- 1Drug Product Design and Development, GlaxoSmithKline, Collegeville, PA, United States
- 2Product and Process Engineering, GlaxoSmithKline, Collegeville, PA, United States
- 3Advanced Manufacturing Technologies, GlaxoSmithKline, Collegeville, PA, United States
Aqueous Two-Phase Systems (ATPSs) have been extensively studied for their ability to simultaneously separate and purify active pharmaceutical ingredients (APIs) and key intermediates with high yields and high purity. Depending on the ATPS composition, it can be adapted for the separation and purification of cells, nucleic acids, proteins, antibodies, and small molecules. This method has been shown to be scalable, allowing it to be used in the milliliter scale for early drug development to thousands of liters in manufacture for commercial supply. The benefits of ATPS in pharmaceutical separations is increasingly being recognized and investigated by larger pharmaceutical companies. ATPSs use identical instrumentation and similar methodology, therefore a change from traditional methods has a theoretical low barrier of adoption. The cost of typical components used to form an ATPS at large scale, particularly that of polymer-polymer systems, is the primary challenge to widespread use across industry. However, there are a few polymer-salt examples where the increase in yield at commercial scale justifies the cost of using ATPSs for macromolecule purification. More recently, Ionic Liquids (ILs) have been used for ATPS separations that is more sustainable as a solvent, and more economical than polymers often used in ATPSs for small molecule applications. Such IL-ATPSs still retain much of the attractive characteristics such as customizable chemical and physical properties, stability, safety, and most importantly, can provide higher yield separations of organic compounds, and efficient solvent recycling to lower financial and environmental costs of large scale manufacturing.
Introduction
The adoption of methods from the scientific literature into industrial applications often follows a period of dormancy. While ATPSs have experienced a recent prolific rise in applications in microfluidics, cellular engineering, bioprinting, and biopatterning since the 2000s (Teixeira et al., 2017), the industrial applications of ATPSs are typically separations and purification, first described in the 1950s (Albertsson, 1956, 1958). Such ATPSs popularized by Albertsson are polymer-polymer or polymer-salt emulsions that have been well-studied for viral (Norrby and Albertsson, 1960; Liu et al., 1998; Effio et al., 2015), cellular (Walter et al., 1976; Sharp et al., 1986; Kumar et al., 2001), nucleic acid (Ribeiro et al., 2002; Gomes et al., 2009; Nazer et al., 2017), protein (Schmidt et al., 1994; Balasubramaniam et al., 2003), and antibody (Desbuquois and Aurbach, 1971; Selber et al., 2004; Rosa et al., 2007b; Azevedo et al., 2009a) separations. Examples can be found for batch (Tavana et al., 2009; Frampton et al., 2011, 2014; Lai et al., 2011) or continuous (Yamada et al., 2004; Nam et al., 2005; SooHoo and Walker, 2009; Tsukamoto et al., 2009; Rosa et al., 2012) processes. While polymer ATPSs are limited to partitioning of macromolecules due to size of the polymers used, the isolation and separation of small molecules (< 900 Daltons) in ATPSs, especially those developed in the pharmaceutical industry, commonly employ ionic liquids (ILs).
Polymer-Based ATPS Separations and Considerations
The mechanism for purification of a material using ATPSs is driven by physical and chemical affinity toward a select phase and the contaminant's affinity toward the other liquid phase. Accurate prediction of partitioning is complicated by several factors. Physical and chemical properties of each phase, such as viscosity, relative volume, density, charge, pH, and volatility are known to impact performance, and thus the choice of polymer or salt is used to tune the systems for effectiveness (Albertsson, 1960). In general, the process of ATPS separations and purification occurs in three major steps: molecular partitioning, physical separation, and isolation of phase of interest (Figure 1). The use of ATPSs was shown to be a high-yield and environmentally sustainable alternative to some current pharmaceutical purification and extraction processes (Chen et al., 2005). Although its adoption in the pharmaceutical industry is relatively early for manufacturing, it currently has its niche applications in pharmaceutical development as well as other industries (Diuzheva et al., 2018; Mocan et al., 2018). Clear documentation of advantages in cost savings, yield and sustainability combined with ease of adoption is needed to ease its widespread use in biopharmaceutical or vaccine manufacturing process (Chen et al., 2005).
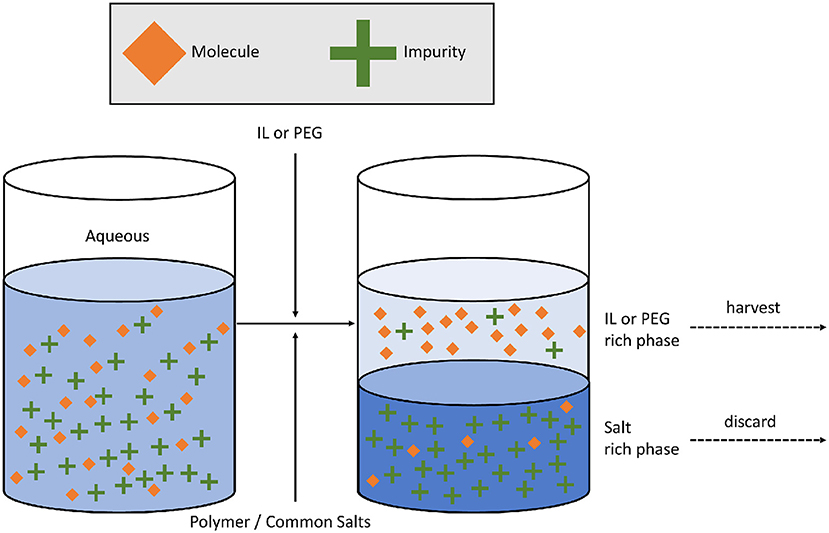
Figure 1. The use of polymer-based or IL-based ATPSs for separations critically depends on selective partitioning of the molecule over impurities. Impeller agitation is often employed to speed up equilibrium partitioning. Depending on viscosity and density differences and interfacial tension values, physical separation can be as simple as sedimentation or as complex as industrial centrifugation to isolate the liquid phase containing the molecule of interest.
Selective partitioning is the first step of separation design. It is advantageous to increase the liquid-liquid interface to accelerate molecular partitioning by producing a fine emulsion of the dispersed aqueous phase inside the continuous aqueous phase. The ability to selectively partition is dependent on the affinity differences for the material of interest for each phase. Polyethylene glycol (PEG) is a widely used component of ATPSs due to its high biocompatibility, biodegradability, water solubility, and low cost. Aside from changes in molecular weight, the properties of PEG with respect to partitioning are limited. To overcome this challenge, multiple groups have functionalized PEG in PEG/salt and PEG/dextran ATPSs with glutaric acid to improve extraction yields of immunoglobins from 28 to 93% (Rosa et al., 2007a), and 23 to 97% (Azevedo et al., 2009b) as well as increasing extraction efficiencies of penicillin up to 96% using imidazole-terminal PEG (Jiang et al., 2009). The addition of a small percentage of a biospecific ligand to ATPSs was shown to increase purification efficiency and yield by several fold (Kula et al., 1991) in a concentration-dependent manner that can be optimized for manufacturing scale of monoclonal antibodies. The use of ATPSs present an opportunity to simplify the manufacturing process of plasmid DNA by allowing for the lysis, recovery, purification and extraction in a single high yield step (Frerix et al., 2005). Polymer ATPSs have been shown to extract plasmid DNA and RNA up to 90 and 70% respectively (Frerix et al., 2006).
