- 1Department of Chemistry, Colorado State University, Fort Collins, CO, United States
- 2Department of Chemistry, Rajabhat Rajanagarindra University, Chachoengsao, Thailand
- 3Cell and Molecular Biology Program, Colorado State University, Fort Collins, CO, United States
- 4Department of Microbiology, Immunology and Pathology, Colorado State University, Fort Collins, CO, United States
51V NMR spectroscopy is used to document, using speciation analysis, that one oxometalate is a more potent growth inhibitor of two Mycobacterial strains than other oxovanadates, thus demonstrating selectivity in its interaction with cells. Historically, oxometalates have had many applications in biological and medical studies, including study of the phase-problem in X-ray crystallography of the ribosome. The effect of different vanadate salts on the growth of Mycobacterium smegmatis (M. smeg) and Mycobacterium tuberculosis (M. tb) was investigated, and speciation was found to be critical for the observed growth inhibition. Specifically, the large orange-colored sodium decavanadate (V10) anion was found to be a stronger inhibitor of growth of two mycobacterial species than the colorless oxovanadate prepared from sodium metavanadate. The vanadium(V) speciation in the growth media and conversion among species under growth conditions was monitored using 51V NMR spectroscopy and speciation calculations. The findings presented in this work is particularly important in considering the many applications of polyoxometalates in biological and medical studies, such as the investigation of the phase-problem in X-ray crystallography for the ribosome. The findings presented in this work investigate the interactions of oxometalates with other biological systems.
Introduction
Polyoxometalates (POMs) are a class of compounds that have been investigated in a range of biological and biomedical systems, as their effects in cell culture studies and in vivo suggest that these compounds have potential for use as therapeutic agents (Moskovitz and Group, 1988; Hill et al., 1990; Rhule et al., 1998; Aureliano and Crans, 2009; Fraqueza et al., 2012; Aureliano et al., 2013, 2016; Wang et al., 2013; Aureliano and Ohlin, 2014; Leon et al., 2014; Kioseoglou et al., 2015; Shah et al., 2015; Sun et al., 2016; Fu et al., 2018; Gumerova et al., 2018). Decavanadate is a homopolyoxometalate anion, and one of the POMs that has been reported to have known biological effects, as documented by studies reported with cells as well as with isolated enzyme systems. (Pluskey et al., 1996; Aureliano and Crans, 2009; Zhai et al., 2009; Fraqueza et al., 2012; Turner et al., 2012; Aureliano et al., 2013; Kioseoglou et al., 2013; Aureliano, 2014, 2016; Aureliano and Ohlin, 2014). Protein crystal structures have been reported for some protein-POM complexes such as those reported between the ribosome and a Dawson oxometallate (Weinstein et al., 1999; Auerbach-Nevo et al., 2005; Bashan and Yonath, 2008; Noeske et al., 2015). Other protein-POM complexes include protein complexes with smaller oxometalates such as decavanadate (Winkler et al., 2017). The limited stability of decavanadate at neutral pH would suggest that hydrolysis intermediates may form and generate stable complexes with proteins or cellular components. Speciation studies are important in this regard, and different species and possibilities must be considered when investigating the mode of action of systems that are not thermodynamically stable (Aureliano and Crans, 2009; Levina et al., 2017a). Even if the speciation is characterized, the active species and mode of action of these complex systems can be non-trivial to interpret (Willsky et al., 1984b, 1985, 2011; Delgado et al., 2005; Crans et al., 2011; Postal et al., 2016; Jakusch and Kiss, 2017). However, a wide range of activities have been reported depending on the protein, biological system or specific vanadium species (Crans, 2000; Crans et al., 2013; Correia et al., 2015; Postal et al., 2016). Recently, it has become clear that compound uptake is critical to the mode of action because many vanadium compounds are modified during the uptake process (Pessoa and Tomaz, 2010; Crans et al., 2011; Le et al., 2017; Levina et al., 2017a). In the case of a large anion such as decavanadate, the question is simply whether the species is too large to enter through protein channels and thus must be taken up through endocytosis or passive transport mechanisms. The alternative possibility is that the uptake is of the smaller vanadium oxovanadates, such as monomeric vanadate, which then oligomerizes to form decavanadate inside the cell. The formation of decavanadate has been demonstrated in yeast (S. cerevisiae) and thus makes this anion a desirable system to understand in greater detail (Willsky et al., 1984b, 1985).
Vanadium is a first-row transition metal ion and is in the group of transition metals that can form POMs (Baes, 1976; Chasteen, 1983; Vilas Boas and Costa Pessoa, 1987; Pope and Müller, 1991; Rehder, 1991; Crans et al., 2004, 2017). Vanadium is particularly prone to forming homopolyoxometalate ions as well (Baes, 1976; Aureliano and Crans, 2009). Indeed, pure crystalline metavanadate and orthovanadate upon dissolution will form several oxovanadate species containing vanadate monomer, V1, vanadate dimer, V2, vanadate tetramer, V4, vanadate pentamer, V5 and decavanadate, V10 (Pettersson et al., 1983, 1985; Crans et al., 1990), Figure 1. Some of these species have been characterized using X-ray crystallography and have been found to interconvert in aqueous solution (Evans, 1966; Crans et al., 1990). However, the specifics of the reactions and their conditions vary. For example, vanadate and oligomeric species containing 2, 4, and 5 vanadium atoms are colorless and rapidly convert at neutral pH, Figure 1A (Crans et al., 1990). In contrast, decavanadate will form rapidly at acidic pH, but is only kinetically stable at neutral pH, Figure 1B (Baes, 1976; Pope and Müller, 1991; Aureliano and Crans, 2009; Crans et al., 2017). Indeed, the kinetic studies have shown that decamer formation is a rapid process and much faster than the V10 decomposition in both neutral and basic solution (Clare et al., 1973a,b; Druskovich and Kepert, 1975; Comba and Helm, 1988; Kustin, 2015; Crans et al., 2017). The decomposition pathways investigated follow several different mechanisms and are dependent on the concentrations of H+ and OH− and the other counter ions present in solution (Clare et al., 1973a,b; Druskovich and Kepert, 1975; Comba and Helm, 1988; Kustin, 2015). Information is needed describing how decavanadate interacts with membrane interfaces and cellular systems, including how decavanadates biological activities compare to monomeric vanadate. Specifically, an attractive alternative mode of action to simple decavanadate binding would be the direct delivery of a vanadium atom from decavanadate to a biomolecule resulting in the dissolution of the decavanadate cluster. The X-ray characterization of decavanadate shows that its dimensions are 5.4 Å × 7.7 Å × 8.3 Å, Figure 1 (Evans, 1966), a large size that cannot be accommodated by many biological transport channels. Therefore, any uptake of decavanadate is likely to be through endocytosis or a passive mechanism. An attractive alternative mode of uptake would involve dissolution of the cluster by direct delivery of vanadium atoms, for example, into a system such as a protein. However, such a mechanism is more difficult to investigate and will require more information regarding the potential interactions of the anion with ligands and interfaces. We have been addressing related questions for some time (Crans et al., 2017) and exploring the interactions of V10 with interfaces (Baruah et al., 2006; Crans et al., 2006, 2017; Samart et al., 2014; Sanchez-Lombardo et al., 2016). Indeed, more information is needed to be able to characterize the process of how the decavanadate converts to the vanadate monomer and smaller clusters, but such processes undoubtedly involve the molecular association of structures that form in solution. The work presented in this manuscript compares the effect of decavanadate and oxovanadates on two mycobacterial species. The results of this comparison are related to the questions of uptake of vanadate and will form the background information on which it will be possible to begin to address issues regarding potential delivery of V-atoms as well as uptake of decavanadate compared to monomeric and oligomeric vanadate.
