37, 844–849. doi: 10.1021/jf00088a002
Walling, C. (1975). Fenton's reagent revisited. Accounts Chem. Res. 8, 125–131. doi: 10.1021/ar50088a003
Wanner, G. T. (2010). O2-zehrende und -anzeigende packstoffe für lebensmittelverpackungen (thesis). Technical University of Munich, Freising, Germany.
Keywords: active packaging, food packaging, oxygen absorber, polyphenol, 3,4,5-trihydroxybenzoic acid
Citation: Pant AF, Özkasikci D, Fürtauer S and Reinelt M (2019) The Effect of Deprotonation on the Reaction Kinetics of an Oxygen Scavenger Based on Gallic Acid. Front. Chem. 7:680. doi: 10.3389/fchem.2019.00680
Received: 05 May 2019; Accepted: 30 September 2019;
Published: 07 November 2019.
Edited by:
Florent Allais, AgroParisTech Institut des Sciences et Industries du Vivant et de L'environnement, FranceReviewed by:
Paul-Henri Ducrot, INRA UMR1318 Institut Jean Pierre Bourgin, FranceStephane Grelier, Université de Bordeaux, France
Copyright © 2019 Pant, Özkasikci, Fürtauer and Reinelt. This is an open-access article distributed under the terms of the Creative Commons Attribution License (CC BY). The use, distribution or reproduction in other forums is permitted, provided the original author(s) and the copyright owner(s) are credited and that the original publication in this journal is cited, in accordance with accepted academic practice. No use, distribution or reproduction is permitted which does not comply with these terms.
*Correspondence: Matthias Reinelt, bWF0dGhpYXMucmVpbmVsdCYjeDAwMDQwO2l2di5mcmF1bmhvZmVyLmRl