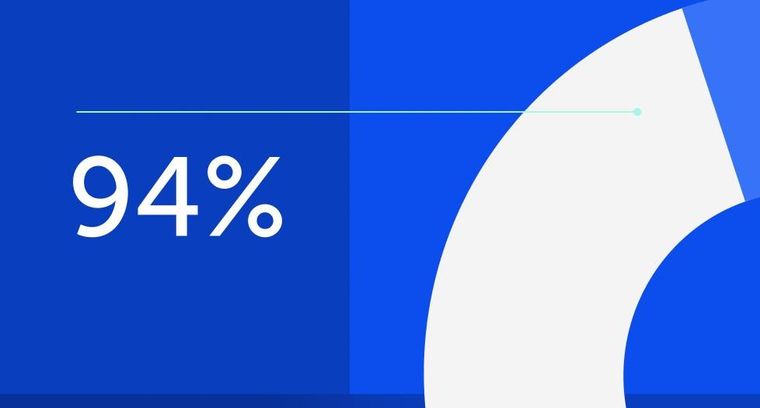
94% of researchers rate our articles as excellent or good
Learn more about the work of our research integrity team to safeguard the quality of each article we publish.
Find out more
MINI REVIEW article
Front. Chem., 30 July 2019
Sec. Polymer Chemistry
Volume 7 - 2019 | https://doi.org/10.3389/fchem.2019.00541
This article is part of the Research TopicCatechol and Polyphenol Chemistry for Smart PolymersView all 7 articles
Catechols are abundant in nature and are believed to perform diverse biological functions that include photoprotection (e.g., melanins), molecular signaling (e.g., catecholamine neurotransmitters), and mechanical adhesion (e.g., mussel glue). Currently, the structure-property-function relationships for catechols remain poorly resolved, and this is especially true for redox-based properties (e.g., antioxidant, pro-oxidant, and radical scavenging activities). Importantly, there are few characterization methods available to probe the redox properties of materials. In this review, we focus on recent studies with redox-active catechol-chitosan films. First, we describe film fabrication methods to oxidatively-graft catechols to chitosan through chemical, enzymatic, or electrochemical methods. Second, we discuss a new experimental characterization method to probe the redox properties of catechol-functionalized materials. This mediated electrochemical probing (MEP) method probes the redox-activities of catechol-chitosan films by: (i) employing diffusible mediators to shuttle electrons between the electrode and grafted catechols; (ii) imposing tailored sequences of input voltages to “tune” redox probing; and (iii) analyzing the output current response characteristics to infer properties. Finally, we demonstrate that the redox properties of catechol-chitosan films enable them to perform antioxidant radical scavenging functions, as well as a pro-oxidant (reactive oxygen-generation) antimicrobial functions. In summary, our increasing knowledge of catechol-chitosan films is enabling us to better-understand the functions of catechols in biology as well as enhancing our capabilities to create advanced functional materials.
In nature, catechols are believed to confer diverse functions to materials: the photo and free radical protection of melanins (Seagle et al., 2005; Dadachova and Casadevall, 2008; Panzella et al., 2013); the hardening of the insect cuticle through crosslinking (Andersen, 2010; Sugumaran and Barek, 2016; Whitten and Coates, 2017); the adhesion of the mussel glue to surfaces (Ryu et al., 2015; Waite, 2017); the virulence of fungal pathogens (Jacobson, 2000; Nosanchuk and Casadevall, 2006); and the innate immunity of insects (Christensen et al., 2005; Nakhleh et al., 2017). In many cases, the synthesis of catecholic materials is initiated by oxidative reactions that yield a reactive intermediate (e.g., the tyrosinase-generated of o-quinones) that can undergo subsequent non-enzymatic reactions. Often the final product has catecholic moieties that could retain redox activity. An overarching hypothesis of our work is that catecholic materials play important roles in redox biology but these roles are under-appreciated, in part, because of the absence of simple characterization methods. Here, we summarize recent research on the biomimetic oxidative-grafting of catechols onto films of the aminopolysaccharide chitosan. To characterize the redox properties of these catechol-chitosan films, we are developing an electrochemical reverse engineering method which is briefly described. Finally, we discuss two applications that highlight the unique technological capabilities for such redox-active catechol-chitosan films.