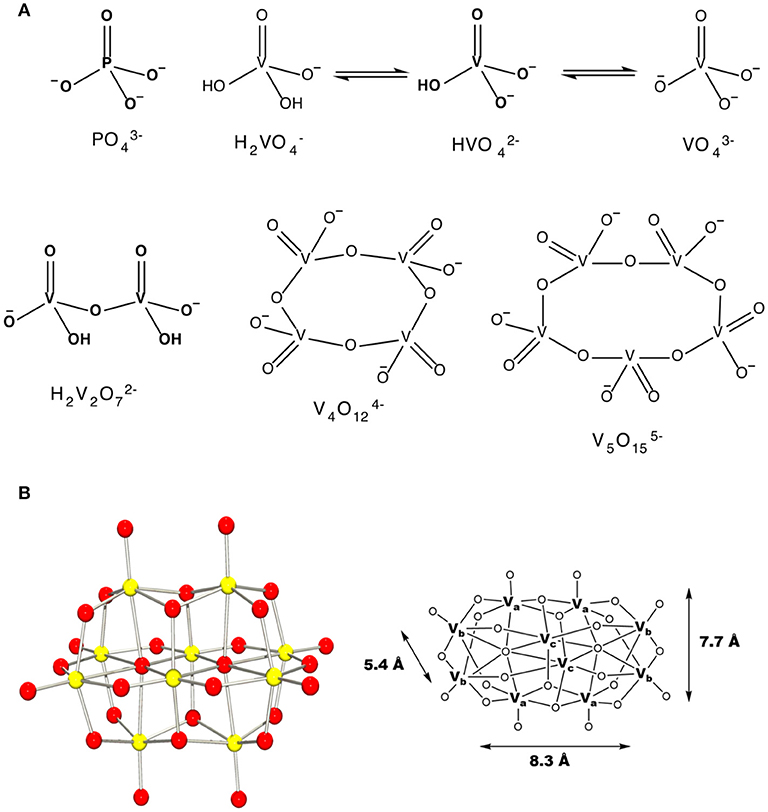
Figure 1. The schematic structures of (A) monomeric vanadate, monomeric and polymeric vanadate series (B) X-ray structure of V10 and the three different types of vanadium atoms in this complex ion with indicated dimensions (Evans, 1966). This figure was adapted with permission from Aureliano and Ohlin (2014), Crans et al. (2017).
Mycobacteria are particularly resistant to hydrophilic drug penetration, and thus studies with these organisms are of general interest. There have been several studies documenting the possibility that vanadium may affect growth of the bacteria, whether in a stimulatory of inhibitory manner; however, these studies did not consider the speciation of the studied compounds in growth media and in the biological context of the cells. For example, previous studies have been carried out investigating the effects of the simple salt vanadate on the cell growth of mycobacteria in the presence and absence of Tween-80 (Costello and Hedgecock, 1959). These studies investigated the possibility that vanadium may be stimulatory to growth because it would be able to replace Fe2+, Fe3+, or biologically active Mo-atoms (Turian, 1951). Dose response curves reported in cultures in the presence of added metavanadate gave complete growth inhibition observed at 5.0 μg/mL V-atom for M. tb (H37Rv), at 10 μg/mL V-atom for Mycobacterium butyricum, at 50 μg/mL V-atom for Mycobacterium phlei, but even at 200 μg/mL V-atom M. smeg was not completely inhibited (Costello and Hedgecock, 1959).
Additionally, studies have been carried out on vanadium coordination complexes and their effect on the growth of mycobacteria (Maiti and Ghosh, 1989; David et al., 2005; Correia et al., 2014; Gajera et al., 2015). The studies explore the possibility that a known drug, or drug derivative complexes binding to vanadium, may exert a greater synergetic activity (Correia et al., 2014; Gajera et al., 2015). For example, in a series of hydroxyquinoline (Correia et al., 2014), fluoroquinoline (Gajera et al., 2015) and acid hydrazides (Martins et al., 2015) vanadium(V) complex studies, the complexes were found with micromolar inhibitory potency against M. tb. In other systems the vanadium complex was found to have little if any effect on the growth of the bacterium (Maiti and Ghosh, 1989; Gajera et al., 2015). Additionally, a series of vanadium thiosemicarbazone and hydroquinoline complexes tested against M. tb were found to have micromolar inhibition constant against growth, but the ligand was more potent than the complex (Correia et al., 2014; Gajera et al., 2015). In these cases, complexation to the vanadium protected the bacterium. Combined these studies demonstrate that vanadium compounds may be inhibitory or protective, depending on the environment and ligands that may be complexed to it, and thus it is of interest to investigate the effects of simple vanadium complexes prior to formation of a coordination complex.
In this work, we determine the effects of decavanadate and the rapidly exchanging oxovanadates on the growth of two mycobacterial species. These studies directly compare the effects of the two vanadium salt species and in doing so, will allow researchers to address the issue of whether such oxometalate species exert different effects, possibly due to uptake or conversion of the salt under physiological conditions. In our studies with M. smeg and M. tb, it was discovered that decavanadate undergoes hydrolysis forming monomeric vanadate in the presence of the cells, suggesting some type of interaction with the bacterial cells or with the material excreted by the bacterial cells. Comparison of metavanadate and decavanadate demonstrates that these two oxovanadate species have very different effects on the growth of these mycobacterial species and depending on species, the potency varies.
Materials and Methods
Materials
Sodium metavanadate (99.9% NaVO3) was purchased from Sigma-Aldrich, hydrochloric acid (36.5–38%, HCl) and citric acid (99% C6H8O7) were purchased from Fischer. Deuterium oxide (D2O, 99.9%) was purchased from Cambridge Isotope Laboratories, Inc. All reagents and other chemicals was purchased from Sigma-Aldrich. Vanadium complexes were prepared according to literature (Samart et al., 2014; Sanchez-Lombardo et al., 2016).
Preparation of Stock Solutions for NMR Spectroscopy Evaluation
Vanadate
Colorless stock solutions of sodium metavanadate (NaVO3, 100 mM) were prepared in deuterium oxide (D2O). The suspension was heated to dissolve the solid, the solution was cooled to room temperature and then the pH was measured (~ pH 7–8) (Crans et al., 1990).
Decavanadate
An orange-red solution of sodium decavanadate (Na6V10O28, 100 mM) was prepared from NaVO3 (1.00 M, 1.22 g). Sodium metavanadate solutions were prepared directly by addition of solid NaVO3 to D2O and heating the solution to dissolve the solid. Once the solution was clear, the pH was adjusted to 4.5–5.8 using 6 M DCl (DCl was prepared from 12 M HCl by the addition of D2O) resulting in an orange colored solution. The V10 content in this solution was verified using 51V NMR spectroscopy and assuming the vanadium was all present as V(V) the concentration of each oxovanadate could be calculated from the integration of the spectra (Crans et al., 1990).
Citric Acid and V-Citrate Complex Solutions
Stock solutions of citric acid (100 mM, pH 2.33) were prepared in deuterium oxide (D2O). The vanadium-citrate complexes were prepared by adding equimolar amounts of citric acid and vanadium. The solution was diluted to form solutions with 75, 50, 10, and 5.0 mM vanadium(V).
Preparation of Stock Solution to Cell Culture Studies
Stock solutions of vanadate and vanadium complexes were prepared in double distilled water (DI H2O), 7H9 media or in 100% DMSO depending on the solubility of these complexes. Specifically, metavanadate, orthovanadate or decavanadate were prepared in double distilled water. Isoniazid (INH) was dissolved in sterile distilled water and used as a positive control.