The second step is physical coalescence of the emulsion into two continuous, phase-separated, liquids. While numerous physical and chemical properties drive selective partitioning, coalescence of ATPS is simply driven by interfacial tension, density and viscosity. ATPSs will spontaneously coalesce into two continuous phases over time by sedimentation according to Stoke's Laws, and physical characteristics such as viscosity slow the process (Asenjo and Andrews, 2012). Centrifugation is often used to accelerate the natural coalescence and sedimentation.
Lastly, the liquid phase containing the purified material is isolated. The interface between the two liquid phases is avoided to prevent contamination, thus resulting in a yield loss. Multi-stage ATPS separations can improve recovery (Benavides et al., 2006) and may be justifiable depending on the relative ATPS cost and compound value. Vessels with a high aspect ratio are recommended to minimize the liquid-liquid interface where compound loss occurs, however homogenization in a high aspect ratio vessel generally has longer mixing times or suffers from undesirable fluid compartmentalization (Magelli et al., 2013). Furthermore, tall vessels result in long centrifugal distances and longer sedimentation times to achieve complete separation.
Polymer-Based ATPS Pharmaceutical Industry Applications
After fermentation, separation of API from cells and cell debris is often achieved via centrifugation, however centrifugation becomes increasingly complex on scale up from bench (50 mL) to industrial scale (1,000 L) (Majekodunmi, 2015). Thus, there exists a need to explore advanced scalable separation processes that maintain yield for a single-step extraction. Polymer-based ATPSs are showing promise for a wide range of commercially valuable molecules, however their versatility also complicates its adoption. The principles governing high-value molecule partitioning has been investigated (Willauer et al., 2002) and the consultation of such toolboxes (Huddleston et al., 1999) to assist in ATPS design and development is highly encouraged.
One other factor that prohibits the attractiveness of polymer-polymer ATPSs is the cost of high molecular weight polymers at industrial scale (Torres-Acosta et al., 2018). As a result, cheaper phosphate or sulfate salts as replacements for polymer-polymer ATPSs to become polymer-salt ATPSs are more attractive. Such polymer-salt ATPSs were demonstrated to have high yield and scalability from bench to industrial scale (Hart et al., 1994). The substitution of a high molecular weight polymer with a salt, introduces an osmotic pressure concern which limits ATPS application in some cellular and biomolecular recoveries (Kühn, 1980; Zijlstra et al., 1996; Li et al., 2000). Despite this, in certain products with challenging yields, ATPSs have financial justification at the industrial scale especially with the reduced costs of polymer-salt ATPSs and IL-ATPSs (Torres-Acosta et al., 2018).
Ionic Liquid ATPS
Ionic liquids have similar tunable properties such as hydrophobicity, polarity, solvation, and phase separation (Greaves et al., 2006; Moreno et al., 2015; Anderson and Clark, 2018). The stark similarities of polymer-based and IL-based ATPSs have facilitated the adoption of IL-based ATPSs. IL-ATPSs provide improvements in separation performance, selectivity, and broader design opportunities for a diverse range of molecules, including small molecules and biomolecules. IL-ATPSs also possess lower molecular weight and viscosity which enables faster separations making them industrially attractive. We note that the separation of cells, which is frequently performed in drug discovery experiments, and an obvious area for improvement through use of IL-ATPSs is not possible or demonstrated with IL-ATPSs, to the best of our awareness, likely due to osmotic pressure effects.
While this review provides a pharmaceutical focus, the reader is encouraged to reference other IL-ATPS reviews that informatively summarize properties for partitioning (Li et al., 2010; Oppermann et al., 2011; Ma et al., 2018), phase behavior and IL recovery (Freire et al., 2012), enzyme separations (Nadar et al., 2017), deep eutectic solvents (DES) (Shishov et al., 2017; Zainal-Abidin et al., 2017), and capillary electrophoresis applications (El-Hady et al., 2016). A review of various roles of ILs in pharmaceutical applications is also recommended (Huddleston et al., 1998).
Except where noted, all processes, physical properties and performance information described were generated at 25°C.
Types and Properties of IL-ATPS
Phase separation can be controlled by the differing nature of the IL and second phase hydrogen bonding properties (Bridges et al., 2007). The IL-rich environment, often the upper phase, is relatively more hydrophobic thus attracting hydrophobic molecules. Phase separation is thus understood to be solvophobic and driven by electrostatic attractions and hydrophobic interactions. Several different systems have been discovered, characterized, and investigated for their potential applications in the pharmaceutical industry. IL-ATPSs can be tuned to improve solubility and thus extraction efficiency and partitioning properties which in turn improves speed and completion of phase separation. Generally, the lower the salt concentration required, the stronger the phase separation ability of the ILs from aqueous solutions of salts.
IL Cation
Cn-methyl-imidazolium based ILs, where n = 2, 4, 6, and 8, are the most frequent systems studied in the literature, along with other functionalized imidazolium cores (Gutowski et al., 2003; Wu et al., 2015). Other systems such as cholinium based carboxylate ILs (oxalate, malonate, succinate, L-malate, fumarate, glutarate, and citrate), and pyridinium based -ILs are described with PEG 400 and 600 as the second phase former (Passos et al., 2012; Mourão et al., 2014). Varying CH2 length resulted in the coexistence of distinct types of self-assembly in the IL-ATP system. The behavior was inconsistent on changing the IL anion. For example, increasing the imidazolium alkyl chain length increased phase separation for a series of BF ILs and decreased for the same series as Br− ILs (Wei et al., 2013). IL-ATPS and their applications were also demonstrated for dodecyl trimethylammonium-based (Flieger et al., 2013), functionalized amino acid and nucleotide ILs, for example glycine and guanidine (Wu et al., 2013; Zeng et al., 2013; Ding et al., 2014; Yao et al., 2016). Phosphonium-based ILs have the advantage of being less dense than water and less viscous than imidazolium based ILs, thus prospectively facilitating the separation of the aqueous waste stream of biomolecule separations in production scale equipment (Louros et al., 2010).
IL Anion
Several different IL counterions have been described in the IL-ATPS literature, although simple anions such as bromide and chloride are most typical. Amino acid anions, L -serine, L-glycine L-alanine, and L-leucine with [bmim] cations formed IL-ATPS with K3PO4 (Wu C. et al., 2011). The ability to form IL-ATPS was related to hydrophobicity of the amino acid anion such that phase formation occurs with increasing hydrophobicity of the anion. Formate, acetate, propionate, lactate, chloride, and bromide salts of functionalized guanidinium ILs were shown to be effective at protein partitioning (Ding et al., 2014). Antioxidant anions (butanoate, propanoate, acetate, lactate, glycolate, bitartrate, dihydrogen phosphate, chloride, dihydrogen citrate, gallate, syringate, vanillate, and caffeate) of cholinium ILs were effective in separating immunoglobulin G (Ramalho et al., 2018).