Chitosan is a pH-responsive film-forming aminopolysaccharide that has been considered for a variety of diverse applications (Shahidi et al., 1999; Krajewska, 2004; Yi et al., 2005; Peppas et al., 2006; Boateng et al., 2008; Crini and Badot, 2008; Kim et al., 2008; Jayakumar et al., 2011; Shin et al., 2017). As illustrated in Figure 1A, we typically electrodeposit our chitosan films through a cathodic neutralization mechanism in which electrochemical reactions are used to controllably generate a region of high pH that neutralizes the chitosan polymer chains and induces their self-assembly into a thin hydrogel film (Wu et al., 2002; Redepenning et al., 2003). The electrodeposited chitosan film is stable in the absence of an applied voltage provided the pH is retained above about 6.5 (chitosan can re-dissolve at lower pH). In a second step, catechol can be oxidatively-grafted to chitosan using various chemical, electrochemical (Wu et al., 2005, 2006), or enzymatic (Sun et al., 1992; Muzzarelli et al., 1994) approaches. In all these fabrication approaches the catechol is oxidized to a reactive o-quinone intermediate that grafts to the chitosan presumably through Schiff-base or Michael-type adduct formation. In summary, chitosan was selected as our film because it: offers appropriate material properties for a range of applications (e.g., biomedical applications); possesses stimuli-responsive film-forming properties that enable electrodeposition; and has nucleophilic primary amines that facilitate catechol's oxidative grafting.
Figure 1. Catechol-chitosan hydrogel films. (A) Fabrication by first electrodepositing a film of the aminopolysaccharide chitosan and then oxidatively-grafting catechol moieties to the film. (B) Electrochemical reverse engineering approach to characterize the redox properties of the catechol-chitosan films.
The conventional approach for characterizing materials is to determine chemical structure and evaluate properties to enable the development of the structure-property relations needed to understand the function of a material in nature or to design a material for a technological application. It has been difficult to apply this conventional approach to catechol-based materials (e.g., melanins and polydopamines) (Lee et al., 2007; D'Ischia et al., 2014; Delparastan et al., 2019) for a couple reasons. First, catechol-based materials are often insoluble in water and most solvents, and may have complex non-homogeneous chemical structures that are difficult to resolve using standard chemical characterization methods. For instance, the oxidatively grafted catechols in Figure 1A may be connected to chitosan through various linkages (e.g., Schiff-bases or Michael-type adducts), may engage in the crosslinking of chitosan chains, and/or may involve the grafting of oligomeric-phenolic species to chitosan. Thus, the catechol-chitosan films likely possess complex structures consistent with complex structures of natural catecholic materials (e.g., melanins).
A second reason why it has been difficult to generate structure-property relations for catecholic materials is that important functions (e.g., antioxidant protection and radical scavenging) often rely on redox-properties and the methods for characterizing redox properties of insoluble materials are not particularly versatile. For our example, initial studies showed that our catechol-chitosan films are non-conducting and could not directly exchange electrons with the underlying electrode (Kim et al., 2010). Presumably, these films are non-conducting because the oxidative grafting of catechol does not generate the conjugated aromaticity that is common to conducting polymers. Further, presumably few of the grafted catechol moieties in these hydrogel films (≈1 μm thick) are contacting the underlying electrode which is needed for direct electron exchange. Although these catechol-chitosan films are non-conducting, they have been shown to be redox-active as the grafted catechol moieties can accept electrons from diffusible reductants and donate electrons to diffusible oxidants. Typical approaches to characterize redox-active but non-conducting materials involve incubation of the material in the presence of a diffusible reductant (or oxidant) and measuring how much of this diffusible species is consumed by donating electrons to (or accepting electrons from) the material. Such methods have significant limitations: the methods can be time-consuming; redox activities are typically observed in one direction (oxidation or reduction); and the methods cannot easily determine if the redox reactions are reversible or irreversible (conventional approaches would require multiple solution exchanges to provide the oxidative and reductive conditions to test for the reversibility of redox reactions).
