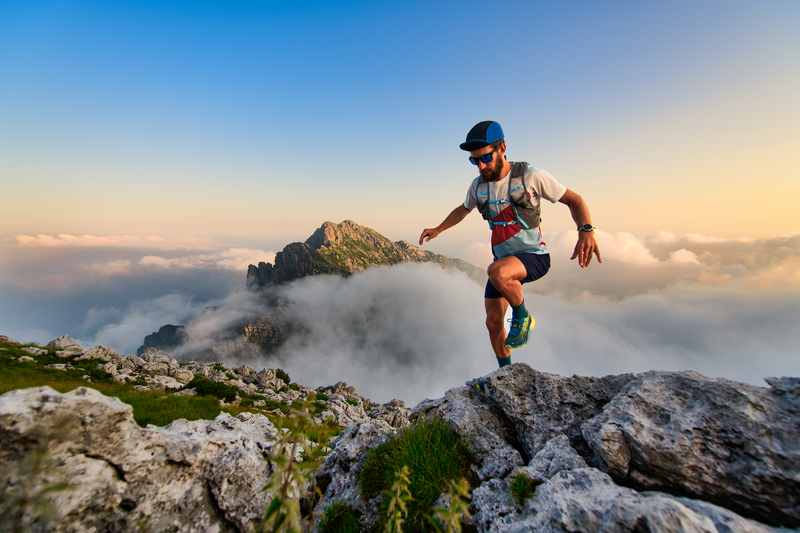
94% of researchers rate our articles as excellent or good
Learn more about the work of our research integrity team to safeguard the quality of each article we publish.
Find out more
REVIEW article
Front. Chem. , 24 July 2019
Sec. Inorganic Chemistry
Volume 7 - 2019 | https://doi.org/10.3389/fchem.2019.00525
This article is part of the Research Topic Molecular Catalysts for CO2 Fixation/Reduction View all 14 articles
The escalating rate of fossil fuel combustion contributes to excessive CO2 emission and the resulting global climate change has drawn considerable attention. Therefore, tremendous efforts have been devoted to mitigate the CO2 accumulation in the atmosphere. Carbon capture and storage (CCS) strategy has been regarded as one of the promising options for controlling CO2 build-up. However, desorption and compression of CO2 need extra energy input. To circumvent this energy issue, carbon capture and utilization (CCU) strategy has been proposed whereby CO2 can be captured and in situ activated simultaneously to participate in the subsequent conversion under mild conditions, offering valuable compounds. As an alternative to CCS, the CCU has attracted much concern. Although various absorbents have been developed for the CCU strategy, the direct, in situ chemical conversion of the captured CO2 into valuable chemicals remains in its infancies compared with the gaseous CO2 conversion. This review summarizes the recent progress on CO2 capture and in situ catalytic transformation. The contents are introduced according to the absorbent types, in which different reaction type is involved and the transformation mechanism of the captured CO2 and the role of the absorbent in the conversion are especially elucidated. We hope this review can shed light on the transformation of the captured CO2 and arouse broad concern on the CCU strategy.
The demand for energy of the rapid industrialization results in large-scale combustion of fossil fuel, which causes excessive emissions of carbon dioxide. As the detrimental environmental impacts of CO2 have drawn considerable attention, various strategies have been developed to mitigate CO2 accumulation in the atmosphere, among which carbon capture and storage/sequestration (CCS) is considered as a promising CO2 reducing option (Alexander et al., 2015). Nowadays, a plethora of CO2 absorbents have been developed to facilitate CO2 capture and desorption. Nevertheless, the extensive energy needed in the absorbent regeneration and CO2 separation is not conducive to the implementation of CCS strategy.
In contrast to carbon sequestration, converting CO2 into valuable chemicals could be a sustainable option, which has been proposed by Ciamician as early as 1912 (Ciamician, 1912). In recent decades, CO2 conversion has attracted considerable concern and been intensively investigated (Rahman et al., 2017). However, in most processes for CO2 conversion, pure or high pressure CO2 is needed, implying that the CO2 from the atmosphere or industrial exhaust cannot be used as C1 source directly and thus the energy issue in CO2 capture and separation still remains.
To address the energy penalties associated with CCS strategy and realize the direct fixation of CO2 from the atmosphere or industrial exhaust, the CO2 capture and utilization (CCU) strategy, whereby the captured CO2 is used as a non-toxic, abundant, and sustainable feedstock to produce valuable organic compounds via chemical, electrochemical or photochemical reactions, was proposed and now is flourishing (Scheme 1). By now, both organic compounds and functional materials containing the bridging-carbonato metal complexes can be obtained from atmospheric CO2 using the CCU strategy (Yang et al., 2011; Liu et al., 2012; Massoud et al., 2015). Although realizing the attractive prospect of the CO2 capture and in situ conversion in the industry scale remains a challenge (Zhang and Lim, 2015), the emergence of efficient absorbents and the development of CO2 transformation will cast light on it. In continuation of our work on the conversion of the captured CO2 into value-added organic chemicals, this review summarized the recent progress on CO2 capture and in situ conversion into organic products.
To realize the carbon capture and in situ conversion strategy, effective absorbents are always necessary. Ideally, the absorbents for CCU strategy should not only capture CO2, but also activate CO2 and even the substrate. Thus, the chemical transformation can proceed under mild conditions. Up to now, organic and inorganic bases, N-heterocyclic carbenes (NHCs) and N-heterocyclic olefins (NHOs), ionic liquids (ILs) and frustrated Lewis pairs (FLPs) have already been applied to CO2 capture and in situ conversion. A plethora of valuable organic chemicals have been obtained through the CCU strategy as shown in Scheme 2.
Due to the electrophilicity of carbon atom in CO2, the organic and inorganic bases containing strong nucleophilic atom have been widely used in CO2 trapping, where the base can interact with CO2 directly or function as a proton acceptor. The resulting CO2 capture products i.e., CO2 adducts have been employed for subsequent synthesis of various valuable chemicals.
Considering the transformations of the captured CO2 derived from primary and secondary amines and amino alcohols to isocyanates, carbamates, ureas, and oxazolidinones have been concerned by several excellent review papers (Hampe and Rudkevich, 2003; Chaturvedi and Ray, 2006; Yang et al., 2012; Tamura et al., 2014; Wang et al., 2017a,b), here we focus on the transcarboxylation effect and other transformations of the captured CO2, namely CO2 derivatives.
In the synthesis of carbamates, the aprotic organic bases can function as CO2 absorbents and transcarboxylation agents. The initial attempt was made by Rossi group, in which CO2 is trapped by a methanol solution of commercially available tetraethylammonium hydroxide. The resulting tetraethylammonium hydrogen carbonate can be used as a surrogate of CO2 in the synthesis of carbamate. Meanwhile, the presence of tetraethylammonium ion as counterion increases the nucleophilicity of carbamate anion (Inesi et al., 1998).
Soon after, Franco group has successfully identified the DBU-CO2 complex via reacting CO2 with DBU (1,8-Diazabicyclo[5.4.0]undec-7-ene) in anhydrous acetonitrile, implying that DBU can be used as CO2 trap reagent (Pérez et al., 2002). Moreover, the resulting reactive DBU-CO2 adduct can be utilized as transcarboxylating reagent for synthesis of N-alkyl carbamates. Later, the same group revealed the activation capacity of CO2 by other bicyclic amidines and observed the inverse relation between the thermal stability and the transcarboxylating activity for the amidine-CO2 adducts (Scheme 3) (Pérez et al., 2004), which is the first time to investigate the activation ability of organic bases to CO2.