Nuclear Magnetic Resonance (NMR) Measurements
All 51V NMR spectroscopy measurements were taken on a Bruker spectrometer at 78.9 MHz for 51V (400 MHz for 1H). The chemical shifts were obtained using external reference for 51V NMR of Na3VO4 (100 mM, pH 12.9, signals at −535 (V1) and −559 ppm (V2)). The samples were prepared fresh to form vanadium-citrate complexes in media and the composition was investigated as a function of time with experiments carried out at time points: 0, 1, 5, and 24 h.
Speciation of vanadium was calculated using the integration of the vanadium peak(s) within the 51V NMR spectra. The concentration of each species was determined using the known added concentration [assuming all vanadium is in the form of V(V)] (Crans et al., 1990), the integration of the vanadium peak(s) in the spectrum and by using the mole fractions for each signal, the concentration of each species could be calculated as shown in Table 1.
Speciation Analysis
The interpretation of the 51V NMR spectral data was supplemented by speciation calculations based on constants measured previously (Pettersson et al., 1983; Ehde et al., 1989; Selling et al., 1994). The species distribution diagrams were calculated by using HySS 2009 software (Alderighi et al., 1999; Carsella et al., 2017) and known speciation constants of the system at hand (Pettersson et al., 1983; Ehde et al., 1989; Selling et al., 1994). The citrate and the phosphate concentrations found in the Middlebrook 7H9 broth medium supplemented with 5% BSA, 2% dextrose, 5% catalase (ADC) enrichment, glycerol (0.2%, v/v), and Tween 80 (0.05%, v/v) were 0.48 and 24 mM, respectively (Bbltm, 2018). The vanadium concentrations that were investigated were concentrations of 5, 3.3, and 10 mM. The speciation diagrams were constructed using the following equilibrium reactions for the binary H+-H2 system [see equation (1)] (Pettersson et al., 1983), and two ternary system, H+-H2C6H5 and H+-H2H2 [see equation (2) (Ehde et al., 1989) and (3) (Selling et al., 1994)]. The alternate formulas were provided in the format of the equations provided for comparison.
As described previously, the equations above describe the nature of the complexes that form. For example, H is described as H2 minus H+ and thus the species is described as (−1,1) where the p being −1 (Pettersson et al., 1983; Ehde et al., 1989).
Bacterial Strains and Culture Conditions
Mycobacterium smegmatis mc2155 was grown in 7H9 Middlebrook Medium supplemented with 0.2% (v/v) glycerol, 10% ADC, 0.05% Tween-80, and incubated at 37°C with shaking for 24 h. The growth of bacteria was monitored to mid-logarithmic growth phase using a spectrophotometer at 600 nm to an optical density of 0.6 (OD600nm) (Bbltm, 2018). Metavanadate (40 mM, pH 8.6) and decavanadate [100 mM V10 (1.0 M V-atoms), pH 3.8] stock solutions and the media with bacteria were added to a 96 well plate in a 3-fold dilution experiment monitored at times 0, 1, 5, and 24 h. The pH of these samples ranged from 5.5 to 6.3 before and after treatment with V-compounds and growth; see spectra for in supplemental for details (Upadhyay et al., 2015).
Culture supernatant after cell growth generated for 51V NMR analysis was prepared by running separate growth experiments for the M. smeg mc2 155 experiments with a total volume of 5 mL. At each time point, a 1 mL aliquot was removed for NMR analysis. 51V NMR spectra were run at the time points 0, 1, 5, and 24 h without lock.
M. tb mc2 6230 is a nonpathogenic deletion mutant (ΔRD1 ΔpanCD) strain H37Rv that can safely be used in a Biosafety Level 2 conditions (Sambandamurthy et al., 2006). The bacteria were grown in 7H9 Middlebrook medium with the addition of D-pantothenate (24 mg/L) at 37°C to an optical density at 600 nm (OD600nm) of 0.6–0.8. The cultures were diluted and treated with the appropriate metavanadate and decavanadate stock solutions in a 96 well plate in a 3-fold dilution experiment, and incubated at 37°C for the duration of the experiment (5–7 days). The pH values of these samples ranged from 5.6 to 6.8 after growth for 5 days; see spectra for details.
51V NMR spectra were acquired on culture supernatant without lock (no D2O in sample) at the beginning and end of the bacterial growth experiment (0 and 5 days) for M. tb mc2 6230 growth samples.
Results
Investigating the effects of both vanadate and decavanadate on mycobacterial growth requires information on the speciation of vanadium under the conditions of the growth assay studies. M. tb and M. smeg were grown in supplemented Middlebrook 7H9 medium. Some of the components of the medium may form vanadium complexes. The major candidates for complex formation based on literature formation constants are phosphate (Gresser et al., 1986), citrate (Pettersson et al., 1985; Levina et al., 2017a), and amino acids (Crans, 2000; Rehder et al., 2002; Esbak et al., 2009). In the following, we investigated whether these complexes formed in the media, Figure 1.
Reaction of Vanadate and Decavanadate With Components in Growth Media
51V NMR studies were carried out with vanadate and decavanadate solutions and Middlebrook 7H9 broth medium supplemented with 10% ADC enrichment (5% BSA, 2% dextrose, 5% catalase), glycerol (0.2%, v/v) and Tween 80 (0.05%, v/v). The mycobacteria grow well at pH 6.8, and decavanadate is known to form between pH 3 to 6.5 (Pope and Müller, 1991; Crans et al., 2004; Baruah et al., 2006; Aureliano and Crans, 2009). V10 is formed at low pH values and the V10 hydrolyzes at neutral pH. When V10 is added to the growth media the pH was increased from pH 7.6 to 6.5/7 by the buffering capacity of the growth media and there is a potential for hydrolysis of V10. Importantly, little hydrolysis is observed in growth media in the absence of mycobacteria. NMR spectra were recorded at a range of pH values because the reactions of vanadate are very sensitive to pH (see Supplemenal Material); however, the spectra shown in Figures 2 and 3 are those of direct relevance to observation and assignment of the species that form in the growth media (see below Figure 4). Importantly, all the spectra are referenced against a reference sample (labeled Ref. in Figure 3, containing 100 mM Na3VO4).
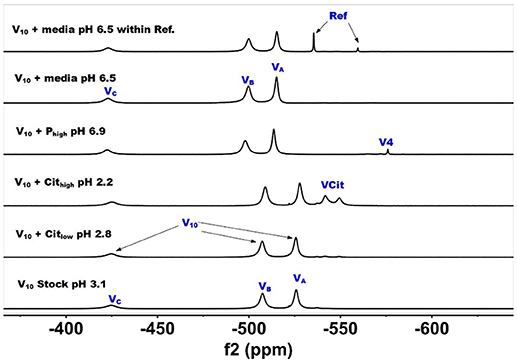
Figure 2. 51V NMR (78.9 MHz) spectra are shown of solution of decavanadate (10 mM V10, 100 mM V-atoms). The samples are from the bottom up diluted V10 stock solution (100 mM V-atom) at pH 3.1; 10 mM V10 in the presence of 0.48 mM and 0.97 mM citrate at pH 2.8 and 2.2, respectively; 10 mM V10 in the presence of 24 mM Pi at pH 6.9; and finally 10 mM V10 in the presence of Middlebrook 7H9 broth medium supplemented with 10% ADC enrichment (5% BSA, 2% dextrose, 5% catalase), glycerol (0.2%, v/v) and Tween 80 (0.05%, v/v) recorded both in the absence and the presence of a capillary reference of 100 mM Na3VO4.