To overcome these limitations, we developed an electrochemical reverse engineering approach that does not require knowledge of chemical structure and focuses on redox properties (Kim et al., 2013b, 2014b; Kang et al., 2018). Specifically, Figure 1B shows that this mediated electrochemical probing (MEP) method: (i) uses electrochemical instrumentation to expose our catechol-chitosan films to controlled voltage inputs; (ii) uses diffusible redox mediators to “transmit” these voltage inputs from the electrode surface into the film; and (iii) generates an output response-current that can be analyzed to assess the film's redox properties.
Experimentally, an electrode coated with the catechol-chitosan film is immersed in a solution containing diffusible mediators that serve to shuttle electrons between the electrode and the grafted catechols. The mediators are molecules that can readily and reversibly donate and accept electrons. Such mediators can be selected from those commonly used in electrochemistry, or can be redox-active molecules derived from biology. Importantly, multiple mediators can be added to the same solution, but each mediator is only “activated” to donate/accept electrons when the voltage of the underlying electrode is poised near this mediator's redox potential (E0). [Note: this is analogous to a solution containing numerous acid and base species, and an individual species only donates/accepts a proton when the pH is near its pKa].
We initiate redox probing by imposing a tailored sequence of input voltages that serve to “tune” the voltage window being probed. In the example illustrated in Figure 1B, we probed the catechol-chitosan film using two mediators and a cyclic imposed electrode voltage. When the imposed voltage is cycled into the reducing region, the Ru(NH3)6Cl3 mediator (Ru3+) is reduced at the electrode to its Ru2+ state which diffuses into the film and can donate electrons to the film presumably converting the oxidized quinone moieties into reduced catechol moieties. As illustrated in Figure 1B, this Ru3+-mediated transfer of electrons from the electrode to the film involves a reductive redox-cycling mechanism. When the imposed voltage is cycled into the oxidative range, the ferrocene dimethanol mediator (Fc) can engage in oxidative redox-cycling that serves to transfer electrons from the film to the electrode presumably converting the reduced catechol moieties into oxidized quinone moieties. [Note: the Ru3+ and Fc mediators engage in one-electron transfer steps while the interconversion between quinone and catechol involves two electrons and may proceed through a semiquinone intermediate].
The output response-current in Figure 1B shows the response-characteristics of the catechol-chitosan film in comparison to those for a control (redox-inactive) chitosan film. One obvious feature of this response is the significant amplification of the Ru3+-reduction and Fc-oxidation currents. Amplification of mediator currents is a signature indicating that the catechol-chitosan films are redox-active and can accept electrons (through Ru3+-mediated reductive redox-cycling) and donate electrons (through Fc+-mediated oxidative redox-cycling). A more subtle point is that these results suggest that the E0 values for the Ru3+ (−0.2 V vs. Ag/AgCl) and Fc+ (+0.25 V) mediators bracket the E0 value for catechol-chitosan film. This E0 range (−0.2 to +0.25 V vs. Ag/AgCl) is consistent with the E0 value for reported for catechol (+0.2 V vs. Ag/AgCl) (Behera et al., 2008). A second feature of the response-characteristic is obvious from the input-output curves which show a steady output pattern over multiple cycles. This steady output-response indicates that the catechol-chitosan film can be repeatedly oxidized and reduced.
In summary, the MEP enabled us to determine that the catechol-chitosan film is redox-active and can be repeatedly oxidized and reduced. This ability to accept, store and donate electrons essentially means the catechol-chitosan film is a redox-capacitor (Kim et al., 2012, 2013a, 2014a, 2015; Liu et al., 2014). Importantly, the redox potential of the catechol-chitosan film is in the mid-physiological range and other studies have shown that this film has a broad “substrate range” in that it can accept electrons from various biological reductants (e.g., NADPH and ascorbate) and donate electrons to biological oxidants (e.g., O2) (Kim et al., 2012, 2014a). In the following, we cite two applications in which the catechol-chitosan redox-capacitor film can engage in biologically-relevant redox-based “communication.”