The combination of organic base and alcohol is an efficient CO2 capture system and the absorbed CO2 can be in situ transformed. The prototypical example is the polyethylene glycol (PEG)/superbase system developed by our group in 2011 (Yang et al., 2011). In the capture step, the superbase is used as a proton acceptor and almost equimolar CO2 per mole superbase can be absorbed (Scheme 4). The resulting liquid amidinium carbonate can directly react with n-butylamine at 110°C to afford dibutyl urea in almost quantitative yield (96%) without any other additives. This protocol can be used in the synthesis of other symmetrical urea derivatives.
In the above examples, the captured CO2 in the transcarboxylating agents can be regarded as the activated CO2 because the linear structure of CO2 is converted to bent structure, which is more liable to nucleophilic attack.
The “CO2 absorption and subsequent transcarboxylation” triggers the research on CO2 capture and in situ transformation. Several years later, M. Yoshida and coworkers use DBU to enrich and activate CO2 in air and perform the first example of directly transforming atmospheric CO2 into the substituted 5-vinylideneoxazolidin-2-ones using propargylic substrate 4-(benzylamino)-2-butynyl carbonates or benzoates as a substrate (Scheme 4) (Yoshida et al., 2008). In their follow-up work, they further improve the reaction efficiency by utilizing AgNO3 as catalyst and propargylic amines as substrates (Scheme 4) (Yoshida et al., 2012).
Inspired by these works, our group designs a series of novel CO2 capture and activation systems. For example, by employing ammonium iodide as catalyst, the cycloaddition reaction of various aziridines with the captured CO2 by NH2PEG150NH2 gives rise to oxazolidinones at 40°C in >94% yield and selectivity (Scheme 4) (Yang et al., 2011).
Soon after, we report the first example of steric-hindrance-controlled CO2 absorption, where the sodium N-alkylglycinates and N-alkylalaninates dissolved in PEG150 are used to capture CO2, generating the carbamic acid rather than the ammonium carbamate (Liu et al., 2012). N-isopropylglycinate is found to be the best absorbent for the rapid and reversible capture of almost equimolar CO2. Crucially, the captured CO2 can be activated simultaneously and the resulting carbamic acid can react with either aziridine or propargyl amine to afford oxazolidinones in the presence of NH4I and AgOAc as a catalyst, respectively (Scheme 4).
Motivated by these results, we further develop potassium phthalimide as absorbent to realize equimolar CO2 capture in PEG150. Moreover, the obtained product can be used as in situ transcarboxylating reagent to synthesize oxazolidinone derivatives (Scheme 4) (Zhang et al., 2014).
Recently, Hu group subtly designs a CCU example (Yu et al., 2016), in which carbamate salts generated from CO2 and primary amines are used as substrates. The captured CO2 not only acts as a reactant but also acts as a protecting reagent for the amine to avoid poisoning of the copper catalyst. By using 5 mol% of CuI as catalyst, carbamate salts can react with aromatic aldehydes and aromatic terminal alkynes, affording the important oxazolidin-2-ones (Scheme 4).
Based on these inspiring results, our group uses ammonium carbamates as surrogates of carbon dioxide and secondary amines in the three-component synthesis of β-oxopropylcarbamates from propargylic alcohols, secondary amines, and CO2. Catalyzed by silver (I) catalyst, ammonium carbamates can react with propargylic alcohols to generate β-oxopropylcarbamates under atmospheric pressure (Scheme 5) (Song et al., 2015). In this example, the substitution of pure CO2 with the captured CO2 can facilitate the reaction running at atmospheric pressures with a broad substrate and reaction application scope. Furthermore, the solid ammonium carbamates are easier to handle and quantify than the volatile amines and gaseous CO2.
The transcarboxylation is an important transformation strategy for the CO2 adducts to valuable chemicals. However, for the CO2 adducts formed by base and CO2, the transcarboxylation is still limited to the substrates including amines, propargylamines and aziridines. Therefore, novel CO2 absorbents and extended substrates are expected to facilitate the application of CO2 adducts as transcarboxylation reagent in CCU strategy. Besides transcarboxylation, the integral transformation of CO2 capture products is another attractive option in CCU strategy, wherein the ammonium carbamates derived from CO2 and amines is a promising raw material. Nevertheless, the integral transformation of ammonium carbamates to valuable chemicals remains sporadic and underexplored. Hopefully, more conversion protocols of ammonium carbamates can be designed based on the reactivity of amine and CO2.
CO2 hydrogenation is widely investigated in the CCU strategy because the basic absorbent can react with the resulting formic acid to form formate, thus overcomes the thermodynamic limitation in the hydrogenation of CO2. In the researches on CO2 capture and in situ hydrogenation, both metal-based homogeneous catalysts (containing Rh-, Ru-, and Fe-based catalysts) and heterogeneous catalysts have been investigated.
The first example of CO2 capture and in situ hydrogenation is reported by our group in 2013, in which polyethyleneimine 600 (PEI600) or the combination of PEI600 and ethylene glycol is developed to absorb gaseous CO2, affording the PEI-CO2 or ethylene glycol-CO2 adducts. With RhCl3·3H2O/CyPPh2 as catalyst, the captured CO2 can be in situ transformed to formate (Scheme 6) (Li et al., 2013). Furthermore, direct hydrogenation of ammonium carbamate derived from CO2, e.g., DETA+CO2 − (DETA = diethylenetriamine), ammonium carbonates such as [DBNH] [OCO2(C2H4O)3H], [DBNH] [OCO2CH2OH] (DBN = 1,5-diaza bicycle[4.3.0]non-5-ene), is also successfully performed facilitated by this Rh-based catalyst. Notably, a higher reaction rate and better results can be achieved when using the captured CO2 in the form of ammonium carbonates as feedstock than using equivalent free gaseous CO2 or ammonium carbamate, implying CO2 activation upon capture with DBN/PEI and glycol.
After that, we further design a tunable ethoxyl-functionalized amidine to absorb CO2 in order to avoid the use of volatile proton donor (Li et al., 2014). As an activated form of CO2, the captured CO2 in the form of zwitterionic amidinium carbonate is further hydrogenated to formate employing RhCl3/DPEphos as the catalyst (Scheme 6). In the same time, we find that the CO2 capture product of potassium phthalimide in PEG150, can also be in situ hydrogenated to formic acid catalyzed by RhCl3·3H2O/CyPPh2 (Scheme 6) (Zhang et al., 2014).
Ru-based catalysts are also promising candidates for the hydrogenation of captured CO2. In the study of Yadav et al., CO2 is captured by DBU and an alcohol to form the alkyl carbonate ionic liquid and the resulting alkyl carbonate is hydrogenated into [DBUH+] formate and methyl formate facilitated by RuCl2(PPh3)3 (Scheme 7) (Yadav et al., 2014). Although the reactive species (i.e., alkyl carbonates or CO2) cannot be identified at this stage, this result indicates that alkyl carbonates may be a substrate for hydrogenation, in addition to free CO2.
In 2015, Sanford group combined CO2 capture to form a carbamate salt with hydrogenation to generate CH3OH (Rezayee et al., 2015). In their study, NHMe2 is used to capture CO2 and a homogeneous Ru-based catalyst is used to facilitate the hydrogenation of the captured CO2 to a mixture of DMF and CH3OH (Scheme 7). Although the formation of carbamate salt can decrease the eletrophilicity of CO2, causing the captured CO2 difficult to hydrogenate, the existence of the equilibrium between DMC (Dimethylammonium dimethylcarbamate) and CO2 allows the release of CO2 possible, thus promoting the CO2 hydrogenation.