ATPS-Forming Salts
IL-ATPSs can be formed with conventional kosmotropic salts (potassium sulfate, citrate, ammonium sulfate), common salts (citric, fumaric, succinic, and tartaric acid sodium salts), amino acids, PEG and surfactants (3-p-nonylphenoxy-2-hydroxypropy trimethyl ammonium bromide, NPTAB and sodium dodecyl sulfonate, SDS), and combinations (Wu C. et al., 2011; Passos et al., 2012; Wei et al., 2013; Abdolrahimi et al., 2015; Dai et al., 2015; Pereira et al., 2015). The size of the biphasic region can be controlled by functionalizing the PEG terminal groups with –OH, –OMe, or –NH2 to change the hydrogen bonding donor or acceptor. Improvements in the extraction performance for a hydrophobic molecule, tryptophan was demonstrated in citrate-based IL-ATPSs for use in continuous extraction of amino acids. The incorporation of surfactants decreased the viscosities of both phases and flipped the IL-rich phase to the bottom phase as a result of density changes (Wei et al., 2013; Dai et al., 2015).
The molecular mechanisms that govern the ability of salt ions to induce IL-ATPS formation was studied using a wide range of salts with diverse combinations of cations and anions, with [bmim][CF3SO3] a hydrophilic ionic liquid (Shahriari et al., 2012). The ions' trend to salt-out the IL into an ATPS follows the Hofmeister series and demonstrates the molar entropy of salt ion hydration as the driving force.
Additives
Addition of a third component to create an IL-ATPS is another tactic used to enhance separation, presumably by increasing the solvophobic nature of the systems. For example, addition of SDS to [bmim]Br/NaCl IL-ATPS enhanced antibiotic extractions into the surfactant-rich upper phase (Yang et al., 2014). Addition of CO2 into aqueous solutions of ILs and amines was shown to be effective in recovering ILs from aqueous mixtures (Xiong et al., 2012). Simply by bubbling CO2 at atmospheric pressure into aqueous IL forms an IL-ATPS and allowed up to 99% recovery of the IL. Interestingly, these systems also modified densities such that the IL-rich phase is at the bottom. They also found phase separation of ammonium salts and the recovery efficiency for ILs are predominantly driven by the pKa of the amine, following the order: 1,2-propylenediamine > monoethanolamine > diethanolamine > N-methylmonoethanolamine > N-ethyldiethanolamine > triethanolamine, except for > N-methyldiethanolamine.
Application of an electrokinetically stable three-phase IL-ATPS, [bmim]Cl/ K2HPO4, [bmim]BF4/ NaH2PO4, and [bmim]BF4/ Na3(citrate) enabled electrokinetic de-mixing (Li et al., 2017). Increased salt concentration and decreased IL concentration were observed to phase separate faster and have a higher efficiency of electrokinetic demixing due to a salting-out effect, whereas electric field had no impact on protein distribution.
In addition, pH-dependent reversible partitioning of IL-ATPS systems at ambient conditions offers dual functionality as a catalytic medium and separation extraction (Ferreira et al., 2017). Adjustment of pH was achieved through changes in salt speciation or bubbling in CO2 or NH3.
IL Extractions
Small Molecule Separations
Several types of small molecules such as antioxidants, flavonoids, alkaloids, sulfonamides, functionalized amino acids, antibiotics such as tetracycline, oxytetracycline, cephalexin, and chloramphenicol effectively partition and extract in IL-ATPSs (Li et al., 2005; Freire et al., 2010; Louros et al., 2010; Han et al., 2011; Berton et al., 2012; Lin et al., 2012; Wu et al., 2013; Mourão et al., 2014; Yang et al., 2014; Abdolrahimi et al., 2015; Yao and Yao, 2017). In general, small molecules partition into the IL-rich upper phase. These systems are advantageous as a sample pre-treatment technique offering reduction of steps, materials and volatiles, and a sample concentration method (Basheer et al., 2008). IL-ATPSs have broad application in selective separation and extraction of small molecules and biomolecules through simple modifications of the IL structure and composition along with parameters that affect the extraction efficiency, such as the salt concentration, pH and extraction temperature, to appropriately modulate the IL-rich phase.
Pei et al. determined the partition coefficients for a series of amino acids in [Cnmim]Br (n = 4, 6, 8)/ K2HPO4 IL-ATPS and showed that they increased with increasing hydrophobicity of the amino acids and ionic liquids, solution pH value, tie-line length of the ATPSs and temperature (Pei et al., 2012). Pre-treatment of aqueous solutions for trace analysis of chloramphenicol with a 147-fold concentration enrichment and high recovery was achieved by applying an electric field across the IL-ATPS (Yao and Yao, 2017). A simple and sensitive method for selective extraction of quinine from human plasma was developed with [bmim]Cl/potassium phosphate buffer (Flieger and Czajkowska-Zelazko, 2015).
Extraction of pharmaceutical molecules (active ingredients or intermediates) is often from plant materials. The isolation of high purity secoisolariciresinol diglucoside, a multi-functional pharmaceutical, from flaxseed, was simplified and improved with an ultrasonic-assisted extraction followed by IL-ATPS formation by the addition of Na2SO4 (Tan et al., 2015). A similar example where fractionation and recovery of bioactive hydroxycinnamic derivatives from stressed carrot mass using IL-ATPS formed from [emim]acetate/potassium buffers gave a different phenolic ratio than conventional ATPS (Sánchez-Rangel et al., 2016).
Enantiomeric separation of 7 racemic amino acid mixtures was achieved by designing task-specific hydrophilic hexafluorophophate IL-ATPSs (Wu et al., 2015). Enantioselectivity was dependent on IL structure with energetically-favored intermolecular interactions between the IL cation, water and the D-enantiomer, allowing separation of the D-enantiomer to the bottom IL-rich phase. The IL-ATPS extraction efficiency was further improved for D,L-phenylalanine using chiral tropine-based ILs, [CnTropine]proline (n = 2, 3, 4, 5, 6, 7, 8) with Cu(acetate)2 and potassium phosphate buffers (Wu et al., 2015).
Macromolecule Extractions/Analytical Biochemistry
The introduction of IL-ATPSs is a significant advancement in analytical biochemistry in terms of time, materials, complexity and efficiency for purification and separation of macromolecules in aqueous fluids. Due to the high water content in both phases, they are biocompatible media for the extraction and purification of a wide range of biomolecules by offering protection from denaturation. Separation is attributed to electrostatic potential differences between the coexisting phases, hydrophobic, and hydrogen bonding interactions of the biomolecules with the IL components in the upper IL-rich phase, and the salting-out effect.
Proteins, such as hemoglobin, ovalbumin, bovine serum albumin (BSA), bovine hemoglobin proteins, lysozyme, cytochrome c, and trypsin appear to require task-specific ILs per entity for successful separation and efficiencies as high as 99.6% (Lin et al., 2013; Wu et al., 2013; Zeng et al., 2013; Ding et al., 2014; Wang et al., 2015; Xu et al., 2015; ČíŽová et al., 2017). Extraction efficiencies are influenced by the amount of IL, the concentration of salt solution, temperature, and protein concentration. Separations were driven by hydrophobic interactions between IL cations and the proteins with minor contributions from electrostatic interactions and the salting-out effect.
In addition to separation, IL-ATPS were also used to improve binding constant estimation by stabilizing the protein of interest (El-Hady et al., 2015; Abd El-Hady and Albishri, 2018). For example, binding constants of (+)-propranolol, methotrexate and vinblastine to human alpha (a1)-acid glycoprotein, the plasma protein were determined by combining affinity capillary electrophoresis with reversible temperature-dependent phase separation using [bmim]Cl/ phosphate IL-ATPS with improved precision.