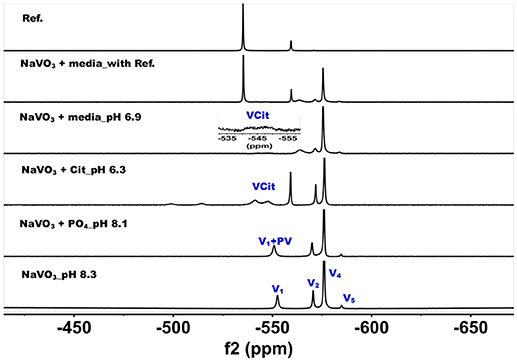
Figure 3. 51V NMR (78.9 MHz) spectra are shown of solution of colorless oxovanadate (40 mM V1, 40 mM V-atoms). The samples are from the bottom up diluted V1 stock solution (40 mM V-atom) at pH 8.3; 10 mM V10 in the presence of 24 mM Pi at pH 8.1; 10 mM V10 in the presence of 0.48 mM citrate at pH 6.3, and finally 10 mM V10 in the presence of Middlebrook 7H9 broth medium supplemented with 10% ADC enrichment (5% BSA, 2% dextrose, 5% catalase), glycerol (0.2%, v/v) and Tween 80 (0.05%, v/v) recorded both in the absence and the presence of a capillary reference of 100 mM Na3VO4. The spectrum labeled Reference is of the capillary reference alone (top spectrum). The key to the signals: V-oligomers, V1 monomer; V2, dimer; V4, tetramer; V5, pentamer; VCit, V-citrate complex; PV, vanadate-phosphate complex.
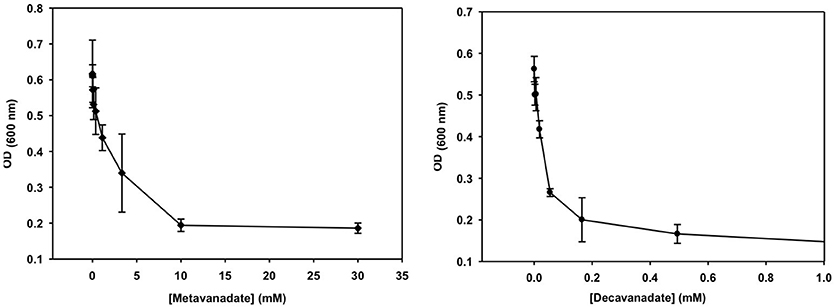
Figure 4. The growth curve is shown for treatment with vanadate (left, prepared from a 40 mM colorless metavanadate solution) and decavanadate [right, prepared from a 100 mM orange decavanadate solution (1.0 M V-atoms) on M. tb].
In Figure 2, we show the spectra of decavanadate under various conditions. First, we show the spectrum of the stock solution at pH 3.1 with the three characteristic signals for the three different V-atoms in the V10 molecule. Next, we show two spectra with added citrate at two concentrations at pH 2.8 and 2.2 where the decavanadate will form VCit complex (two signals at −548 and −552 ppm). The next three spectra at higher pH values are shown first in the presence of phosphate (Pi), demonstrating that the signals for V10 shifts as the pH increases from 3 to 6.9. As shown in the final two spectra in Figure 2, V10 in the presence of media that contains both citrate and Pi did not form any other V-products when all V-atoms are in the form of V10. The spectrum of V10 in media is shown both in the presence and absence of a capillary tube filled with Na3VO4 and used to reference the spectra (Figure 2). All the NMR spectra were referenced in this manner and the other NMR spectra are provided in the Supplemental Material. In summary, these spectra shown that when vanadium is present in the form of V10, other vanadium species do not form in the cell media.
The spectra shown in Figure 2 demonstrated that the V10 is stable in the growth media, which is consistent with studies in yeast media reported previously (Willsky et al., 1984a, 1985). The data shown in Figure 2 demonstrated that at pH 2, the V10 forms complexes with citrate but as the pH increased to near neutral pH, V10 did not form such products with growth media components (Ehde et al., 1989; Zhou et al., 1999; Crans, 2000; Kaliva et al., 2002, 2003; Chen et al., 2007, 2014; Lodyga-Chruscinska et al., 2008). In contrast, when V10 is converted to the oxovanadates or V1 is the starting form at vanadium, the VCit and PV complexes are formed (this is generally observed in solutions at neutral or more basic pH values, Figure 3). However, if the pH of the growth media decreases (that is the acidity increase and pH value decrease), V10 hydrolyzes and forms oxometalates that are able to react with media components (see Figures 2, 3).
The spectra of solutions from metavanadate (NaVO3) containing vanadate monomer and other oxovanadates prepared in aqueous solution and in media are shown in Figure 3. Since vanadate solutions contain rapidly converting species (Crans et al., 1990) and the composition depends on pH and concentration, the species present is dependent on the solution composition. The first spectrum shows a solution containing the interconverting oxovanadates, V1, V2, V4, and V5. In the presence of Pi and at slightly lower pH value, the V1 signal shifted and the linewidth increased, which is indicative of the formation of a vanadate-phosphate complex (abbreviated PV on the spectra in Figure 2) (Gresser et al., 1986; Andersson et al., 2005). Although there are no X-ray structures for these species, the Gresser-Tracey team proposed that there is both a vanadium species that is four or five-coordinate and octahedral based on the chemistry shifts). The formation of the PV species (Gresser et al., 1986; Andersson et al., 2005) is rapid on the NMR time scale, and thus results in shifting of the vanadate monomer (V1) signal in place of observing two separate signals one for V1 and one for PV. As the pH decreased to 6.3, V1 protonates and the monomer signal shifts to about−560 ppm. At these higher pH values, the VCit complex is found to form in solutions containing both V1 and citrate. Many different VCit complexes are known, however, the broad doublet signal observe for the VCit could be several structures as described in the literature (Ehde et al., 1989; Zhou et al., 1999; Crans, 2000; Kaliva et al., 2002, 2003; Chen et al., 2007, 2014; Lodyga-Chruscinska et al., 2008). The final spectra show the addition of the vanadate stock solution to media, which at pH 6.9 results in a solution that contains a signal of V1 and PV, V2, and V4 and a trace of V5. The amount of VCit formed is low and it is difficult to see the signal from in the normal growth media spectrum, so we used an increased amplification to show the VCit signal and part of such spectrum is shown in the spectral insert.
In summary, if the growth of the mycobacteria is conducted near pH 6.8, the speciation of vanadium (V) will readily reach thermodynamic control. Solutions prepared from NaVO3 will contain little to no V10 and solutions containing V10 will be relatively stable. Therefore, growth studies will be carried out with either V1 or V10 species, and it will be possible to determine if there is a difference in the growth effects on mycobacteria by these two species. That is the growth effects of the V1 with the oligomeric oxovanadates and V10 are measured in media with known vanadium speciation and under conditions where it is possible to observe the effect of each species.
Growth Inhibition Experiments of Vanadate Monomer and Decavanadate
Growth inhibition experiments were designed to measure the effects of V10 and V1 each on M. tb mc2 6230 and M. smeg mc2 155. The measurements monitor growth using absorbance at 600 nm and the concentration of the vanadium compound was changing with 3-fold dilution experiments. In this manner, the growth of the bacteria was measured over a 2,000-fold range of concentrations of vanadium compound. The results with the M. tb are shown in Figure 4, and the EC50 (concentration of compound where growth is reduced by half) value calculated for the V1 experiment was 2.0 mM (2.0 mM V-atoms) and for the V10 experiment 29 μM (0.29 mM V-atoms, Table 2).