By employing pentaethylenehexamine (PEHA) as CO2 absorbent and Ru-based complexes as catalyst, Olah and Surya Prakash group develops a process that combines CO2 capture and the following hydrogenation in an ethereal solvent for the production of MeOH (Kothandaraman et al., 2016a). CO2 from air can be captured by an aqueous solution of PEHA and up to 61% yield of MeOH can be obtained in the triglyme/H2O mixtures at 155°C in the following hydrogenation (Scheme 7). The resulting MeOH can be easily separated by simple distillation from the reaction mixture.
Later, the same group captures CO2 with aqueous amine solution and then in situ hydrogenates the resulting ammonium bicarbonate/carbonate utilizing Ru- and Fe-based pincer complexes in a biphasic solvent system (water/Me-THF) (Kothandaraman et al., 2016b). The superbases (DABCO, TMG, and DBU) shows to be efficient for both CO2 capture and hydrogenation with more than 90% yield of formate under moderate reaction conditions (50 bar H2 at 55°C) (Scheme 7). The biphasic system features easy separation of product and catalyst and the catalyst can be reused for at least five cycles.
Besides homogeneous catalysts, the heterogeneous catalysts were also used in the hydrogenation of CO2 capture products. For example, H. Lin group applies Pd/AC catalyst to the hydrogenation of CO2 capture products originated from ammonia. In the hydrogenation step, the dependence of the activity of CO2 capture products on the solvent is observed (Su et al., 2015a,b). For example, the ammonium bicarbonate in water and ammonium carbamate in 70 wt% ethanol-water solution can offer more than 90% yield of formate under high H2 pressure (5.52 and 2.75 MPa, respectively) at 20°C. The ammonium carbonate presents similar activity with ammonium carbamate. Identification of the species in the reactant solutions suggests the bicarbonate ion and ethyl carbonate ion, instead of the carbamate ion, are the activation forms of CO2 in the hydrogenation (Scheme 8). Coincidently, Enthaler finds that sodium bicarbonate in methanol can be hydrogenated to sodium formate catalyzed by the nickel hydride complex while CO2 cannot be hydrogenated in the identical conditions, which further confirms the activity of the captured CO2 in hydrogenation (Enthaler et al., 2015).
Mertens and coworkers report the in situ hydrogenation of the captured CO2 using Cu/ZnO-Al2O3 as catalyst under retrieval of the CO2 capture reagent N,N-diethylethanolamine (DEEA) (Reller et al., 2014). In the reaction, DEEA can also function as a trapping reagent for the resulting formic acid and drives the hydrogenation forward. The authors find that the generation of the products 2-diethylaminoethylformate and methanol can be regulated by the reaction temperature (Scheme 9). The combination of CO2 capture and hydrogenation realizes the energy integration by using the reaction heat of CO2 hydrogenation in the energy demanding CO2 stripping process.
In addition to the liquid absorption system, the alkali metal and alkali earth metal based solid CO2 adsorbents are also developed (Li et al., 2010, 2011; Lee et al., 2011) and applied in the CCU strategy recently. Duyar et al. design a series of novel dual function materials (DFM) consisting of the catalyst and adsorbent components to couple the endothermic CO2 desorption step with the exothermic hydrogenation of CO2 (Duyara et al., 2016). The results show that DFM with the composition of 5% Ru 10% K2CO3/Al2O3 and 5% Ru 10% Na2CO3/Al2O3 have a methanation capacity of 0.91 and 1.05 g-mol/kg DFM, respectively. Similarly, A. Urakawa group develops the catalyst consisting of earth-abundant chemical elements (FeCrCu/K/MgO–Al2O3), which can trap CO2 from fuel gas in the form of surface carbonates and subsequently hydrogenated the adsorbed CO2 to CO (Bobadilla et al., 2016). Accordingly, these DFMs are identified as promising candidates for CO2 capture and direct utilizations.
Hydrogenation of captured CO2 to energy chemicals can facilitate turning hydrogen gas to liquid fuel as well as realize carbon cycling. Albeit the hydrogenation of captured CO2 has been extensively investigated and various capture reagents and catalysts have been developed, the identification of CO2 activation forms is still controversial. For example, the CO2 capture products alkyl carbonate ammonium salts are considered as the activated CO2 species (Li et al., 2013, 2014; Su et al., 2015a,b). However, the results of Jessop group show that [DBUH][OC(O)OMe] salt is less active than free CO2 when using RuCl(O2CMe)(PMe3)4 as catalyst in MeOH solution (Munshi et al., 2002). Thus, the relationship between the activity of CO2 capture products and the catalyst is still underdeveloped.
It has been verified that N-heterocyclic carbenes and N-heterocyclic olefins can react with CO2, forming the CO2 adduct which can be used as “all-in-one” carboxylating agent (Zhou et al., 2008; Kelemen et al., 2014; Dong et al., 2015; Talapaneni et al., 2015; Finger et al., 2016; Saptal and Bhanage, 2016). For example, Tommasi group shows the CO2 adduct 1-butyl-3-methylimidazolium-2-carboxylate and 1,3-dimethylimidazolium-2-carboxylate behaves as active CO2-carriers and reacted with CH3OH and acetophenone for the synthesis of methylcarbonate and benzoylacetate. The other organic compounds with active hydrogen (acetone, cyclohexanone, benzylcyanide, and propargyl alcohols) can also be carboxylated with these CO2-transfer agents for the synthesis of carboxylates of pharmaceutical interest (Tommasi and Sorrentino, 2005, 2006, 2009) (Scheme 10). Similarly, the transcarboxylation of IPrCO2 (1,3-bis(2,6-diisopropylphenyl)-imidazolum-2-carboxylate) to acetophenone with NaBPh4 to yield sodium benzoylacetate and direct dicarboxylation of MeCN using ItBuCO2 (1,3-bis(tert-butyl)-imidazolium-2-carboxylate) are also reported (Van Ausdall et al., 2011) (Scheme 10).
The transcarboxylation capacity of NHOs-CO2 adducts has also been verified by 1-ethyl-3-methyl-imidazolium-2-methylenecarboxylate through realizing the C-C coupling of CO2 and MeCN (Scheme 10) (Finger et al., 2016). In this transcarboxylation process, the basicity of NHOs should be strong enough to abstract proton from the CH acid.
As highly efficient carboxylating agents, the NHC-CO2 and NHO-CO2 complexes can be easily obtained by reacting NHCs or NHOs with atmospheric CO2. However, instead of serving as absorbent, NHCs and NHOs are usually used as catalysts to promote the conversion of pure CO2 by forming transient NHC-CO2 and NHO-CO2 complexes (Kayaki et al., 2009; Zhou et al., 2017). The reason is that NHCs and NHOs are sensitive to air and moisture thus they cannot be used as absorbents for CO2 in air and industry exhaust. Nowadays, it is found that the imidazolium ionic liquids containing basic anion can absorb CO2, producing imidazolium carboxylates (Gurau et al., 2011; Wang and Wang, 2016). Considering the imidazolium ionic liquids are stable to air and moisture, it opens a new way for the utilization of NHC-CO2 and NHO-CO2 complexes in CCU strategy.