The antibody, immunoglobulin G (IgG) was extracted from serum samples using cholinium based IL-ATPSs (Ramalho et al., 2018). Although some systems had superior IgG recovery values to conventional techniques, improvement in IgG purity levels was insignificant. IL-ATPS represents a far simpler separation method when compared to current multistep methods for antibody isolation from serum samples. Such technology is worth further development and optimization.
Catalytic Enhancement
IL-ATPS also found utility as catalyst performance enhancers that offer a dual advantage in synthetic processes when selective separation is considered (Dyson et al., 2001; Wu D. X.et al., 2011; Mai and Koo, 2014). The bioconversion of the steroid 16a,17-epoxyprogesterone to 11a-hydroxy-16a, 17-epoxyprogesterone catalyzed by free R. nigricans cells was improved when performed in an IL-ATPS without the addition of salt, and demonstrated in semi-continuous production for 60 h at ~5 mL (total) scale (Wu D. X.et al., 2011). A temperature-dependent reversible IL-ATPS was demonstrated as a medium for transition metal catalyzed hydrogenation of a water-soluble substrate, offering potential re-use of the high-value catalyst and avoidance of product metal contamination (Dyson et al., 2001). Similarly, temperature-controlled reversible phase separation offers the advantage of product recovery and maintaining catalytic activity of an in-situ penicillin G hydrolysis (Mai and Koo, 2014). In keeping with the well-known trends of enzymatic activity in ILs, catalytic activity was found to increase in IL hydrophobicity.
Conventional and IL-ATPS Crossover
There are a few examples of note where polymer-based ATPS were combined with ILs. Extraction efficiency of three antioxidants, gallic, vanillic, and syringic acids, was further improved by tuning the polarity of the PEG-rich bottom phase with the addition of an IL (Almeida et al., 2014). The relationship between hydrogen bonding of the IL with the phenolic acids and extraction was found to be more important than the total amount of IL and increasing the polymer molecular weight increased the likelihood of phase separation. Tropine-salt IL-ATPSs were used to remove or recover hydrophilic ILs that cannot form IL-ATPSs with kosmotropic salts, polymers or carbohydrates, in an attempt to provide a means to reduce environmental contamination (Wu et al., 2016). The ILs preferentially partitioned to the tropine-rich upper phase, which is then further processed to complete the recovery. IL-ATPSs have also been employed for polymer recovery and recycling (Jiang et al., 2009) which offers the benefits of cost and carbon footprint reduction and may be key to wider industrial adoption (Figure 2).
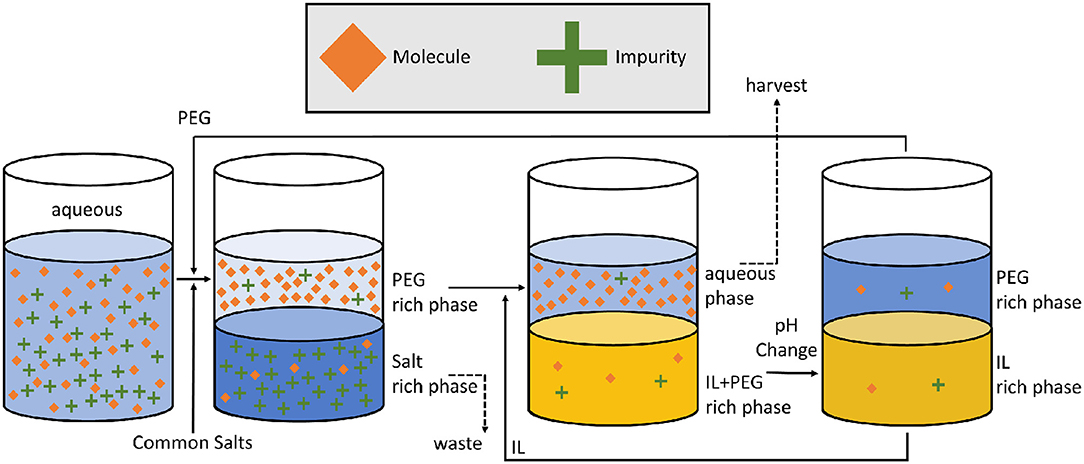
Figure 2. IL assisted polymer recovery for ATPS extraction proposed by Jiang et al. (2009). The recent merge between IL and conventional ATPSs creates new opportunities to further decrease the carbon footprint of ATPS extractions which also result in cost savings. Such financial and environment drivers are key to industrial adoption.
Forward Statement
ATPSs have already found industrial use in niche areas of pharmaceutical development and possibly manufacturing. Meanwhile, ILs are simultaneously emerging as sustainable alternatives for small molecule pharmaceutical extractions. The recent development of IL-based ATPSs provides new opportunities for decreasing the carbon footprint and costs of pharmaceutical extractions which are important drivers for wider industrial adoption. The body of literature is clear that IL-based and polymer-based ATPSs are suitable sustainable alternatives to organic solvents and produce higher or equivalent yields. We believe the barriers to industrial adoption have been cracked due, in part, to the scientific works highlighted in this review, and that there is a bright pharmaceutical future for polymer and IL-based ATPSs.
Author Contributions
LM provided review on ILs and DL provided review on polymer-based ATPS processes.
Conflict of Interest Statement
LM and DL are employed by GlaxoSmithKline.
Acknowledgments
The authors would like to acknowledge their colleagues of GlaxoSmithKline for their support in writing this article, specifically Kenneth Wells, Philip Dell'Orco and Alex Povey for their review and insights.
References
Abd El-Hady, D., and Albishri, H. M. (2018). Temperature controlled ionic liquid aqueous two phase system combined with affinity capillary electrophoresis for rapid and precise pharmaceutical-protein binding measurements. Methods. 146, 120–125. doi: 10.1016/j.ymeth.2018.02.007
Abdolrahimi, S., Nasernejad, B., and Pazuki, G. (2015). Influence of process variables on extraction of Cefalexin in a novel biocompatible ionic liquid based-aqueous two phase system. Phys. Chem. Chem. Phys. 17, 655–669. doi: 10.1039/C4CP02923B
Albertsson, P. A. (1958). Partition of proteins in liquid polymer–polymer two-phase systems. Nature. 182. 709–711. doi: 10.1038/182709a0
Albertsson, P. E. R. Å. (1956). Chromatography and partition of cells and cell fragments. Nature. 177, 771–774. doi: 10.1038/177771a0
Albertsson, P. E. R. Å. (1960). Partition of Cell Particles and Macromolecules. New York, NY: Wiley.