These results show that V10 is a more potent inhibitor than V1 by a factor of 100 in terms of concentration and a factor of 10 in terms of concentration of V-atoms. This result demonstrates that the large V10 anion is more inhibitory than the V1 atom even when counting for the fact that there are 10 V-atoms in each V10 species. As discussed below, 51V NMR spectroscopy was used to examine the speciation in the solutions and verify that the V10 solution indeed contained V10 (see below for details).
The growth effects were also measured for M. smeg mc2 155 for both V10 and V1 (data not shown). Similarly, the EC50 values were calculated for the V1 experiment to be 190 μM (0.190 mM V-atoms) and for the V10 experiment to be 3.7 μM (0.037 mM V-atoms). These results show that the V10 is a more potent inhibitor than V1, by a factor of 100 in terms of concentration and a factor of 10 in terms of concentration of V-atoms as we also observed for M. tb.
These results present evidence that the large compact V10-anion is a stronger inhibitor than V1 of cell growth of the two mycobacterial strains. The more potent inhibition by V10 compared to V1 shows that the V10 is an inhibitor, and that it is not V1 formed from hydrolyzed V10. However, it should be mentioned that it is solutions of V10 that are causing the inhibitory growth effect, and we cannot rule out the possibility that the effect is caused by several species including the hydrolysis products, or some mechanism in which V10 delivers the V-atom to the cells. Regardless, these studies demonstrate the effects of simple oxovanadates and the large decavanadate. The fact that V10 has a greater effect than V1 (or 10 V1 molecules) supports the interpretation that the growth effects observed are not due to V1 formed from hydrolyzed V10. To support this interpretation, we acquired the 51V NMR spectra of the growth media solutions at various times during the growth experiment.
Speciation Studies in Media of Mycobacteria Treated With Vanadate and Decavanadate
51V NMR Spectra of Media That Have Grown Mycobacteria and Have Been Treated With Metavanadate
51V NMR spectra were recorded at several concentration and time-points in the growth media in which M. smeg had grown because as mentioned above the bacteria were subjected to a 2000-fold concentration range. In Figure 5, we show the 51V NMR spectra recorded at 3.3 mM and 10 mM oxometalates, respectively. Both these series show the presence of the different oxometalates (V1, V2 and V4). In addition, neither series show any evidence for change as the experiment proceeded. This is somewhat surprising, because experiments with other cellular systems, such as yeast, fungi and red blood cells, all showed significant signs of signal reduction (Willsky et al., 1984b, 1985; Crans et al., 2002; Delgado et al., 2005; Jakusch et al., 2014). Since the V1 or oxovanadate is not a strong inhibitor of growth, it is possible that the simple vanadate system is not getting into the M. smeg cells very effectively.
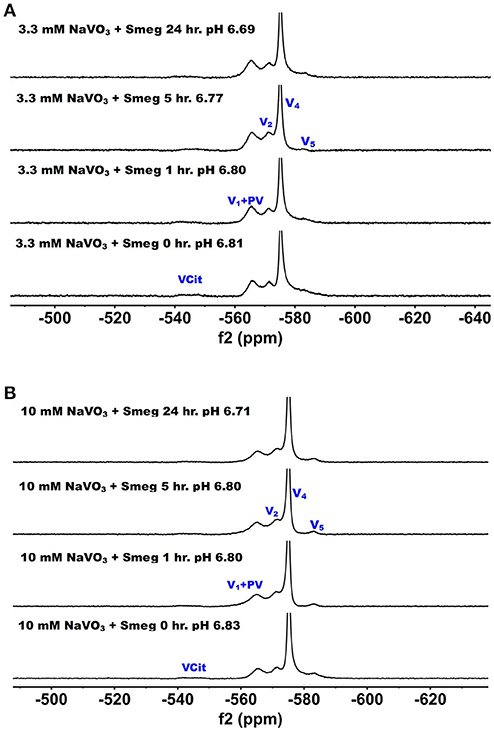
Figure 5. The 51V NMR spectra of media in which M. smeg had grown at 0, 1, 5, and 24 h time points. Data for two concentrations are shown in (A) 3.3 mM V1 treatment (3.3 mM V-atoms) and (B) 10 mM V1 treatment (10 mM V-atoms). See Figure 3 caption for key to labeling.
51V NMR Spectra of Media in Which Mycobacteria Have Been Treated With Decavanadate
51V NMR spectra were recorded at several concentrations and time-points in the growth media in which M. smeg had grown. In Figure 6, we show the 51V NMR spectra recorded at 3.3 and 10 mM, respectively. Both series show the presence of V10. Although the stock solutions and growth media without bacterial cells contains 100% V10 (Figure 2), the addition of growth media containing cells immediately caused some decomposition of V10 (Figure 6). NMR spectra were recorded multiple times of the 3.3 mM V and resulted in approximately a 1:1 ratio of V10 to oxovanadates as determined by integration of the spectra. As shown in Figure 6, after 1 h the composition of the sample was very similar to the content at the beginning of treatment, whereas at the 5 h time point the V10 had decreased significantly and at 24 h very little was left. This demonstrates that the V10 is not stable in the media in the presence of the M. smeg cells at 33 mM total V-atom concentration even though the pH is between 5.8 and 6.8 which is a pH range where there should not have been any hydrolysis of V10 according to the known solution chemistry of V10. However, V10 treatment may cause signal reduction or the V(V) may interact with large structural components in the mycobacterial cells (or their excretion products) and the 51V NMR signal has broadened beyond detection. Precedent for these possibilities has been reported previously (Willsky et al., 1984b, 1985; Crans et al., 2002). In the series of spectra at 10 mM V10 in the media, there is less evidence for V10 removal is apparent at the different time points. However, when we integrated the signals a slow decrease in the V10 signals compared to the oxovanadate signals was clear. The ratio at time zero was 1:0.71, at time 1 h it was 1:1.08; at time 5 h it was 1:1.18 and at 24 h it was 1:1.24. Thus, providing evidence for a slow decrease in V10 content as the experiment progressed. This data is consistent with the possibility that there is some reduction of the vanadium from the POM resulting in hydrolysis and formation of the oxovanadates.
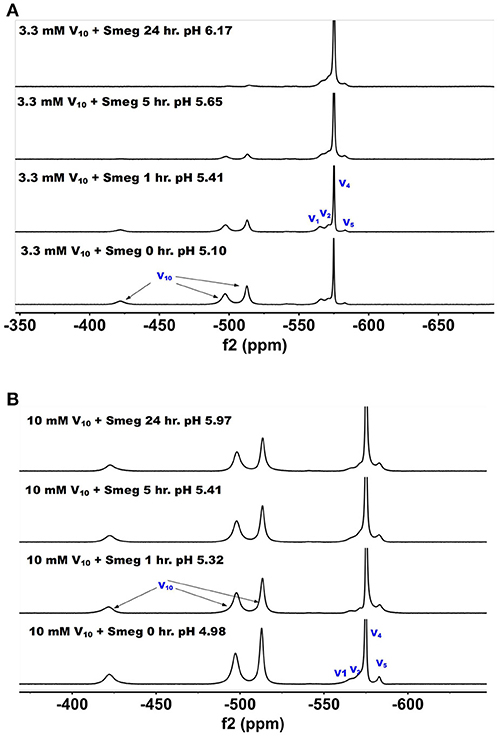
Figure 6. The 51V NMR spectra of media in which M. smeg had grown at 0, 1, 5, and 24 hr time points. Data for two concentrations are shown in (A) 3.3 mM V10 treatment (33 mM V-atoms) and (B) 10 mM V10 treatment (100 mM V-atoms).