Ionic liquids (ILs) offer a new opportunity for developing novel CO2 capture reagents (Huang and Rüther, 2009; Gurkan et al., 2010; Wang et al., 2011; Yang and He, 2014). Especially, the active site-containing ionic liquids can trap and activate CO2 through chemical absorption. Besides, IL can also function as catalyst in CO2 transformation (Lang et al., 2016; Zhang et al., 2017; Xia et al., 2018). Therefore, it is promising to combine the multiple roles of ILs in CCU strategy. Up to now, cyclocarbonates, oxazolidinones and quinazoline-2,4-(1H,3H)-diones have been synthesized using ILs as CO2 absorbents and catalysts.
Wang group performs a series of investigation on ILs-based CO2 capture and conversion. For example, they design bifunctionalized ionic liquids to capture and simultaneously fix CO2 in the simulation of fuel gas to cyclic carbonates (Scheme 11) (Luo et al., 2016). The cation can capture CO2 and the anion I− can activate the substrate to facilitate CO2 insertion. In the presence of a small amount of water, the yield of product can be improved, making this reaction more applicable to industrial exhaust.
Later, the same group finds that the basicity of anion of ILs is very important for CO2 capture and transformation. A hydroxyl functionalized aprotic ionic liquid shows high efficiency in synthesis of quinazoline-2,4(1H,3H) -diones from atmospheric CO2. The captured CO2 instead of atmospheric CO2 is also used and only 13% yield is obtained, being ascribed to the strong interaction between [Im]− and CO2 (Scheme 11) (Shi et al., 2018). They also demonstrates the feasibility of using captured CO2 as starting material in their another report, where the CO2 captured by azole-type anion [DEIm]− renders a high yield of alkylidene carbonates in the carboxylative cyclization of propargyl alcohol due to the weak interaction between CO2 and the anion (Scheme 11) (Chen et al., 2016).
Liu group reports that azole-anion-based ILs with the [Bu4P]+ cation can capture CO2. With appropriate substrates, the forming carbamate intermediates can be transformed into the α-alkylidenecyclic carbonate, quinazoline-2,4(1H,3H)-diones and benzimidazolone without other catalysts (Scheme 11) (Zhao et al., 2016). Later, the same group reveals that a series of tetrabutylphosphonium ([Bu4P]+)-based ILs with multiple-site for CO2 capture and activation in their anions can be used in CO2 capture and conversion, wherein the IL [Bu4P]3[2,4-OPym-5-Ac] shows the optimal performance in preparation of α-alkylidene cyclic carbonates from propargylic alcohol substrate (Wu et al., 2017). The resulting polycarbonates derived from CO2 and the anion is proved to be the key intermediate in this reaction (Scheme 11).
The ILs [HDBU][MIm] and [HDBU][TFE] can capture CO2 and also show catalytic activity to the reaction of CO2 and propargylic amines for the synthesis of 2-oxazolidinones and the reaction of CO2 with 2-aminobenzonitrile derivatives to synthesis quinazoline-2,4-(1H,3H)-diones. By enhancing the mass transfer with gas-liquid laminar flow continuous-flow microreactor, the simultaneous capture and fixation CO2 to 2-oxazolidinones and quinazoline-2,4-(1H,3H)-diones is realized (Scheme 11) (Vishwakarma et al., 2017).
Due to the dual function as CO2 absorbents and conversion catalysts, ionic liquids can realize the transformation of captured CO2 with several kinds of substrates. Furthermore, the non-volatility characteristic of ionic liquids can facilitate product separation. Thus, the ionic liquids are considered as promising absorbents for the CCU process.
The CO2 capture capacity of FLPs has been reported soon after the FLPs concept was in 2006 proposed (Welch et al., 2006; Momming et al., 2009; Travis et al., 2013; Weicker and Stephan, 2015; Wolff et al., 2016). As early as 2010, Stephan group revealed that 1:2 mixtures of PMes3/AlX3 (X = Cl or Br) in bromobenzene can react with CO2, forming CO2 adduct which can be converted to CH3OH with ammonia borane as reductant (Scheme 12) (Ménard and Stephan, 2010). In the same year, Piers group found that CO2 captured by FLP consisting of 2,2,6,6-tetramethylpiperidine (TMP) and B(C6F5)3 can be reduced to methane with triethylsilane (Scheme 12) (Berkefeld et al., 2010). Soon, it is found that the FLP composed by bis-borane 1,2-C6H4(BCl2)2 and PtBu3 can capture CO2 and the forming capture product can be reduced to methanol by reductant such as amine-borane Me2NHBH3 or [C5H6Me4NH2][HB(C6F5)2(C7H11)] (Scheme 12) (Sgro et al., 2012). Wang group reports the FLP comprising of bis(2,4,6-tris(trifluoromethyl)phenyl)borane and a secondary amine (such as HNiPr2 or HNEt2) readily reacts with CO2 at 80°C, affording carbamate boryl esters which can function as an intramolecular FLP to activate H2, affording ammonium borylformate salt and formamide adducts (Scheme 12) (Lu et al., 2013).
Recently, Yan group incorporates FLP acceptor and donor into the styrene-based monomers, respectively to prepare two diblock copolymers consisting of the complementary FLP blocks and common polystyrene block. These two diblock copolymers can bind CO2, forming nanoparticle. The nanoparticle is then used as CO2 reservoir and catalyst to facilitate the formylation of amines with phenylsilane (Scheme 12) (Chen et al., 2018).
The CO2 capture and H2 activation capacity makes FLPs attractive for CCU strategy, especially for the hydrogenation of captured CO2. However, in the current study, the FLPs promoted CO2 hydrogenation encounters difficulty in FLPs regeneration (Ashley et al., 2009). By now, the FLPs are merely used as CO2 capture reagents and reductants are still needed. Thus, the hydrogen activation ability of FLPs hasn't been utilized. Recently, the breakthrough is made by Jazzar and Bertrand group. By combining the copper catalyst and Lewis pair, hydrogenation of carbon dioxide into formate is realized (Romero et al., 2018). Latter, X. Hu and Y. Wu group reports the first catalytic hydrogenation process of CO2 to formate using transition metal free catalyst (B(C6F5)3/M2CO3, M = Na, K, and Cs) (Zhao et al., 2019). These results open new vistas in the field of FLPs facilitated CO2 capture and hydrogenation.
In addition to the CO2 capture and transformation strategy, there are also examples that the CO2 from waste streams can be directly converted by designing catalysts that tolerate to the contaminants in the waste streams such as exogenous water, nitrogen, SO2, amine etc. For example, Williams et al. reports the synthesis of poly(cyclohexylene carbonate) using the power station generated CO2 facilitated by the homogeneous dinuclear Zn or Mg catalysts, which is stable in the presence of contaminants from gas streams (Chapman et al., 2015) (Scheme 13). D'Elia and Basset group develops the combination of early transition metal halides (Y, Sc, Zr) and TBAB to quantitatively convert CO2 from diluted streams and produce cyclic organic carbonates (Barthel et al., 2016). The features of metal-organic frameworks (MOFs) to selectively capture and catalyze CO2 conversion make them a new type of platform for diluted CO2 transformation. Recently, Hong group design and synthesize an acid-base resistant Cu(II)-MOF which can convert CO2 from simulated post-combustion flue gas into corresponding cyclic carbonates (Liang et al., 2017). In direct conversion of diluted CO2, the stability of the catalysts to the contaminants in the gas streams is crucial to the success of the process.