Almeida, M. R., Passos, H., Pereira, M. M., Lima, Á. S., Coutinho, J. A. P., and Freire, M. G. (2014). Ionic liquids as additives to enhance the extraction of antioxidants in aqueous two-phase systems. Sep. Purif. Technol. 128, 1–10. doi: 10.1016/j.seppur.2014.03.004
Anderson, J. L., and Clark, K. D. (2018). Ionic liquids as tunable materials in (bio)analytical chemistry. Anal. Bioanal. Chem. 410, 4565–4566. doi: 10.1007/s00216-018-1125-4
Asenjo, J. A., and Andrews, B. A. (2012). Aqueous two-phase systems for protein separation: phase separation and applications. J. Chromatogr. A. 1238, 1–10. doi: 10.1016/j.chroma.2012.03.049
Azevedo, A. M., Gomes, A. G., Rosa, P. A., Ferreira, I. F., Pisco, A. M., and Aires-Barros, M. R. (2009a). Partitioning of human antibodies in polyethylene glycol–sodium citrate aqueous two-phase systems. Sep. Purif. Technol. 65, 14–21. doi: 10.1016/j.seppur.2007.12.010
Azevedo, A. M., Rosa, P. A. J., Ferreira, I. F., Pisco, A. M. M. O., de Vries, J., Korporaal, R., et al. (2009b). Affinity-enhanced purification of human antibodies by aqueous two-phase extraction. Sep. Purif. Technol. 65, 31–39. doi: 10.1016/j.seppur.2008.03.006
Balasubramaniam, D., Wilkinson, C., Van Cott, K., and Zhang, C. (2003). Tobacco protein separation by aqueous two-phase extraction. J. Chromatogr. A. 989, 119–129. doi: 10.1016/S0021-9673(02)01900-3
Basheer, C., Alnedhary, A. A., Madhava Rao, B. S., Balasubramanian, R., and Lee, H. K. (2008). Ionic liquid supported three-phase liquid-liquid-liquid microextraction as a sample preparation technique for aliphatic and aromatic hydrocarbons prior to gas chromatography-mass spectrometry. J. Chromatogr. A. 1210, 19–24. doi: 10.1016/j.chroma.2008.09.040
Benavides, J., Mena, J. A., Cisneros-Ruiz, M., Ramírez, O. T., Palomares, L. A., and Rito-Palomares, M. (2006). Rotavirus-like particles primary recovery from insect cells in aqueous two-phase systems. J. Chromatogr. B. 842, 48–57. doi: 10.1016/j.jchromb.2006.05.006
Berton, P., Monasterio, R. P., and Wuilloud, R. G. (2012). Selective extraction and determination of vitamin B12 in urine by ionic liquid-based aqueous two-phase system prior to high-performance liquid chromatography. Talanta 97, 521–526. doi: 10.1016/j.talanta.2012.05.008
Bridges, N. J., Gutowski, K. E., and Rogers, R. D. (2007). Investigation of aqueous biphasic systems formed from solutions of chaotropic salts with kosmotropic salts (salt–salt ABS). Green Chem. 9, 177–183. doi: 10.1039/B611628K
Chen, J., Spear, S. K., Huddleston, J. G., and Rogers, R. D. (2005). Polyethylene glycol and solutions of polyethylene glycol as green reaction media. Green Chem. 7, 64-82. doi: 10.1039/b413546f
ČíŽová, A., Korcová, J., Farkaš, P., and Bystrický, S. (2017). Efficient separation of mannan–protein mixtures by ionic liquid aqueous two-phase system, comparison with lectin affinity purification. Int. J. Biol. Macromol. 98, 314–318. doi: 10.1016/j.ijbiomac.2017.02.001
Dai, C., Liu, Y., Wang, S., Du, M., Peng, D., Wang, K., et al. (2015). Investigation on the phase behaviors of aqueous surfactant two-phase systems in a mixture of N-dodecyl-N-methylpiperidinium bromide (C12MDB) and sodium dodecyl sulfate (SDS). Colloi. Surf. A 468, 322–326. doi: 10.1016/j.colsurfa.2014.12.061
Desbuquois, B., and Aurbach, G. (1971). Use of polyethylene glycol to separate free and antibody-bound peptide hormones in radioimmunoassays. J. Clin. Endocrinol. Metab. 33, 732–738. doi: 10.1210/jcem-33-5-732
Ding, X., Wang, Y., Zeng, Q., Chen, J., Huang, Y., and Xu, K. (2014). Design of functional guanidinium ionic liquid aqueous two-phase systems for the efficient purification of protein. Anal. Chim. Acta. 815, 22–32. doi: 10.1016/j.aca.2014.01.030
Diuzheva, A., Carradori, S., Andruch, V., Locatelli, M., De Luca, E., Tiecco, M., et al. (2018). Use of innovative (Micro)extraction techniques to characterise harpagophytum procumbens root and its commercial food supplements. Phytochem. Anal. 29, 233–241. doi: 10.1002/pca.2737
Dyson, P. J., Ellis, D. J., and Welton, T. (2001). A temperature-controlled reversible ionic liquid - Water two phase - Single phase protocol for hydrogenation catalysis. Can. J. Chem. 79, 705–708. doi: 10.1139/v01-084
El-Hady, D. A., Albishri, H. M., Rengarajan, R., Deeb, S. E., and Wätzig, H. (2015). Stabilizing proteins for affinity capillary electrophoresis using ionic liquid aqueous two phase systems: pharmaceuticals and human serum albumin. Electrophoresis. 36, 3080–3087. doi: 10.1002/elps.201500199
El-Hady, D. A., Albishri, H. M., and Wätzig, H. (2016). Ionic liquids in enhancing the sensitivity of capillary electrophoresis: off-line and on-line sample preconcentration techniques. Electrophoresis. 37, 1609–1623. doi: 10.1002/elps.201600069
Ferreira, A. M., Cláudio, A. F. M., Válega, M., Domingues, F. M. J., Silvestre, A. J. D., Rogers, R. D., et al. (2017). Switchable (pH-driven) aqueous biphasic systems formed by ionic liquids as integrated production–separation platforms. Green Chem. 19, 2768–2773. doi: 10.1039/C7GC00157F
Flieger, J., and Czajkowska-Zelazko, A. (2015). Aqueous two phase system based on ionic liquid for isolation of quinine from human plasma sample. Food Chem. 166, 150–157. doi: 10.1016/j.foodchem.2014.06.037
Flieger, J., Siwek, A., Pizon, M., and Czajkowska-Zelazko, A. (2013). Ionic liquids as surfactants in micellar liquid chromatography. J. Sep. Sci. 36, 1530–1536. doi: 10.1002/jssc.201201059
Frampton, J. P., Lai, D., Sriram, H., and Takayama, S. (2011). Precisely targeted delivery of cells and biomolecules within microchannels using aqueous two-phase systems. Biomed. Microdevices. 13, 1043–1051. doi: 10.1007/s10544-011-9574-y
Frampton, J. P., White, J. B., Simon, A. B., Tsuei, M., Paczesny, S., and Takayama, S. (2014). Aqueous two-phase system patterning of detection antibody solutions for cross-reaction-free multiplex ELISA. Sci. Rep. 4:4878. doi: 10.1038/srep04878
Freire, M. G., Cláudio, A. F. M., Araújo, J. M. M., Coutinho, J. A. P., Marrucho, I. M., Lopes, J. N. C., et al. (2012). Aqueous biphasic systems: a boost brought about by using ionic liquids. Chem. Soc. Rev. 41, 4966–4995. doi: 10.1039/c2cs35151j