To further investigate whether there was any reduction taking place upon vanadium treatment, we also measured the color change in these samples, Table 3. More data and the pH measurements on all the samples shown are provided in the Supplemental Material, and the pH values are all in the range from 6.1 to 7.4. Vanadium(V) on reduction is known to change from colorless (V1 oxovanadates) or yellow/orange (V10) to a green color (Crans et al., 2004, 2010, 2013; Pessoa and Tomaz, 2010; Jakusch et al., 2014). The 7H9 media alone did not cause any reduction as evidenced by the media sample maintained the color prior to treatment, Table 3. All the samples containing media and M. smegmatis treated with V10 developed a greenish tint as the treatment progressed beyond 5 h time points, whereas the samples treated with V1 remained colorless, see Table 3. This is consistent with reduction of some of the V(V) in the V10 salt. Interesting, similar reduction was not observed for the samples treated with the V1 solutions. These observations are consistent with the selective reduction of the V(V) and that the reduction was more prevalent after longer treatments, higher concentrations of vanadium and V10 compared to V1. Since the reduction only took place in the presence of V10, this anion induced a response that was not induced by V1. Furthermore, the supernatant of a batch of grown M. smeg. bacteria and centrifuging down the bacteria and removing them was collected to investigate if the bacteria excrete a component responsible for the observed reduction. The V10 and V1 samples were added to this supernatant and the data are shown in column 4 in Table 3. This supernatant also generate the color change from orange to green upon incubation. This experiment showed that the reduction is something that was induced by a material excreted from the M. smeg. cells during growth. This suggest that the reduction is likely to come from the excretion of siderophores (Hider and Kong, 2010) or proteins such as the reported protein tyrosine phosphatases from mycobacteria (Beresford et al., 2009; Dutta et al., 2016). Since we also carried out such studies of supernatant that had been heated (see Supplemental Material), such a product is either a very heat stable protein or a siderophore-type of material.
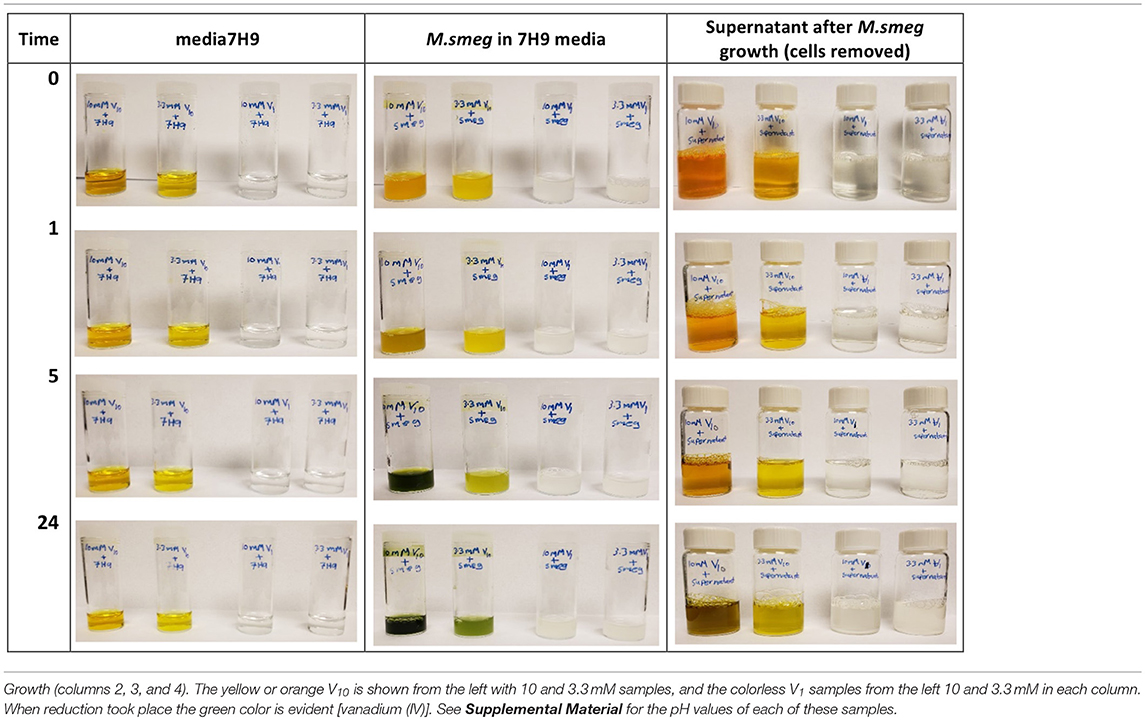
Table 3. Samples treated with V10 and V1 in 7H9 media, M. smeg cultures and supernatant after M. smeg.
Combined these studies show that there is selectivity in the effects of interactions of vanadium with mycobacteria, and that for these systems the most effective form is not the V1, which is a potent phosphatase inhibitor and a common active form of vanadium. These studies show that the V10, a POM is more potent inhibitor of growth of mycobacteria than the well-known phosphatase inhibitor, V1. The studies also demonstrate that there is some redox chemistry involved in this process and that the mycobacteria excrete a component that interact with V10 and cause the redox chemistry and hydrolysis of the V10. Eventually the V1 samples would also show the color change consistent with reduction, but this required growth periods beyond several weeks and this process may be different than the reduction, of V10.
Modeling Speciation Vanadium species Distribution in Mycobacteria Media Added Metavanadate
To quantify the V-species that are present in the growth assay media, we carried out speciation analyses modeling experimentally the distribution of vanadium species in the media. This analysis is based on analyzing solutions containing V-species that are governed by thermodynamics and can be calculated the HySS program (Alderighi et al., 1999). This model can analyze the experimental conditions found in solution prepared by the addition of metavanadate and exposed to the conditions of the cell growth assay. We used the reactions reported previously with regard to the exchange of labile oxovanadates (Pettersson et al., 1983, 1985; Gresser et al., 1986; Ehde et al., 1989; Crans et al., 1990, 2000; Crans, 2000, 2005; Andersson et al., 2005; Baruah et al., 2006; Aureliano and Crans, 2009), the formation of the Vcit complex (Ehde et al., 1989; Crans, 2000), the formation of the PV complex (Gresser et al., 1986; Andersson et al., 2005), and the thermodynamics relating to the decavanadate deprotonation reactions (Crans, 2005; Baruah et al., 2006; Aureliano and Crans, 2009). The known speciation parameters used for vanadate at various pH values were measured in the presence of 0.6 M NaCl to keep the salt concentration constant for all the components in the system. As shown in Figure 5, the species composition for the conditions of three different concentrations of vanadium (0.0050, 3.3, and 10 mM) are illustrated. As discussed above, the vanadium samples prepared with decavanadate will not contain this speciation distribution because all V(V) was converted to V10. The addition of this solution to the media will only slowly hydrolyze the V10 species to the equilibrium oxovanadate mixture if above or near pH 7.
The speciation analysis of the conditions observed in the media with freely exchangeable oxovanadate shown in Figure 3 demonstrate that an observable amount of VCit complex forms. Depending on the concentration of the vanadium, the contribution of VCit is larger percentage-wise at the lower concentrations of vanadate; for example, at 0.005 and 1.0 mM V-atoms at neutral pH the amount is 75–80% (in terms of mole fraction) of VCit complex. In contrast, at 10 mM V-atoms at neutral pH the amount is ~10%. However, if the amounts are calculated, this would correspond to 0.0004, and 1.0 mM VCit complex in the media. These observations are consistent with the very strong formation constant for VCit. The PV complex, however, is much less stable, and even though there is much more phosphate in the assay, the PV complex is only observed in a significant concentration at high V and Pi concentrations (5 and 24 mM, respective). Although the PV complex is only observed in high concentrations at mM V-treatments the shifting of the V1 signal attests to the presence of the PV adduct in the assays with M. tb and M. smeg.