In nature, melanins are believed to perform protective functions that are due, in some cases, to their ability to scavenge free radicals (e.g., radiation-induced free radicals). One of the most extreme examples of such protection is suggested by the ability of melanized fungi to survive in the highly radioactive Chernobyl site (Dadachova and Casadevall, 2008; Dighton et al., 2008; Gessler et al., 2014). We used our MEP system to test the hypothesis that the catechol-chitosan film could donate electrons to scavenge (i.e., quench) oxidative free radicals.
To test this radical-scavenging hypothesis, we prepared catechol-chitosan films with different extents of catechol modification (Cao et al., 2018). We used electrochemical measurements to provide a semi-quantitative estimate of film's modification ratio (MR); ratio of grafted catechol moieties per chitosan amine (MRs = 0.23, 0.32, 0.42, and 0.52). Experimentally, we tested for radical-scavenging activity using the standard reagent 2,2′-azino-bis(3-ethylbenzothiazoline-6-sulfonic acid) diammonium salt (ABTS) that can be electrochemically oxidized to generate the ABTS+• radical. As illustrated in Figure 2A we used cyclic voltage inputs to oxidize ABTS for radical generation and used two output measurements to provide evidence that the catechol-chitosan films can quench the ABTS+• radicals by donating electrons through a redox-cycling mechanism. One output measurement was current, and we observed that ABTS oxidation currents were amplified for catechol-modified films compared to unmodified chitosan films, and the extent of amplification increased with the extent of catechol-modification.
Figure 2. Anti- and pro- oxidant functions of catechol-chitosan films. (A) Antioxidant radical scavenging activities: ABTS oxidative redox-cycling associated with radical scavenging is apparent from the amplified electrochemical (electrical) currents and attenuated optical absorbance (results from Cao et al., 2018). (B) Bio-inspired pro-oxidant activities associated with reactive oxygen species (ROS) generation confer antimicrobial activities to wound dressings (results from Liu et al., 2018).
The second output measurement was absorbance at 420 nm: the ABTS+• radical solution is green-colored while the ABTS solution is colorless. As illustrated in Figure 2A, when the imposed voltage is cycled into the oxidative region, the absorbance increases consistent with the generation of the ABTS+• radical. For the case, of the unmodified chitosan film, the absorbance is observed to reach a peak and then decrease when the voltage was cycled into the reducing region which is consistent with the electrochemical reduction of the ABTS+• radical. In comparison to the unmodified chitosan film, Figure 2A shows that the catechol-chitosan films attenuated the optical absorbance with the extent of attenuation increasing with the extent of catechol modification.
In summary, these results demonstrate that the catechol-chitosan film can accept electrons to quench the oxidative ABTS+• radical (Cao et al., 2018). We observed this radical scavenging activity is context dependent: the films must be in a reduced state to have available electrons to donate to the ABTS+• radical (this is why Ru3+ is included in the electrochemical experiment illustrated in Figure 2A). Importantly, the films can be poised in their reduced state using non-electrochemical reductants such as the inexpensive biological reductant, ascorbic acid (i.e., vitamin C). In additional studies, we observed that the catechol-chitosan film can not only donate electrons to scavenge an oxidative free radical (i.e., ABTS+•) but it can also accept electrons to quench a reductive free radical (e.g., paraquat) (Cao et al., 2018). We envision such catechol-chitosan films could be used as protective coating for diverse applications (e.g., clothing).