Freire, M. G., Neves, C.M.S.S., Marrucho, I. M., Canongia Lopes, J. N., Rebelo, L. P. N., and Coutinho, J. A. P. (2010). High-performance extraction of alkaloids using aqueous two-phase systems with ionic liquids. Green Chem. 12, 1715–1718. doi: 10.1039/c0gc00179a
Frerix, A., Müller, M., Kula, M.-R., and Hubbuch, J. (2005). Scalable recovery of plasmid DNA based on aqueous two-phase separation. Biotechnol. Appl. Biochem. 42, 57–66. doi: 10.1042/BA20040107
Frerix, A., Schönewald, M., Geilenkirchen, P., Müller, M., Kula, M.-R., and Hubbuch, J. (2006). Exploitation of the coil-globule plasmid DNA transition induced by small changes in temperature, pH salt, and poly(ethylene glycol) compositions for directed partitioning in aqueous two-phase systems. Langmuir. 22, 4282–4290. doi: 10.1021/la052745u
Gomes, G. A., Azevedo, A. M., Aires-Barros, M. R., and Prazeres, D. M. F. (2009). Purification of plasmid DNA with aqueous two phase systems of PEG 600 and sodium citrate/ammonium sulfate. Sep. Purif. Technol. 65, 22–30. doi: 10.1016/j.seppur.2008.01.026
Greaves, T. L., Weerawardena, A., Fong, C., Krodkiewska, I., and Drummond, C. J. (2006). Protic ionic liquids: solvents with tunable phase behavior and physicochemical properties. J. Phys. Chem. B 110, 22479–22487. doi: 10.1021/jp0634048
Gutowski, K. E., Broker, G. A., Willauer, H. D., Huddleston, J. G., Swatloski, R. P., Holbrey, J. D., et al. (2003). Controlling the aqueous miscibility of ionic liquids: aqueous biphasic systems of water-miscible ionic liquids and water-structuring salts for recycle, metathesis, and separations. J. Am. Chem. Soc. 125, 6632–6633. doi: 10.1021/ja0351802
Han, J., Wang, Y., Yu, C. L., Yan, Y. S., and Xie, X. Q. (2011). Extraction and determination of chloramphenicol in feed water, milk, and honey samples using an ionic liquid/sodium citrate aqueous two-phase system coupled with high-performance liquid chromatography. Anal. Bioanal. Chem. 399, 1295–1304. doi: 10.1007/s00216-010-4376-2
Hart, R. A., Lester, P. M., Reifsnyder, D. H., Ogez, J. R., and Builder, S. E. (1994). Large Scale, In situ Isolation of Periplasmic IGF–I from E. coli. Biotechnology. 12, 1113–1117. doi: 10.1038/nbt1194-1113
Huddleston, J. G., Willauer, H. D., Griffin, S. T., and Rogers, R. D. (1999). Aqueous polymeric solutions as environmentally benign liquid/liquid extraction media. Ind. Eng. Chem. Res. 38, 2523–2539. doi: 10.1021/ie980505m
Huddleston, J. G., Willauer, H. D., Swatloski, R. P., Visser, A. E., and Rogers, R. D. (1998). Room temperature ionic liquids as novel media for ‘clean' liquid–liquid extraction. Chem. Commun. 1765–1766.
Jiang, Y., Xia, H., Yu, J., Guo, C., and Liu, H. (2009). Hydrophobic ionic liquids-assisted polymer recovery during penicillin extraction in aqueous two-phase system. Chem. Eng. J. 147, 22–26. doi: 10.1016/j.cej.2008.11.012
Kühn, I. (1980). Alcoholic fermentation in an aqueous two-phase system. Biotechnol. Bioeng. 22, 2393–2398. doi: 10.1002/bit.260221114
Kula, M.-R., Elling, L., and Walsdorf, A. (1991). Investigations of liquid-liquid partition chromatography of proteins. J. Chromatogr. A 548, 3–12. doi: 10.1016/S0021-9673(01)88589-7
Kumar, A., Kamihira, M., Galaev, I. Y., Mattiasson, B., and Iijima, S. (2001). Type-specific separation of animal cells in aqueous two-phase systems using antibody conjugates with temperature-sensitive polymers. Biotechnol. Bioeng. 75, 570–580. doi: 10.1002/bit.10080
Ladd Effio, C, Wenger, L, Ötes, O., Oelmeier, S.A, Kneusel, R, and Hubbuch, J (2015). Downstream processing of virus-like particles: single-stage and multi-stage aqueous two-phase extraction. J. Chromatogr. A 1383, 35–46. doi: 10.1016/j.chroma.2015.01.007
Lai, D., Frampton, J. P., Sriram, H., and Takayama, S. (2011). Rounded multi-level microchannels with orifices made in one exposure enable aqueous two-phase system droplet microfluidics. Lab Chip. 11, 3551–3554. doi: 10.1039/c1lc20560a
Li, C., Ouyang, F., and Bai, J. (2000). Extractive cultivation of Lactococcus lactis. using a polyethylene glycol/MgSO4· 7H2O aqueous two-phase system to produce nisin. Biotechnol. Lett. 22, 843–847. doi: 10.1023/A:1005634626801
Li, S., He, C., Liu, H., Li, K., and Liu, F. (2005). Ionic liquid-based aqueous two-phase system, a sample pretreatment procedure prior to high-performance liquid chromatography of opium alkaloids. J. Chromatogr. B Anal. Technol. Biomed. Life Sci. 826, 58–62. doi: 10.1016/j.jchromb.2005.08.005
Li, X., Liu, Y., and Li, F. (2017). Effects of DC electric field on phase equilibrium and partitioning of ionic liquid-based aqueous two-phase systems. Biochem. Eng. J. 126, 146–154. doi: 10.1016/j.bej.2016.09.008
Li, Z., Pei, Y., Wang, H., Fan, J., and Wang, J. (2010). Ionic liquid-based aqueous two-phase systems and their applications in green separation processes. TrAC Trends Anal. Chem. 29, 1336–1346. doi: 10.1016/j.trac.2010.07.014
Lin, X., Wang, Y., Liu, X., Huang, S., and Zeng, Q. (2012). ILs-based microwave-assisted extraction coupled with aqueous two-phase for the extraction of useful compounds from Chinese medicine. Analyst. 137, 4076–4085. doi: 10.1039/c2an35476d
Lin, X., Wang, Y., Zeng, Q., Ding, X., and Chen, J. (2013). Extraction and separation of proteins by ionic liquid aqueous two-phase system. Analyst 138, 6445–6453. doi: 10.1039/c3an01301d
Liu, C.-L., Kamei, D. T., King, J. A., Wang, D. I. C., and Blankschtein, D. (1998). Separation of proteins and viruses using two-phase aqueous micellar systems. J. Chromatogr. B Biomed. Sci. Appl. 711, 127–138. doi: 10.1016/S0378-4347(98)00013-9
Louros, C. L. S., Cláudio, A. F. M., Neves, C. M. S. S., Freire, M. G., Marrucho, I. M., Pauly, J., et al. (2010). Extraction of biomolecules using phosphonium-based ionic liquids + K3PO4 aqueous biphasic systems. Int. J. Mol. Sci. 11, 1777–1791. doi: 10.3390/ijms11041777
Ma, C., Laaksonen, A., Liu, C., Lu, X., and Ji, X. (2018). The peculiar effect of water on ionic liquids and deep eutectic solvents. Chem. Soc. Rev. 47, 8685–8720. doi: 10.1039/C8CS00325D
Magelli, F., Montante, G., Pinelli, D., and Paglianti, A. (2013). Mixing time in high aspect ratio vessels stirred with multiple impellers. Chem. Eng. Sci. 101, 712–720. doi: 10.1016/j.ces.2013.07.022