The results shown in Figure 7 are in general agreement with the experimental data obtained in Figures 2, 3, 5, 6 with regard to formation of the VCit and PV complexes. Figure 7 shows that there are four different VCit complexes formed at low vanadium concentration, but at higher concentration and in the presence of phosphate two major VCit complexes formed. There are several assumptions on which these estimations are based, including the differences in ionic strengths, the low concentration of the vanadium used, and the fact that some reduction takes place during the growth experiment to be changing the concentrations somewhat of the VCit and PV complexes. Regardless, our general considerations demonstrate that VCit and PV complexes should form as predicted by the speciation analysis (Pettersson et al., 1983; Ehde et al., 1989; Selling et al., 1994) and reflect the equilibrium mixture observed by NMR analysis.
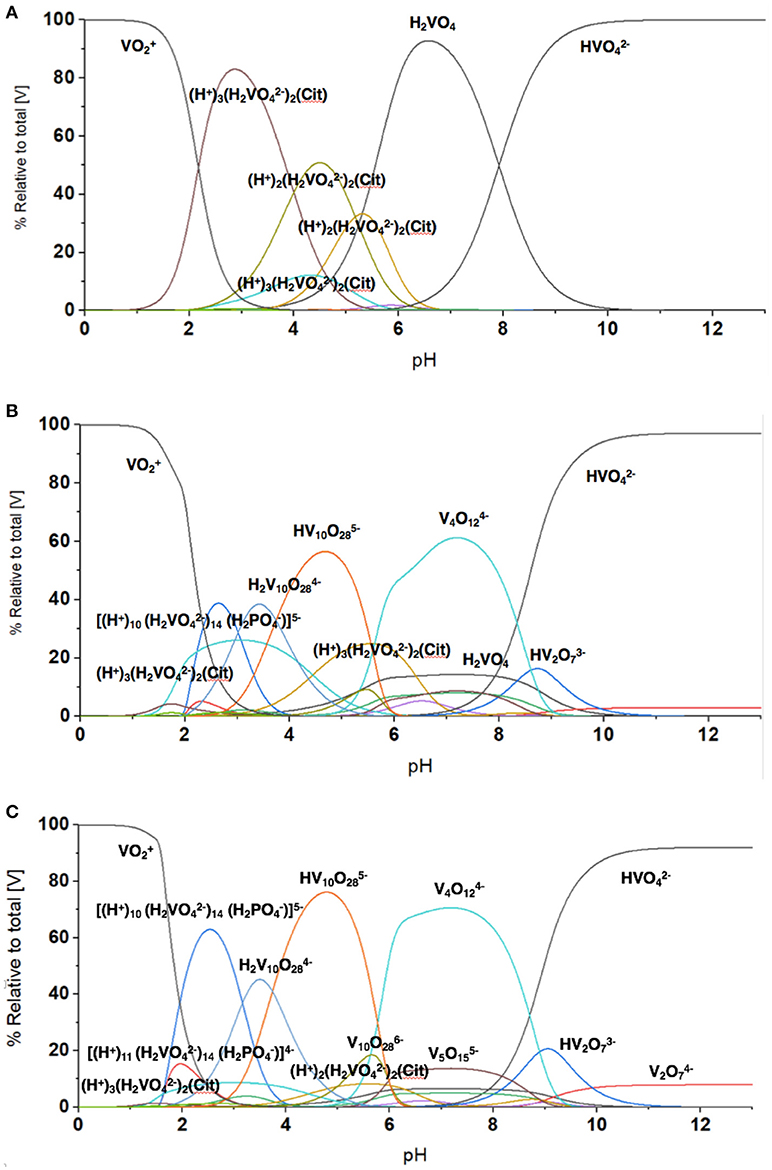
Figure 7. The evaluation of the speciation at different vanadium concentration using HySS Program (vs. 2009) (Alderighi et al., 1999). The speciation diagram shown was calculated at several vanadium (V-atom) concentrations in the presence of 0.48 mM citrate and 24 mM phosphate found in the growth media and (A) 5.0 μM vanadate, (B) 3.3 mM vanadate, and (C) 10 mM vanadate. Note, the concentrations of different species regardless of nuclearity are here shown in terms of V-atoms.
Discussion
Vanadium derivatives have been considered as therapeutic agents since 1899 (Lyonnet et al., 1899; Crans et al., 2018). Recent studies demonstrate that vanadium compounds and salts are effective antidiabetic agents (Sakurai, 2002; Thompson and Orvig, 2006; Kiss et al., 2008; Smith et al., 2008; Thompson et al., 2009; Willsky et al., 2011, 2013; Crans, 2015; Trevino et al., 2015; Boulmier et al., 2017; Levina and Lay, 2017b) but their therapeutic properties have been expanded for these materials to be anticancer agents (Lu and Zhu, 2011; Leon et al., 2017; Medina et al., 2017; Crans et al., 2018), immunotherapy agents (Lu and Zhu, 2011; Selman et al., 2018) as well as treatment for other diseases (Zwolak, 2014; Pessoa et al., 2015). Many of the compounds are coordination complexes, which form vanadate during processing after administration (Thompson et al., 2009; Willsky et al., 2011). Considering the ease with which vanadium undergoes redox chemistry (Crans et al., 2010), it is known that some of the vanadium is converted from V(V) to lower oxidation states upon administration (Thompson et al., 2009; Willsky et al., 2011). Furthermore, studies were carried out on the salts prior to investigation of coordination complexes (Sakurai et al., 1990; Goldfine et al., 2000; Willsky et al., 2001, 2013; Smith et al., 2008). The biological activity of homo-oxovanadates have been of considerable interest considering the varied biological effects depending on the specific vanadium species (Sakurai et al., 1990; Willsky et al., 2001; Postal et al., 2016). Information on cellular uptake by vanadium species is of interest, and the studies presented here compare the effect of monomeric vanadate and decavanadate. Such direct comparison is important to understand the interconversion between these species, and despite the many reports in the literature (reviewed in Aureliano and Crans, 2009), no similar study showing data for direct comparison between the two different species have been reported.
Cellular studies have been reported in different types of organisms that consider the uptake of vanadate. Willsky reported studies with yeast (Willsky et al., 1984b, 1985; Crans et al., 2002), and concluded that V1 was the species entering the cells; however, upon entry processing took place; forming decavanadate in the lysosomes, and forming V(IV) through redox processes. The treatment of the yeast cells with vanadate solutions containing mainly vanadate monomer and oligomers is evidenced by 51V NMR spectroscopy and EPR spectroscopy. These studies were very elegant and truly important, because they documented the formation of decavanadate in cells from monomeric vanadate for the first time (Willsky et al., 1984b). Recently, Zakrzewska and Zivic reported a series of studies in fungi exploring the uptake of both vanadate and V(IV) using 51V and EPR spectroscopy, as well as polarographic studies (Zizic et al., 2013, 2016; Hadzibrahimovic et al., 2017). The initial 51V NMR studies on Phycomyce blakesleeanus mycelium were supplemented with polarography, which allowed for the assignment of the signal at −535 ppm accumulating inside the fungi cells to V1 even though the pH of the system was defined by HEPES buffer at 7.2 where V1 generally has a peak position at a higher frequency (Zizic et al., 2016). The authors attribute the signal shifting to intracellular complexation of V1. Alternatively, these observations are consistent with a hydrophobic environment having been reported in some cases to alter composition and speciation and impact the shifting of observed signals. Combined, these studies showed convincingly that the cell wall is not responsible for reduction of vanadate (Hadzibrahimovic et al., 2017) and these studies also confirm that tetrameric vanadate is not able to enter the cells (Zizic et al., 2016) as reported in yeast as well (Crans et al., 2002) (Willsky et al., 1984a,b.)