Scheme 13. Homogeneous dinuclear Zn or Mg catalysts used for the production of poly(cyclohexene carbonate).
The past 10 years have witnessed great advances in CO2 capture and in situ conversion. Different CO2 absorbents including inorganic and organic bases, NHCs, and NHOs, ILs, FLPs and polymeric functional materials have been employed in this field. As an emerging field, much effort is still desired to explore the potential conversion of the captured CO2.
Considering amines and CO2 can be involved in diverse reactions, it is hoped that the conversion of ammonia carbamate can be further extended to other valuable products besides isocyanates, carbamates and ureas.
On the other hand, although the hydrogenation of CO2 captured by inorganic/organic bases and the combination of base and alcohol has been investigated, the hydrogenation of CO2 captured by ILs and/or FLPs remains sporadic and underexplored. In light of the successful electrochemical reduction of IL-captured CO2 and hydrogenation of CO2 in ILs (Zhang et al., 2008, 2009; Wesselbaum et al., 2012; Scott et al., 2017), it is reasonable to conclude that the captured CO2 by IL can be hydrogenated by designing appropriate catalyst. Besides, the breakthrough in FLP-mediated CO2 hydrogenation opens new possibilities for FLP-based CO2 capture and transformation (Romero et al., 2018; Zhao et al., 2019).
Another important transformation pathway for CO2 adducts derived from reacting with amines, NHCs, NHOs and ILs is transcarboxylation reaction in the synthesis of cyclocarbonates, oxazolidinones and quinazoline-2,4-(1H,3H)-diones. It is foreseeable that the application of these transcarboxylating reagents will be investigated continuously with the emergence of new CO2 conversion reactions.
Although a plethora of CO2 absorbents has been developed and valuable products can be obtained from the resulting CO2 adducts by different strategy, however, the cost and feasibility must be considered from the viewpoint of industrial application. With this in mind, amines and ionic liquids are considered as promising absorbents for the CCU strategy. For amine absorbents, their features of high absorbing capacity and comerical availability are attractive for industrial application. Besides, the CO2 adducts derived from amines and CO2 are non-volatile liquids or solids, which can be used as starting materials instead of the volatile amines and CO2 gas to develop the gas free progress. For ionic liquid- based CO2 absorbents, the acceptable cost, tunable structure as well as their high CO2 capture capacity make them competent to the commercial CCU process. Moreover, the ionic liquids are nonvolatile and the corrosion of equipment can be avoided by subtly design the structure of ionic liquids, all of which can facilitate the industry operability.
In summary, the CO2 capture and in situ catalytic transformation is still in its infancy. We hope this review can inspire the extensive research on CO2 capture and in situ transformation, which will benefit for the design of efficient CO2 capture and utilization system (CCU) and realize the valorization of diluted CO2 in waste gas streams or directly from the atmosphere.
All authors contributed for the writing of the manuscript. L-NH designed this proposal and determined the contents. H-RL wrote the Abstract, Introduction, Conclusion, and Outlook parts. H-CF wrote the Inorganic/organic bases and Ionic liquids parts. FY wrote N-heterocyclic carbenes and N-heterocyclic olefins and frustrated lewis pairs parts. H-RL and L-NH revised the manuscript.
This research was funded by National Key Research and Development Program (2016YFA0602900), the National Natural Science Foundation of China (21672119).
The authors declare that the research was conducted in the absence of any commercial or financial relationships that could be construed as a potential conflict of interest.
We thank the Ministry of Science and Technology of China (National Key Research and Development Program) and the National Natural Science Foundation of China.
Alexander, O., Grube, T., Schiebahn, S., and Stolten, D. (2015). Closing the loop: captured CO2 as a feedstock in the chemical industry. Energy Environ. Sci. 8, 3283–3297. doi: 10.1039/c5ee02591e
Ashley, A. E., Thompson, A. L., and O'Hare, D. (2009). Non-metal-mediated homogeneous hydrogenation of CO2 to CH3OH. Angew. Chem. Int. Ed. 48, 9839–9843. doi: 10.1002/anie.200905466
Barthel, A., Saih, Y., Gimenez, M., Pelletier, J. D. A., Kühn, F. E., D'Elia, V., et al. (2016). Highly integrated CO2 capture and conversion: direct synthesis of cyclic carbonates from industrial flue gas. Green Chem. 18, 3116–3123. doi: 10.1039/c5gc03007b
Berkefeld, A., Piers, W. E., and Parvez, M. (2010). Tandem frustrated lewis pair/tris(pentafluorophenyl)borane-catalyzed deoxygenative hydrosilylation of carbon dioxide. J. Am. Chem. Soc. 132, 10660–10661. doi: 10.1021/ja105320c
Bobadilla, L. F., Riesco-García, J. M., Penelás-Pérez, G., and Urakawa, A. (2016). Enabling continuous capture and catalytic conversion of flue gas CO 2 to syngas in one process. J. CO2 Util. 14, 106–111. doi: 10.1016/j.jcou.2016.04.003
Chapman, A. M., Keyworth, C., Kember, M. R., Lennox, A. J. J., and Williams, C. K. (2015). Adding value to power station captured CO2: tolerant Zn and Mg homogeneous catalysts for polycarbonate polyol production. ACS Catal. 5, 1581–1588. doi: 10.1021/cs501798s
Chaturvedi, D., and Ray, S. (2006). Versatile use of carbon dioxide in the synthesis of carbamates. Monatsh. Chem. 137, 127–145. doi: 10.1007/s00706-005-0423-7
Chen, K. H., Shi, G. L., Dao, R. N., Mei, K., Zhou, X. Y., Li, H. R, et al. (2016). Tuning the basicity of ionic liquids for efficient synthesis of alkylidene carbonates from CO2 at atmospheric pressure. Chem. Commun. 52, 7830–7833. doi: 10.1039/c6cc02853e
Chen, L., Liu, R. J., and Yan, Q. (2018). Polymer meets frustrated lewis pair: second-generation CO2 -responsive nanosystem for sustainable CO2 conversion. Angew. Chem. Int. Ed. 57, 9336–9340. doi: 10.1002/anie.201804034