Mai, N. L., and Koo, Y. M. (2014). Enzymatic hydrolysis of penicillin and in situ product separation in thermally induced reversible phase-separation of ionic liquids/water mixture. Enzyme Microb. Technol. 63, 34–38. doi: 10.1016/j.enzmictec.2014.05.002
Majekodunmi, S. O. (2015). A review on centrifugation in the pharmaceutical industry. Am. J. Biomed. Eng. 5, 67–78. doi: 10.5923/j.ajbe.20150502.03
Mocan, A., Diuzheva, A., Carradori, S., Andruch, V., Massafra, C., Moldovan, C., et al. (2018). Development of novel techniques to extract phenolic compounds from Romanian cultivars of Prunus domestica L. and their biological properties. Food Chem. Toxicol. 119, 189–198. doi: 10.1016/j.fct.2018.04.045
Moreno, J. S., Jeremias, S., Moretti, A., Panero, S., Passerini, S., Scrosati, B., et al. (2015). Ionic liquid mixtures with tunable physicochemical properties. Electrochim. Acta. 151, 599–608. doi: 10.1016/j.electacta.2014.11.056
Mourão, T., Tomé, L. C., Florindo, C., Rebelo, L. P. N., and Marrucho, I. M. (2014). Understanding the role of cholinium carboxylate ionic liquids in PEG-based aqueous biphasic systems. ACS Sustain. Chem. Eng. 2, 2426–2434. doi: 10.1021/sc500444w
Nadar, S. S., Pawar, R. G., and Rathod, V. K. (2017). Recent advances in enzyme extraction strategies: a comprehensive review. Int. J. Biol. Macromol. 101, 931–957. doi: 10.1016/j.ijbiomac.2017.03.055
Nam, K.-H., Chang, W.-J., Hong, H., Lim, S.-M., Kim, D.-I., and Koo, Y.-M. (2005). Continuous-flow fractionation of animal cells in microfluidic device using aqueous two-phase extraction. Biomed. Microdevices. 7, 189–195. doi: 10.1007/s10544-005-3025-6
Nazer, B., Dehghani, M. R., and Goliaei, B. (2017). Plasmid DNA affinity partitioning using polyethylene glycol–sodium sulfate aqueous two-phase systems. J. Chromatogr. B. 1044, 112–119. doi: 10.1016/j.jchromb.2017.01.002
Norrby, E. C. J., and Albertsson, P. Å. (1960). Concentration of poliovirus by an aqueous polymer two-phase system. Nature 188:1047. doi: 10.1038/1881047a0
Oppermann, S., Stein, F., and Kragl, U. (2011). Ionic liquids for two-phase systems and their application for purification, extraction and biocatalysis. Appl. Microbiol. Biotechnol. 89, 493–499. doi: 10.1007/s00253-010-2933-4
Passos, H., Ferreira, A. R., Cláudio, A. F. M., Coutinho, J. A. P., and Freire, M. G. (2012). Characterization of aqueous biphasic systems composed of ionic liquids and a citrate-based biodegradable salt. Biochem. Eng. J. 67, 68–76. doi: 10.1016/j.bej.2012.05.004
Pei, Y., Li, Z., Liu, L., and Wang, J. (2012). Partitioning behavior of amino acids in aqueous two-phase systems formed by imidazolium ionic liquid and dipotassium hydrogen phosphate. J. Chromatogr. A. 1231, 2–7. doi: 10.1016/j.chroma.2012.01.087
Pereira, J. F. B., Kurnia, K. A., Freire, M. G., Coutinho, J. A. P., and Rogers, R. D. (2015). Controlling the formation of ionic-liquid-based aqueous biphasic systems by changing the hydrogen bonding ability of polyethylene glycol end groups. Chemphyschem. 16, 2219–2225. doi: 10.1002/cphc.201500146
Ramalho, C. C., Neves, C. M., Quental, M. V., Coutinho, J. A., and Freire, M. G. (2018). Separation of immunoglobulin G using aqueous biphasic systems composed of cholinium-based ionic liquids and poly(propylene glycol). J. Chem. Technol. Biotechnol. 93, 1931–1939. doi: 10.1002/jctb.5594
Ribeiro, S., Monteiro, G., Cabral, J., and Prazeres, D. (2002). Isolation of plasmid DNA from cell lysates by aqueous two-phase systems. Biotechnol. Bioeng. 78, 376–384. doi: 10.1002/bit.10227
Rosa, P., Azevedo, A., Sommerfeld, S., Bäcker, W., and Aires-Barros, M. (2012). Continuous aqueous two-phase extraction of human antibodies using a packed column. J. Chromatogr. B 880, 148–156. doi: 10.1016/j.jchromb.2011.11.034
Rosa, P. A., Azevedo, A., Ferreira, I., De Vries, J., Korporaal, R., Verhoef, H., et al. (2007a). Affinity partitioning of human antibodies in aqueous two-phase systems. J. Chromatogr. A 1162, 103–113. doi: 10.1016/j.chroma.2007.03.067
Rosa, P. A., Azevedo, A. M., and Aires-Barros, M. R. (2007b). Application of central composite design to the optimisation of aqueous two-phase extraction of human antibodies. J. Chromatogr. A 1141, 50–60. doi: 10.1016/j.chroma.2006.11.075
Sánchez-Rangel, J. C., Jacobo-Velázquez, D. A., Cisneros-Zevallos, L., and Benavides, J. (2016). Primary recovery of bioactive compounds from stressed carrot tissue using aqueous two-phase systems strategies. J. Chem. Technol. Biotechnol. 91, 144–154. doi: 10.1002/jctb.4553
Schmidt, A., Ventom, A., and Asenjo, J. (1994). Partitioning and purification of α-amylase in aqueous two-phase systems. Enzyme Microb. Technol. 16, 131–142. doi: 10.1016/0141-0229(94)90076-0
Selber, K., Tjerneld, F., Collén, A., Hyytiä, T., Nakari-Setälä, T., Bailey, M., et al. (2004). Large-scale separation and production of engineered proteins, designed for facilitated recovery in detergent-based aqueous two-phase extraction systems. Proc. Biochem. 39, 889–896. doi: 10.1016/S0032-9592(03)00198-5
Shahriari, S., Neves, C. M. S. S., Freire, M. G., and Coutinho, J. A. P. (2012). Role of the Hofmeister series in the formation of ionic-liquid-based aqueous biphasic systems. J. Phys. Chem. B 116, 7252–7258. doi: 10.1021/jp300874u
Sharp, K. A., Yalpani, M., Howard, S. J., and Brooks, D. E. (1986). Synthesis and application of a poly (ethylene glycol)-antibody affinity ligand for cell separations in aqueous polymer two-phase systems. Anal. Biochem. 154, 110–117. doi: 10.1016/0003-2697(86)90503-8
Shishov, A., Bulatov, A., Locatelli, M., Carradori, S., and Andruch, V. (2017). Application of deep eutectic solvents in analytical chemistry. A review. Microchem. J. 135, 33–38. doi: 10.1016/j.microc.2017.07.015
SooHoo, J. R., and Walker, G. M. (2009). Microfluidic aqueous two phase system for leukocyte concentration from whole blood. Biomed. Microdevices 11, 323–329. doi: 10.1007/s10544-008-9238-8
Tan, Z. J., Wang, C. Y., Yang, Z. Z., Yi, Y. J., Wang, H. Y., Zhou, W. L., et al. (2015). Ionic liquid-based ultrasonic-assisted extraction of secoisolariciresinol diglucoside from flaxseed (Linum usitatissimum. L.) with further purification by an aqueous two-phase system. Molecules. 20, 17929–17943. doi: 10.3390/molecules201017929