While the above example illustrates that the electron donating activities of the catechol-chitosan film enable it to perform protective antioxidant functions, these same redox-activities enable the film to perform pro-oxidant functions. Specifically, Figure 2B illustrates that electron-donation to O2 could generate damaging reactive oxygen species (ROS). In fact, is was suggested that the melanin that is synthesized by insects to encapsulate invading pathogenic bacteria (Christensen et al., 2005; Falabella et al., 2012; Nakhleh et al., 2017) may be redox-active and capable of transferring electrons from endogenous reducing equivalents to O2 for the sustained and localized generation of antimicrobial ROS (Nappi and Christensen, 2005). Inspired by this example, we prepared free-standing catechol-chitosan films, and converted them to either an oxidized state (by a 2-h exposure to water while pure O2 was being bubbled through the water) or to a reduced state (by a 15-min incubation in a 300 mM ascorbic acid solution), and then evaluated their antimicrobial activities (Liu et al., 2018). Specifically, we performed antimicrobial studies using methicillin resistant Staphylococcus aureus (MRSA). The photograph of the Petri dish in Figure 2B shows a large zone of inhibition for the catechol-chitosan film that was poised in a reduced state, while little or no inhibition is observed for the catechol-chitosan film that was poised in an oxidized state or for the unmodified chitosan film. [Chemical evidence for H2O2-generation by the reduced film is provided in the original paper along with evidence that intermittent ascorbate treatments could be used to regenerate the ROS-generating abilities of the catechol-chitosan film (Liu et al., 2018)].
One application for a film that offers localized and prolonged ROS-generating activities is as an antimicrobial wound dressing. Initial studies to test for in vivo antimicrobial activities involved an infected wound model using Sprague Dawley (SD) rats. Specifically, an incision was pre-seeded with MRSA, treated with a film (i.e., model dressing) and after 3 days inspected. The photographs in Figure 2B show the wound treated with a catechol-chitosan film that had been poised in its reduced state (by ascorbic acid treatment) showed no obvious festering compared to the control catechol-chitosan film that had been poised in an oxidized state. After taking these photographs, the films were recovered and analyzed, and we observed a 2 order-of-magnitude lower bacterial concentration for the reduced catechol-chitosan film compared to the control films (Liu et al., 2018).
In summary, the oxidative grafting of catechols to chitosan confers redox activities that can be used to perform either protective antioxidant radical-scavenging functions, or pro-oxidant antimicrobial functions. Importantly, these results indicate that catecholics are active materials and display context-dependent behaviors (oxidant vs. reductant) that could have important implications in biology and technology.
EK developed fabrication and electrochemical reverse engineering methodologies. MK developed spectroelectrochemical reverse engineering methodology. HL performed experiments demonstrating antimicrobial properties and wound healing capabilities. CC performed experiments demonstrating radical scavenging activities. CL provided insights on requirements for biomedical materials for wound healing. WB provided insights on the use of mediated electrochemical probing in biological systems. XQ coordinated research on the antimicrobial wound dressing. GP coordinated research on the radical scavenging films and prepared the manuscript.
This work was supported by the United States National Science Foundation (DMREF-1435957) and China's 111 Project (B14018).
The authors declare that the research was conducted in the absence of any commercial or financial relationships that could be construed as a potential conflict of interest.