Dong, L., Wen, J., and Li, W. Y. (2015). A theoretical investigation of substituent effects on the stability and reactivity of N-heterocyclic olefin carboxylates. Org. Biomol. Chem. 13, 8533–8544. doi: 10.1039/c5ob01021g
Duyara, M. S., Wang, S., Arellano-Treviño, M. A., and Farrauto, R. J. (2016). CO2 utilization with a novel dual function material (DFM) for capture and catalytic conversion to synthetic natural gas: an update. J. CO2 Util. 15, 65–71. doi: 10.1016/j.jcou.2016.05.003
Enthaler, S., Brück, A., Kammer, A., Junge, H., Irran, E., and Gülak, S. (2015). Exploring the reactivity of nickel pincer complexes in the decomposition of formic acid to CO2/H2 and the hydrogenation of NaHCO3 to HCOONa. ChemCatChem 7, 65–69. doi: 10.1002/cctc.201402716
Finger, L. H., Guschlbauer, J., Harms, K., and Sundermeyer, J. (2016). N-heterocyclic olefin-carbon dioxide and -sulfur dioxide adducts: structures and interesting reactivity patterns. Chem. Eur. J. 22, 16292–16303. doi: 10.1002/chem.201602973
Gurau, G., Rodríguez, H., Kelley, S. P., Janiczek, P., Kalb, R. S., and Rogers, R. D. (2011). Demonstration of chemisorption of carbon dioxide in 1,3-dialkylimidazolium acetate ionic liquids. Angew. Chem. Int. Ed. 50, 12024–12026. doi: 10.1002/anie.201105198
Gurkan, B. E., de la Fuente, J. C., Mindrup, E. M., Ficke, L. E., Goodrich, B. F., Price, E. A., et al. (2010). Equimolar CO2 absorption by anion-functionalized ionic liquids. J. Am. Chem. Soc. 132, 2116–2117. doi: 10.1021/ja909305t
Hampe, E. M., and Rudkevich, D. M. (2003). Exploring reversible reactions between CO2 and amines. Tetrahedron 59, 9619–9625. doi: 10.1016/j.tet.2003.09.096
Huang, J. H., and Rüther, T. (2009). Why are ionic liquids attractive for CO2 absorption? An overview. Aust. J. Chem. 62, 298–308. doi: 10.1071/CH08559
Inesi, A., Mucciante, V., and Rossi, L. (1998). A convenient method for the synthesis of carbamate esters from amines and tetraethylammonium hydrogen carbonate. J. Org. Chem. 63, 1337–1338. doi: 10.1021/jo971695y
Kayaki, Y., Yamamoto, M., and Ikariya, T. (2009). N-Heterocyclic carbenes as efficient organocatalysts for CO2 fixation reactions. Angew. Chem. Int. Ed. 48, 4194–4197. doi: 10.1002/anie.200901399
Kelemen, Z., Peter-Szabo, B., Szekely, E., Holloczki, O., Firaha, D. S., Kirchner, B., et al. (2014). An abnormal N-heterocyclic carbene-carbon dioxide adduct from imidazolium acetate ionic liquids: the importance of basicity. Chem. Eur. J. 20, 13002–13008. doi: 10.1002/chem.201402912
Kothandaraman, J., Goeppert, A., Czaun, M., Olah, G. A., and Prakash, G. K. (2016a). Conversion of CO2 from air into methanol using a polyamine and a homogeneous ruthenium catalyst. J. Am. Chem. Soc. 138, 778–781. doi: 10.1021/jacs.5b12354
Kothandaraman, J., Goeppert, A., Czaun, M., Olah, G. A., and Surya Prakash, G. K. (2016b). CO2 capture by amines in aqueous media and its subsequent conversion to formate with reusable ruthenium and iron catalysts. Green Chem. 18, 5831–5838. doi: 10.1039/c6gc01165a
Lang, X. D., Yu, Y. C., Li, Z. M., and He, L. N. (2016). Protic ionic liquids-promoted efficient synthesis of quinazolines from 2-aminobenzonitriles and CO2 at ambient conditions. J. CO2 Util. 15, 115–122. doi: 10.1016/j.jcou.2016.03.002
Lee, J. B., Eom, T. H., Oh, B. S., Baek, J. I., Ryu, J., Jeon, W. S., et al. (2011). CO2 capture from flue gas using potassium-based dry regenerable sorbents. Energy Procedia. 4, 1494–1499. doi: 10.1016/j.egypro.2011.02.016
Li, L., Li, Y., Wen, X., Wang,F., Zhao, N., Xiao, F., et al. (2011). CO2 Capture over K2CO3/MgO/Al2O3 dry sorbent in a fluidized bed. Energy Fuels 25, 3835–3842. doi: 10.1021/ef200499b
Li, L., Wen, X., Fu, X., Wang, F., Zhao, N., Xiao, F., et al. (2010). MgO/Al2O3 sorbent for CO2 capture. Energy Fuels 24, 5773–5780. doi: 10.1021/ef100817f
Li, Y. N., He, L. N., Lang, X. D., Liu, X. F., and Zhang, S. (2014). An integrated process of CO2 capture and in situ hydrogenation to formate using a tunable ethoxyl-functionalized amidine and Rh/bisphosphine system. RSC Adv. 4, 49995–50002. doi: 10.1039/c4ra08740b
Li, Y. N., He, L. N., Liu, A. H., Lang, X. D., Yang, Z. Z., Yu, B., et al. (2013). In situ hydrogenation of captured CO2 to formate with polyethyleneimine and Rh/monophosphine system. Green Chem. 15, 2825–2829. doi: 10.1039/C3GC41265B
Liang, L., Liu, C., Jiang, F., Chen, Q., Zhang, L., Xue, H., et al. (2017). Carbon dioxide capture and conversion by an acid-base resistant metal-organic framework. Nat. Commun. 8:1233. doi: 10.1038/s41467-017-01166-3
Liu, A. H., Ma, R., Song, C., Yang, Z. Z., Yu, A., Cai, Y., et al. (2012). Equimolar CO2 capture by N-substituted amino acid salts and subsequent conversion. Angew. Chem. Int. Ed. 51, 11306–11310. doi: 10.1002/anie.201205362
Lu, Z., Wang, Y., Liu, J., Lin, Y. J., Li, Z. H., and Wang, H. (2013). Synthesis and reactivity of the CO2 adducts of amine/bis(2,4,6-tris(trifluoromethyl)phenyl)borane pairs. Organometallics 32, 6753–6758. doi: 10.1021/om4007246
Luo, X., Chen, K., Li, H., and Wang, C. (2016). The capture and simultaneous fixation of CO2 in the simulation of fuel gas by bifunctionalized ionic liquids. Int. J. Hydrogen Energy 41, 9175–9182. doi: 10.1016/j.ijhydene.2015.12.223
Massoud, S. S, Louka,F. R., Al-Hasan, M. A., Vicenteb, R., and Mautner, F. A. (2015). Magneto-structural properties of carbonato-bridged copper(II) complexes: fixation of atmospheric CO2. N. J. Chem. 39, 5944–5952. doi: 10.1039/c5nj00285k
Ménard, G., and Stephan, D. W. (2010). Room temperature reduction of CO2 to methanol by Al-based frustrated lewis pairs and ammonia borane. J. Am. Chem. Soc. 132, 1796–1797. doi: 10.1021/ja9104792
Momming, C. M., Otten, E., Kehr, G., Frohlich, R., Grimme, S., Stephan, D. W., et al. (2009). Reversible metal-free carbon dioxide binding by frustrated lewis pairs. Angew. Chem. Int. Ed. 48, 6643–6646. doi: 10.1002/anie.200901636
Munshi, P., Main, A. D., Linehan, J. C., Tai, C. C., and Jessop, P. G. (2002). Hydrogenation of carbon dioxide catalyzed by ruthenium trimethylphosphine complexes: the accelerating effect of certain alcohols and amines. J. Am. Chem. Soc. 124, 7963–7971. doi: 10.1021/ja0167856