Tavana, H., Jovic, A., Mosadegh, B., Lee, Q., Liu, X., Luker, K., et al. (2009). Nanolitre liquid patterning in aqueous environments for spatially defined reagent delivery to mammalian cells. Nat. Mater. 8, 736–741. doi: 10.1038/nmat2515
Teixeira, A. G., Agarwal, R., Ko, K. R., Grant-Burt, J., Leung, B. M., and Frampton, J. P. (2017). emerging biotechnology applications of aqueous two-phase systems. Adv. Healthc. Mater. 7:e1701036. doi: 10.1002/adhm.201701036
Torres-Acosta, M. A., Pereira, J. F. B., Freire, M. G., Aguilar-Yáñez, J. M., Coutinho, J. A. P., Titchener-Hooker, N. J., et al. (2018). Economic evaluation of the primary recovery of tetracycline with traditional and novel aqueous two-phase systems. Sep. Purif. Technol. 203, 178–184. doi: 10.1016/j.seppur.2018.04.041
Tsukamoto, M., Taira, S., Yamamura, S., Morita, Y., Nagatani, N., Takamura, Y., et al. (2009). Cell separation by an aqueous two-phase system in a microfluidic device. Analyst. 134, 1994–1998. doi: 10.1039/b909597g
Walter, H., Krob, E. J., and Brooks, D. E. (1976). Membrane surface properties other than charge involved in cell separation by partition in polymer, aqueous two-phase systems. Biochemistry. 15, 2959–2964. doi: 10.1021/bi00659a004
Wang, Z., Pei, Y., Zhao, J., Li, Z., Chen, Y., and Zhuo, K. (2015). Formation of ether-functionalized ionic-liquid-based aqueous two-phase systems and their application in separation of protein and saccharides. J. Phys. Chem. B 119, 4471–4478. doi: 10.1021/jp510984d
Wei, X. L., Wang, X. H., Ping, A. L., Du, P. P., Sun, D. Z., Zhang, Q. F., et al. (2013). Formation and characteristics of aqueous two-phase systems formed by a cationic surfactant and a series of ionic liquids. J. Chromatogr. B Anal. Technol. Biomed. Life Sci. 939, 1–9. doi: 10.1016/j.jchromb.2013.09.006
Willauer, H. D., Huddleston, J. G., and Rogers, R. D. (2002). Solute partitioning in aqueous biphasic systems composed of polyethylene glycol and salt: the partitioning of small neutral organic species. Ind. Eng. Chem. Res. 41, 1892–1904. doi: 10.1021/ie010598z
Wu, C., Wang, J., Li, Z., Jing, J., and Wang, H. (2013). Relative hydrophobicity between the phases and partition of cytochrome-c in glycine ionic liquids aqueous two-phase systems. J. Chromatogr. A 1305, 1–6. doi: 10.1016/j.chroma.2013.06.066
Wu, C., Wang, J., Wang, H., Pei, Y., and Li, Z. (2011). Effect of anionic structure on the phase formation and hydrophobicity of amino acid ionic liquids aqueous two-phase systems. J. Chromatogr. A 1218, 8587–8593. doi: 10.1016/j.chroma.2011.10.003
Wu, D. X., Guan, Y. X., Wang, H. Q., and Yao, S. J. (2011). 11α-Hydroxylation of 16α,17-epoxyprogesterone by Rhizopus nigricans in a biphasic ionic liquid aqueous system. Bioresour. Technol. 102, 9368–9373. doi: 10.1016/j.biortech.2011.07.060
Wu, H., Yao, S., Qian, G., and Song, H. (2016). Development of tropine-salt aqueous two-phase systems and removal of hydrophilic ionic liquids from aqueous solution. J. Chromatogr. A 1461, 1–9. doi: 10.1016/j.chroma.2016.06.081
Wu, H., Yao, S., Qian, G., Yao, T., and Song, H. (2015). A resolution approach of racemic phenylalanine with aqueous two-phase systems of chiral tropine ionic liquids. J. Chromatogr. A 1418, 150–157. doi: 10.1016/j.chroma.2015.09.058
Xiong, D., Wang, H., Li, Z., and Wang, J. (2012). Recovery of ionic liquids with aqueous two-phase systems induced by carbon dioxide. ChemSusChem. 5, 2255–2261. doi: 10.1002/cssc.201200307
Xu, K., Wang, Y., Huang, Y., Li, N., and Wen, Q. (2015). A green deep eutectic solvent-based aqueous two-phase system for protein extracting. Anal. Chim. Acta. 864, 9–10. doi: 10.1016/j.aca.2015.01.026
Yamada, M., Kasim, V., Nakashima, M., Edahiro, J.I., and Seki, M. (2004). Continuous cell partitioning using an aqueous two-phase flow system in microfluidic devices. Biotechnol. Bioeng. 88, 489–494. doi: 10.1002/bit.20276
Yang, X., Zhang, S., Yu, W., Liu, Z., Lei, L., Li, N., et al. (2014). Ionic liquid-anionic surfactant based aqueous two-phase extraction for determination of antibiotics in honey by high-performance liquid chromatography. Talanta. 124, 1–6. doi: 10.1016/j.talanta.2014.02.039
Yao, T., and Yao, S. (2017). Magnetic ionic liquid aqueous two-phase system coupled with high performance liquid chromatography: a rapid approach for determination of chloramphenicol in water environment. J. Chromatogr. A 1481, 12–22. doi: 10.1016/j.chroma.2016.12.039
Yao, T., Yao, S., Tang, D., Jing, L., Wang, D., and Song, H. (2016). Synthesis, magnetism, aqueous-two phase formation and physical properties of novel guanidinium-based magnetic ionic liquids. RSC Adv. 6, 52898–52904. doi: 10.1039/C6RA09879G
Zainal-Abidin, M. H., Hayyan, M., Hayyan, A., and Jayakumar, N. S. (2017). New horizons in the extraction of bioactive compounds using deep eutectic solvents: a review. Anal. Chim. Acta 979, 1–23. doi: 10.1016/j.aca.2017.05.012
Zeng, Q., Wang, Y., Li, N., Huang, X., Ding, X., Lin, X., et al. (2013). Extraction of proteins with ionic liquid aqueous two-phase system based on guanidine ionic liquid. Talanta. 116, 409–416. doi: 10.1016/j.talanta.2013.06.011
Keywords: aqueous two-phase systems, biphasic systems, ionic liquids, pharmaceutical extractions, pharmaceutical separations
Citation: McQueen L and Lai D (2019) Ionic Liquid Aqueous Two-Phase Systems From a Pharmaceutical Perspective. Front. Chem. 7:135. doi: 10.3389/fchem.2019.00135
Received: 24 August 2018; Accepted: 21 February 2019;
Published: 15 March 2019.
Edited by:
John Paul Frampton, Dalhousie University, CanadaReviewed by:
Daniel Kamei, University of California, Los Angeles, United StatesMarcello Locatelli, Università degli Studi G. d'Annunzio Chieti e Pescara, Italy
Copyright © 2019 McQueen and Lai. This is an open-access article distributed under the terms of the Creative Commons Attribution License (CC BY). The use, distribution or reproduction in other forums is permitted, provided the original author(s) and the copyright owner(s) are credited and that the original publication in this journal is cited, in accordance with accepted academic practice. No use, distribution or reproduction is permitted which does not comply with these terms.
*Correspondence: Lisa McQueen, bGlzYS5sLm1jcXVlZW5AZ3NrLmNvbQ==
David Lai, ZGF2aWQueC5sYWlAZ3NrLmNvbQ==