Andersen, S. O. (2010). Insect cuticular sclerotization: a review. Insect Biochem. Mol. Biol. 40, 166–178. doi: 10.1016/j.ibmb.2009.10.007
Behera, S., Sampath, S., and Raj, C. R. (2008). Electrochemical functionalization of a gold electrode with redox-active self-assembled monolayer for electroanalytical application. J. Phys. Chem. C 112, 3734–3740. doi: 10.1021/jp076007l
Boateng, J. S., Matthews, K. H., Stevens, H. N. E., and Eccleston, G. M. (2008). Wound healing dressings and drug delivery systems: a review. J. Pharm. Sci. 97, 2892–2923. doi: 10.1002/jps.21210
Cao, C., Kim, E., Liu, Y., Kang, M., Li, J., Yin, J. J., et al. (2018). Radical scavenging activities of biomimetic catechol-chitosan films. Biomacromolecules 19, 3502–3514. doi: 10.1021/acs.biomac.8b00809
Christensen, B. M., Li, J., Chen, C.-C., and Nappi, A. J. (2005). Melanization immune responses in mosquito vectors. Trends Parasitol. 21, 192–199. doi: 10.1016/j.pt.2005.02.007
Crini, G., and Badot, P.-M. (2008). Application of chitosan, a natural aminopolysaccharide, for dye removal from aqueous solutions by adsorption processes using batch studies: a review of recent literature. Prog. Polym. Sci. 33, 399–447. doi: 10.1016/j.progpolymsci.2007.11.001
Dadachova, E., and Casadevall, A. (2008). Ionizing radiation: how fungi cope, adapt, and exploit with the help of melanin. Curr. Opin. Microbiol. 11, 525–531. doi: 10.1016/j.mib.2008.09.013
Delparastan, P., Malollari, K. G., Lee, H., and Messersmith, P. B. (2019). Direct evidence for the polymeric nature of polydopamine. Angew. Chemie Int. Ed. 58, 1077–1082. doi: 10.1002/anie.201811763
Dighton, J., Tugay, T., and Zhdanova, N. (2008). Fungi and ionizing radiation from radionuclides. FEMS Microbiol. Lett. 281, 109–120. doi: 10.1111/j.1574-6968.2008.01076.x
D'Ischia, M., Napolitano, A., Ball, V., Chen, C. T., and Buehler, M. J. (2014). Polydopamine and eumelanin: from structure-property relationships to a unified tailoring strategy. Acc. Chem. Res. 47, 3541–3550. doi: 10.1021/ar500273y
Falabella, P., Riviello, L., Pascale, M., Lelio, I. D., Tettamanti, G., Grimaldi, A., et al. (2012). Functional amyloids in insect immune response. Insect Biochem. Mol. Biol. 42, 203–211. doi: 10.1016/j.ibmb.2011.11.011
Gessler, N. N., Egorova, A. S., and Belozerskaya, T. A. (2014). Melanin pigments of fungi under extreme environmental conditions (Review). Appl. Biochem. Microbiol. 50, 105–113. doi: 10.1134/S0003683814020094
Jacobson, E. S. (2000). Pathogenic roles for fungal melanins. Clin. Microbiol. Rev. 13, 708–717. doi: 10.1128/CMR.13.4.708
Jayakumar, R., Prabaharan, M., Sudheesh Kumar, P. T., Nair, S. V., and Tamura, H. (2011). Biomaterials based on chitin and chitosan in wound dressing applications. Biotechnol. Adv. 29, 322–337. doi: 10.1016/j.biotechadv.2011.01.005
Kang, M., Kim, E., Temoçin, Z., Li, J., Dadachova, E., Wang, Z., et al. (2018). Reverse engineering to characterize redox properties: revealing melanin's redox activity through mediated electrochemical probing. Chem. Mater. 30, 5814–5826. doi: 10.1021/acs.chemmater.8b02428
Kim, E., Gordonov, T., Bentley, W. E., and Payne, G. F. (2013a). Amplified and in situ detection of redox-active metabolite using a biobased redox capacitor. Anal. Chem. 85, 2102–2108. doi: 10.1021/ac302703y
Kim, E., Gordonov, T., Liu, Y., Bentley, W. E., and Payne, G. F. (2013b). Reverse engineering to suggest biologically relevant redox activities of phenolic materials. ACS Chem. Biol. 8, 716–724. doi: 10.1021/cb300605s