Pérez, E. R., Santos, R. H. A., Gambardella, M. T. P., Macedo, L. G. M., de Rodrigues-Filho, U. P., Launay, J., et al. (2004). Activation of carbon dioxide by bicyclic amidines. J. Org. Chem. 69, 8005–8011. doi: 10.1021/jo049243q
Pérez, E. R., Silva, M. O., da Costa, V. C., Rodrigues-Filho, U. P., and Franco, D. W. (2002). Efficient and clean synthesis of N-alkyl carbamates by transcarboxylation and O-alkylation coupled reactions using a DBU–CO2 zwitterionic carbamic complex in aprotic polar media. Tetrahedron Lett. 43, 4091–4093. doi: 10.1016/S0040-4039(02)00697-4
Rahman, F. A., Aziz, M. M. A., Saidur, R., Bakar, W. A. W. A., Hainin, M. R., Putrajaya, R., et al. (2017). Pollution to solution: capture and sequestration of carbon dioxide (CO2) and its utilization as a renewable energy source for a sustainable future. Renew. Sust. Energ. Rev. 71, 112–126. doi: 10.1016/j.rser.2017.01.011
Reller, C., Pöge, M., Lißner, A., and Mertens, F. O. R. L. (2014). CO2 capture and hydrogenation in one process step. Environ. Sci. Technol. 48, 14799–14804. doi: 10.1021/es503914d
Rezayee, N. M., Huff, C. A., and Sanford, M. S. (2015). Tandem amine and ruthenium-catalyzed hydrogenation of CO2 to methanol. J. Am. Chem. Soc. 137, 1028–1031. doi: 10.1021/ja511329m
Romero, E. A., Zhao, T., Nakano, R., Hu, X. B., Wu, Y. T., Jazzar, R., et al. (2018). Tandem copper hydride–lewis pair catalysed reduction of carbon dioxide into formate with dihydrogen. Nat. Catal. 1, 743–747. doi: 10.1038/s41929-018-0140-3
Saptal, V. B., and Bhanage, B. M. (2016). N-Heterocyclic olefins as robust organocatalyst for the chemical conversion of carbon dioxide to value-added chemicals. ChemSusChem 9, 1980–1985. doi: 10.1002/cssc.201600467
Scott, M., Molinos, B. B., Westhues, C, Francik, G., and Leitner, W. (2017). Aqueous biphasic systems for the synthesis of formates by catalytic CO2 hydrogenation: integrated reaction and catalyst separation for CO2-scrubbing solutions. ChemSusChem 10, 1085–1093. doi: 10.1002/cssc.201601814
Sgro, M. J., Domer, J., and Stephan, D. W. (2012). Stoichiometric CO2 reductions using a bis-borane-based frustrated lewis pair. Chem. Commun. 48, 7253–7255. doi: 10.1039/c2cc33301e
Shi, G. L., Chen, K. H., Wang, Y. T., Li, H. R., and Wang, C. M. (2018). Highly efficient synthesis of quinazoline-2,4(1H,3H)-diones from CO2 by hydroxyl functionalized aprotic Ionic Liquids. ACS Sustain. Chem. Eng. 6, 5760–5765. doi: 10.1021/acssuschemeng.8b01109
Song, Q. W., Zhou, Z. H., Yin, H., and He, L. N. (2015). Silver(I)-catalyzed synthesis of β-oxopropylcarbamates from propargylic alcohols and CO2 surrogate: a gas-free process. ChemSusChem 8, 3967–3972. doi: 10.1002/cssc.201501176
Su, J., Lu, M., and Lin, H. F. (2015a). High yield production of formate by hydrogenating CO2 derived ammonium carbamate/carbonate at room temperature. Green Chem. 17, 2769–2773. doi: 10.1039/c5gc00397k
Su, J., Yang, L. S., Lu, M., and Lin, H. F. (2015b). Highly efficient hydrogen storage system based on ammonium bicarbonate/formate redox equilibrium over palladium nanocatalysts. ChemSusChem 8, 813–816. doi: 10.1002/cssc.201403251
Talapaneni, S. N., Buyukcakir, O., Je, S. H., Srinivasan, S., Seo, Y., Polychronopoulou, K., et al. (2015). Nanoporous polymers incorporating sterically confined N-heterocyclic carbenes for simultaneous CO2 capture and conversion at ambient pressure. Chem. Mater. 27, 6818–6826. doi: 10.1021/acs.chemmater.5b03104
Tamura, M., Honda, M., Nakagawa, Y., and Tomishige, K. (2014). Direct conversion of CO2 with diols, aminoalcohols and diamines to cyclic carbonates, cyclic carbamates and cyclic ureas using heterogeneous catalysts. J. Chem. Technol. Biotechnol. 89, 19–33. doi: 10.1002/jctb.4209
Tommasi, I., and Sorrentino, F. (2005). Utilisation of 1,3-dialkylimidazolium-2-carboxylates as CO2-carriers in the presence of Na+ and K+: application in the synthesis of carboxylates, monomethylcarbonate anions and halogen-free ionic liquids. Tetrahedron Lett. 46, 2141–2145. doi: 10.1016/j.tetlet.2005.01.106
Tommasi, I., and Sorrentino, F. (2006). Synthesis of 1,3-dialkylimidazolium-2-carboxylates by direct carboxylation of 1,3-dialkylimidazolium chlorides with CO2. Tetrahedron Lett. 47, 6453–6456. doi: 10.1016/j.tetlet.2006.06.106
Tommasi, I., and Sorrentino, F. (2009). 1,3-dialkylimidazolium-2-carboxylates as versatile N-heterocyclic carbene–CO2 adducts employed in the synthesis of carboxylates and α-alkylidene cyclic carbonates. Tetrahedron Lett. 50, 104–107. doi: 10.1016/j.tetlet.2008.10.107
Travis, A. L., Binding, S. C., Zaher, H., Arnold, T. A. Q., Buffet, J. C., and O'Hare, D. (2013). Small molecule activation by frustrated lewis pairs. Dalton Trans. 42, 2431–2437. doi: 10.1039/c2dt32525j
Van Ausdall, B. R., Poth, N. F., Kincaid, V. A., Arif, A. M., and Louie, J. (2011). Imidazolidene carboxylate bound MBPh4 complexes (M = Li, Na) and their relevance in transcarboxylation reactions. J. Org. Chem. 76, 8413–8420. doi: 10.1021/jo201647b
Vishwakarma, N. K., Singh, A. K., Hwang, Y. H., Ko, D. H., Kim, J. O., Babu, A. G., et al. (2017). Integrated CO2 capture-fixation chemistry via interfacial ionic liquid catalyst in laminar gas/liquid flow. Nat. Commun. 8:14676. doi: 10.1038/ncomms14676
Wang, C. M., Luo, X. Y., Luo, H. M., Jiang, D. E., Li, H. R., and Dai, S. (2011). Tuning the basicity of ionic liquids for equimolar CO2 capture. Angew. Chem. Int. Ed. 50, 4918–4922. doi: 10.1002/ange.201008151
Wang, H., Xin, Z., and Li, Y. H. (2017b). Synthesis of ureas from CO2. Top. Curr. Chem. 375:49. doi: 10.1007/s41061-017-0137-4
Wang, P. X., Liu, S., and Deng, Y. Q. (2017a). Important green chemistry and catalysis: non-phosgene syntheses of isocyanates – thermal cracking way. Chin. J. Chem. 35, 821–835. doi: 10.1002/cjoc.201600745
Wang, S., and Wang, X. (2016). Imidazolium ionic liquids, imidazolylidene heterocyclic carbenes, and zeolitic imidazolate frameworks for CO2 capture and photochemical reduction. Angew. Chem. Int. Ed. 55, 2308–2320. doi: 10.1002/anie.201507145
Weicker, S. A., and Stephan, D. W. (2015). Activation of carbon dioxide by silyl triflate-based frustrated lewis pairs. Chem. Eur. J. 21, 13027–13034. doi: 10.1002/chem.201501904