Kim, E., Leverage, W. T., Liu, Y., White, I. M., Bentley, W. E., and Payne, G. F. (2014a). Redox-capacitor to connect electrochemistry to redox-biology. Analyst 139, 32–43. doi: 10.1039/C3AN01632C
Kim, E., Liu, Y., Bentley, W. E., and Payne, G. F. (2012). Redox capacitor to establish bio-device redox-connectivity. Adv. Funct. Mater. 22, 1409–1416. doi: 10.1002/adfm.201101946
Kim, E., Liu, Y., Leverage, W. T., Yin, J. J., White, I. M., Bentley, W. E., et al. (2014b). Context-dependent redox properties of natural phenolic materials. Biomacromolecules 15, 1653–1662. doi: 10.1021/bm500026x
Kim, E., Liu, Y., Shi, S.-W., and Yang, X. (2010). Biomimetic approach to confer redox activity to thin chitosan films. Adv. Funct. Mater. 20, 2683–2694. doi: 10.1002/adfm.200902428
Kim, E., Xiong, Y., Cheng, Y., Wu, H.-C., Liu, Y., Morrow, B. H., et al. (2015). Chitosan to connect biology to electronics: fabricating the bio-device interface and communicating across this interface. Polymers 7, 1–46. doi: 10.3390/polym7010001
Kim, I.-Y., Seo, S. J., Moon, H. S., Yoo, M. K., Park, I. Y., Kim, B. C., et al. (2008). Chitosan and its derivatives for tissue engineering applications. Biotechnol. Adv. 26, 1–21. doi: 10.1016/j.biotechadv.2007.07.009
Krajewska, B. (2004). Application of chitin- and chitosan-based materials for enzyme immobilizations: a review. Enzyme Microb. Technol. 35, 126–139. doi: 10.1016/j.enzmictec.2003.12.013
Lee, H., Dellatore, S. M., Miller, W. M., and Messersmith, P. B. (2007). Mussel-inspired surface chemistry for multifunctional coatings. Science 318, 426–430. doi: 10.1126/science.1147241
Liu, H., Qu, X., Kim, E., Lei, M., Dai, K., Tan, X., et al. (2018). Bio-inspired redox-cycling antimicrobial film for sustained generation of reactive oxygen species. Biomaterials 162, 109–122. doi: 10.1016/j.biomaterials.2017.12.027
Liu, Y., Kim, E., White, I. M., Bentley, W. E., and Payne, G. F. (2014). Information processing through a bio-based redox capacitor: signatures for redox-cycling. Bioelectrochemistry 98, 94–102. doi: 10.1016/j.bioelechem.2014.03.012
Muzzarelli, R. A. A., Ilari, P., Xia, W., Pinotti, M., and Tomasetti, M. (1994). Tyrosinase-mediated quinone tanning of chitinous materials. Carbohydr. Polym. 24, 295–300. doi: 10.1016/0144-8617(94)90074-4
Nakhleh, J., El Moussawi, L., and Osta, M. A. (2017). The melanization response in insect immunity. Adv. Insect Physiol. 52, 83–109. doi: 10.1016/bs.aiip.2016.11.002
Nappi, A. J., and Christensen, B. M. (2005). Melanogenesis and associated cytotoxic reactions: applications to insect innate immunity. Insect Biochem. Mol. Biol. 35, 443–459. doi: 10.1016/j.ibmb.2005.01.014
Nosanchuk, J. D., and Casadevall, A. (2006). Impact of melanin on microbial virulence and clinical resistance to antimicrobial compounds. Antimicrob. Agents Chemother. 50, 3519–3528. doi: 10.1128/AAC.00545-06
Panzella, L., Gentile, G., D'Errico, G., Della Vecchia, N. F., Errico, M. E., Napolitano, A., et al. (2013). Atypical structural and π-electron features of a melanin polymer that lead to superior free-radical-scavenging properties. Angew. Chemie Int. Ed. 52, 12684–12687. doi: 10.1002/anie.201305747
Peppas, N. A., Hilt, J. Z., Khademhosseini, A., and Langer, R. (2006). Hydrogels in biology and medicine: from molecular principles to bionanotechnology. Adv. Mater. 18, 1345–1360. doi: 10.1002/adma.200501612
Redepenning, J., Venkataraman, G., Chen, J., and Stafford, N. (2003). Electrochemical preparation of chitosan/hydroxyapatite composite coatings on titanium substrates. J. Biomed. Mater. Res. 66A, 411–416. doi: 10.1002/jbm.a.10571
Ryu, J. H., Hong, S., and Lee, H. (2015). Bio-inspired adhesive catechol-conjugated chitosan for biomedical applications: a mini review. Acta Biomater. 27, 101–115. doi: 10.1016/j.actbio.2015.08.043
Seagle, B.-L. L., Rezai, K. A., Gasyna, E. M., Kobori, Y., Rezaei, K. A., and Norris, J. R. Jr. (2005). Time-resolved detection of melanin free radicals quenching reactive oxygen species. J. Am. Chem. Soc. 127, 11220–11221. doi: 10.1021/ja052773z
Shahidi, F., Arachchi, J. K. V., and Jeon, Y.-J. (1999). Food applications of chitin and chitosans. Trends Food Sci. Technol. 10, 37–51. doi: 10.1016/S0924-2244(99)00017-5
Shin, M., Park, S. G., Oh, B. C., Kim, K., Jo, S., Lee, M. S., et al. (2017). Complete prevention of blood loss with self-sealing haemostatic needles. Nat. Mater. 16, 147–152. doi: 10.1038/nmat4758
Sugumaran, M., and Barek, H. (2016). Critical analysis of the melanogenic pathway in insects and higher animals. Int. J. Mol. Sci. 17:1753. doi: 10.3390/ijms17101753
Sun, W. Q., Payne, G. F., Moas, M. S. G. L., Chu, J. H., and Wallace, K. K. (1992). Tyrosinase reaction/chitosan adsorption for removing phenols from wastewater. Biotechnol. Prog. 8, 179–186. doi: 10.1021/bp00015a002
Waite, J. H. (2017). Mussel adhesion - essential footwork. J. Exp. Biol. 220, 517–530. doi: 10.1242/jeb.134056
Whitten, M. M. A., and Coates, C. J. (2017). Re-evaluation of insect melanogenesis research: views from the dark side. Pigment Cell Melanoma Res. 30, 386–401. doi: 10.1111/pcmr.12590
Wu, L.-Q., Gadre, A. P., Yi, H., Kastantin, M. J., Rubloff, G. W., Bentley, W. E., et al. (2002). Voltage-dependent assembly of the polysaccharide chitosan onto an electrode surface. Langmuir 18, 8620–8625. doi: 10.1021/la020381p
Wu, L.-Q., Ghodssi, R., Elabd, Y. A., and Payne, G. F. (2005). Biomimetic pattern transfer. Adv. Funct. Mater. 15, 189–195. doi: 10.1002/adfm.200400279
Wu, L.-Q., McDermott, M. K., Zhu, C., Ghodssi, R., and Payne, G. F. (2006). Mimicking biological phenol reaction cascades to confer mechanical function. Adv. Funct. Mater. 16, 1967–1974. doi: 10.1002/adfm.200500792
Keywords: chitosan, catechol, redox, radical scavenger activity, antimicrobial dressing
Citation: Kim E, Kang M, Liu H, Cao C, Liu C, Bentley WE, Qu X and Payne GF (2019) Pro- and Anti-oxidant Properties of Redox-Active Catechol-Chitosan Films. Front. Chem. 7:541. doi: 10.3389/fchem.2019.00541
Received: 14 March 2019; Accepted: 15 July 2019;
Published: 30 July 2019.
Edited by:
Henrik Birkedal, Aarhus University, DenmarkCopyright © 2019 Kim, Kang, Liu, Cao, Liu, Bentley, Qu and Payne. This is an open-access article distributed under the terms of the Creative Commons Attribution License (CC BY). The use, distribution or reproduction in other forums is permitted, provided the original author(s) and the copyright owner(s) are credited and that the original publication in this journal is cited, in accordance with accepted academic practice. No use, distribution or reproduction is permitted which does not comply with these terms.
*Correspondence: Xue Qu, cXV4dWVAZWN1c3QuZWR1LmNu; Gregory F. Payne, Z3BheW5lQHVtZC5lZHU=
†These authors have contributed equally to this work
Disclaimer: All claims expressed in this article are solely those of the authors and do not necessarily represent those of their affiliated organizations, or those of the publisher, the editors and the reviewers. Any product that may be evaluated in this article or claim that may be made by its manufacturer is not guaranteed or endorsed by the publisher.
Research integrity at Frontiers
Learn more about the work of our research integrity team to safeguard the quality of each article we publish.