Welch, G. C., Juan, R. R. S., Masuda, J. D., and Stephan, D. W. (2006). Reversible, metal-free hydrogen activation. Science 314, 1124–1126. doi: 10.1126/science.1134230
Wesselbaum, S., Hintermair, U., and Leitner, W. (2012). Continuous-flow hydrogenation of carbon dioxide to pure formic acid using an integrated scCO2 process with immobilized catalyst and base. Angew. Chem. Int. Ed. 51, 8585–8588. doi: 10.1002/ange.201203185
Wolff, N., von, Lefèvre, G., Berthet, J. C., Thuèry, P., and Cantat, T. (2016). Implications of CO2 activation by frustrated lewis pairs in the catalytic hydroboration of CO2: a view using N/Si+ frustrated lewis pairs. ACS Catal. 5, 4526–4535. doi: 10.1021/acscatal.6b00421
Wu, Y. Y., Zhao, Y. F., Li, R. P., Yu, B., Chen, Y., Liu, X. W., et al. (2017). Tetrabutylphosphonium-based ionic liquid catalyzed CO2 transformation at ambient conditions: a case of synthesis of α-alkylidene cyclic carbonates. ACS Catal. 7, 6251–6255. doi: 10.1021/acscatal.7b01422
Xia, S. M., Chen, K. H., Fu, H. C., and He, L. N. (2018). Ionic liquids catalysis for carbon dioxide conversion with nucleophiles. Front. Chem. 6:462. doi: 10.3389/fchem.2018.00462
Yadav, M., Linehan, J. C., Karkamkar, A. J., van der Eide, E., and Heldebrant, D. J. (2014). Homogeneous hydrogenation of CO2 to methyl formate utilizing switchable ionic liquids. Inorg. Chem. 53, 9849–9854. doi: 10.1021/ic501378w
Yang, Z. Z., and He, L. N. (2014). Efficient CO2 capture by tertiary amine-functionalized ionic liquids through Li+-stabilized zwitterionic adduct formation. Beilstein J. Org. Chem. 10, 1959–1966. doi: 10.3762/bjoc.10.204
Yang, Z. Z., He, L. N., Gao, J., Liu, A. H., and Yu, B. (2012). Carbon dioxide utilization with C–N bond formation: carbon dioxide capture and subsequent conversion. Energy Environ. Sci. 5, 6602–6639. doi: 10.1039/c2ee02774g
Yang, Z. Z., He, L. N., Zhao, Y. N., Li, B., and Yu, B. (2011). CO2 capture and activation by superbase/polyethylene glycol and its subsequent conversion. Energy Environ. Sci. 4, 3971–3975. doi: 10.1039/c1ee02156g
Yoshida, M., Komatsuzaki, Y., and Ihara, M. (2008). Synthesis of 5-vinylideneoxazolidin-2-ones by DBU-mediated CO2-fixation reaction of 4-(benzylamino)-2-butynyl carbonates and benzoates. Org. Lett. 10, 2083–2086. doi: 10.1021/ol800663v
Yoshida, M., Mizuguchi, T., and Shishido, K. (2012). Synthesis of oxazolidinones by efficient fixation of atmospheric CO2 with propargylic amines by using a silver/1,8-diazabicyclo[5.4.0]undec-7-ene (DBU) Dual-catalyst system. Chem. Eur. J. 18, 15578–15581. doi: 10.1002/chem.201203366
Yu, B., Cheng, B. B., Liu, W. Q., Li, W., Wang, S. S., Cao, J., et al. (2016). Atmospheric pressure of CO2 as protecting reagent and reactant: efficient synthesis of oxazolidin-2-ones with carbamate salts, aldehydes and alkynes. Adv. Synth. Catal. 358, 90–97. doi: 10.1002/adsc.201500921
Zhang, S., Li, Y. N., Zhang, Y. W., He, L. N., Yu, B., Song, Q. W., et al. (2014). Equimolar carbon absorption by potassium phthalimide and in situ catalytic conversion under mild conditions. ChemSusChem 7, 1484–1489. doi: 10.1002/cssc.201400133
Zhang, Y. G., and Lim, D. S. W. (2015). Synergistic carbon dioxide capture and conversion in porous materials. ChemSusChem 8, 2606–2608. doi: 10.1002/cssc.201500745
Zhang, Z. F., Hu, S. Q., Song, J. L., Li, W. J., Yang, G. Y., and Han, B. X. (2009). Hydrogenation of CO2 to formic acid promoted by a diamine-functionalized ionic liquid. ChemSusChem 2, 234–238. doi: 10.1002/cssc.200800252
Zhang, Z. F., Xie, Y., Li, W. J., Hu, S. Q., Song, J. L., Jiang, T., et al. (2008). Hydrogenation of carbon dioxide is promoted by a task-specific ionic liquid. Angew. Chem. Int. Ed. 47, 1127–1129. doi: 10.1002/ange.200704487
Zhang, Z. G., Fan, F. J., Xing, H. B., Yang, Q. W., Bao, Z. B., and Ren, Q. L. (2017). Efficient synthesis of cyclic carbonates from atmospheric CO2 using a positive charge delocalized ionic liquid catalyst. ACS Sustain. Chem. Eng. 5, 2841–2846. doi: 10.1021/acssuschemeng.7b00513
Zhao, T., Hu, X., Wu, Y., and Zhang, Z. (2019). Hydrogenation of CO2 to formate with H2: transition metal free catalyst based on a lewis pair. Angew. Chem. Int. Ed. 58, 722–726. doi: 10.1002/anie.201809634
Zhao, Y. F., Wu, Y. Y., Yuan, G. F., Hao, L. D., Gao, X., Yang, Z. Z., et al. (2016). Azole-anion-based aprotic ionic liquids: functional solvents for atmospheric CO2 transformation into various heterocyclic compounds. Chem. Asian J. 11, 2735–2740. doi: 10.1002/asia.201600281
Zhou, H., Wang, G. X., and Lu, X. B. (2017). CO2 Adducts of a-Carbon Alkylated N-Heterocyclic olefins:highly active organocatalysts for CO2 chemical transformation. Asian J. Org. Chem. 6, 1264–1269. doi: 10.1002/ajoc.201700152
Keywords: CO2 capture, activation, conversion, in situ catalysis, green chemistry
Citation: Fu H-C, You F, Li H-R and He L-N (2019) CO2 Capture and in situ Catalytic Transformation. Front. Chem. 7:525. doi: 10.3389/fchem.2019.00525
Received: 11 January 2019; Accepted: 09 July 2019;
Published: 24 July 2019.
Edited by:
Hitoshi Ishida, Kitasato University, JapanReviewed by:
Salah S. Massoud, University of Louisiana at Lafayette, United StatesCopyright © 2019 Fu, You, Li and He. This is an open-access article distributed under the terms of the Creative Commons Attribution License (CC BY). The use, distribution or reproduction in other forums is permitted, provided the original author(s) and the copyright owner(s) are credited and that the original publication in this journal is cited, in accordance with accepted academic practice. No use, distribution or reproduction is permitted which does not comply with these terms.
*Correspondence: Hong-Ru Li, bGlob25ncnVAbmFua2FpLmVkdS5jbg==; Liang-Nian He, aGVsbkBuYW5rYWkuZWR1LmNu
Disclaimer: All claims expressed in this article are solely those of the authors and do not necessarily represent those of their affiliated organizations, or those of the publisher, the editors and the reviewers. Any product that may be evaluated in this article or claim that may be made by its manufacturer is not guaranteed or endorsed by the publisher.
Research integrity at Frontiers
Learn more about the work of our research integrity team to safeguard the quality of each article we publish.