Cellular studies in mammalian systems have also been carried out, and many of these studies are linked to the stability and speciation of vanadium compounds in blood (Delgado et al., 2005; Zhang et al., 2006; Li et al., 2009; Jakusch et al., 2011, 2014; Willsky et al., 2013; Sanna et al., 2014, 2017; Jakusch and Kiss, 2017). Although bacterial cells do not contain blood, they do infect mammals and thus the vanadium chemistry in blood is relevant to bacterial infections as well. These studies include detailed investigations into various complexes, and projections based on studies in model systems what would be observed in mammalian systems and in vivo. Although such studies remain model studies, they are important because they begin to provide a view of how it will look like in the in vivo cells. Studies were also reported by Garner and coworkers using 1H NMR spin echo and 51V NMR spectroscopy to characterize the uptake of vanadate and subsequent reduction to form V(IV) in erythrocytes (Garner et al., 1997). The reduction of the vanadate was attributed to glutathione as demonstrated in a time-dependent response using both 51V NMR and 1H spin echo NMR (Garner et al., 1997). The addition of the blocking agent 4,4′-diisothio-cyanatostilbene-2,2b-disulfonic acid (DIDS) specifically blocks the anion transporter preventing the vanadate from entering the erythrocytes. Adding the blocker prevented vanadate entering, and the depletion of glutathione was arrested. Thus, depletion of intracellular gluthathione could be correlated with entering vanadate and the authors proposed that the reduced vanadium [presumably V(IV)] formed complexed with cellular components, because no or little V(IV) formed (Garner et al., 1997).
These studies stand in direct contrast to studies in which decavanadate is associated with proteins and have been characterized by X-ray crystallography (Winkler et al., 2017). However, consideration of both solution and solid state is necessary to characterize both the effects of decavanadate and simple oxovanadates. The studies shown here demonstrate three important findings. First, the fact that decavanadate is a potent inhibitor of growth of two mycobacterial species and more so than monomeric vanadate, which is a known potent phosphatase inhibitor (Crans, 2015; Mclauchlan et al., 2015). Indeed, phosphatase inhibition has recently been reported by V10 of the Leishmania acid phosphatase as well as earlier reports (Aureliano and Crans, 2009; Dorsey et al., 2018). Second, the fact that monomeric vanadate is not as potent inhibitor for M. tb and M. smeg. Third, these studies also demonstrate that under conditions in which the decavanadate would remain stable, in the presence of Mycobacterium spp. the decavanadate undergoes hydrolysis. These combined observations suggest that some type of mechanism that involves the bacterium (or something excreted by the bacterium) interacting with the decavanadate and causes the conversion to oxovanadates. This is particularly interesting because similar response is not observed in the media without bacteria.
Conclusion
The studies presented demonstrate that decavanadate (V10) inhibits the growth of two mycobacterial species, M. tb and M. smeg, whereas the oxovanadates prepared from NaVO3 inhibit growth with 10-100-fold less potency. The inhibition was observed in media containing citrate and phosphate, resulting in the formation of a VCit complex and PV complexes. However, neither of these complexes appeared to interfere with the observed inhibition for V10 and the inhibition by V1 was still 10-100-fold less. The greater inhibitory selectivity of V10 (or a component of V10) result is important because it demonstrates that simple vanadium salts can have different effects on cells, and that a vanadium compound other than the potent phosphatase inhibitor, monomeric vanadate (V1), can inhibit growth. Importantly, these studies suggest that other oxometalates may have the ability to inhibit growth of mycobacteria and other pathogens.
In addition, these studies demonstrated that mycobacteria or some component excreted by the mycobacteria which catalyzes the hydrolysis of V10 and the same effect was not observed in media in the absence of growing cells. This process is particularly interesting because it implies that the V10 interacts with the some cell component, possibly through some mechanism involving V10 delivering a V-atom to such a cell component. This type of mechanism is novel and further investigation into such process will be of general interest and important for the mode of action of POMs in biological systems.
Author Contributions
NS carried out the 51V NMR studies, prepared the figures and wrote part of the manuscript. ZA carried out the growth studies with vanadate and decavanadate and recorded some of the NMR spectra. ZA also collected the experiments with the colors of the various media. HM did the speciation calculations. SK and DCri advised and oversaw the biological studies. DCra combined the chemical and biological components of the work, advised and oversaw the NMR studies and wrote the manuscript with the assistance of NS. All authors contributed to the editing of the manuscript.
Funding
NS was funded by Rajabhat Rajanagarindra University to take a summer leave to carry out research. ZA was funded by a fellowship from Libyan Ministry of Education. Supplies were obtained in part from funds from the Arthur P. Cope Foundation administered by the American Chemical Society (to DCC) and the NSF grant (CHE-1709564) (to DCri and DCra).
Conflict of Interest Statement
The authors declare that the research was conducted in the absence of any commercial or financial relationships that could be construed as a potential conflict of interest.
Acknowledgments
We thank the Core Facilities at Colorado State University for their assistance in carrying out the work described in this manuscript. NS thanks Rajabhat Rajanagarindra University for the support to travel and carry out research.
Supplementary Material
The Supplementary Material for this article can be found online at: https://www.frontiersin.org/articles/10.3389/fchem.2018.00519/full#supplementary-material
More figures are included in the supplementary materials showing additional spectra supporting the results presented in the manuscript and include NMR experiments, growth experiments and additional information on the speciation calculations.
References
Alderighi, L., Gans, P., Ienco, A., Peters, D., Sabatini, A., and Vacca, A. (1999). Hyperquad simulation and speciation (HySS): a utility program for the investigation of equilibria involving soluble and partially soluble species. Coor. Chem. Rev. 184, 311–318. doi: 10.1016/S0010-8545(98)00260-4
Andersson, I., Gorzsas, A., Kerezsi, C., Toth, I., and Pettersson, L. (2005). Speciation in the aqueous H+/H2VO4-H+2O2/phosphate system. Dalton Trans. 22, 3658–3666. doi: 10.1039/b508273k
Auerbach-Nevo, T., Zarivach, R., Peretz, M., and Yonath, A. (2005). Reproducible growth of well diffracting ribosomal crystals. Acta Crystallogr. Sec. D. 61, 713–719. doi: 10.1107/S0907444905006311
Aureliano, M. (2014). Decavanadate contribution to vanadium biochemistry: in vitro and in vivo studies. Inorg. Chim. Acta 420, 4–7. doi: 10.1016/j.ica.2013.10.010
Aureliano, M. (2016). Decavanadate toxicology and pharmacological activities: V-10 or V-1, both or none? Oxid. Med. Cell Longev. 2016:6103457. doi: 10.1155/2016/6103457
Aureliano, M., and Crans, D. C. (2009). Decavanadate (V10) and oxovanadates: oxometalates with many biological activities. J. Inorg. Biochem. 103, 536–546. doi: 10.1016/j.jinorgbio.2008.11.010
Aureliano, M., Fraqueza, G., and Ohlin, C. A. (2013). Ion pumps as biological targets for decavanadate. Dalton Trans. 42, 11770–11777. doi: 10.1039/c3dt50462j
Aureliano, M., and Ohlin, C. A. (2014). Decavanadate in vitro and in vivo effects: facts and opinions. J. Inorg. Biochem. 137, 123–130. doi: 10.1016/j.jinorgbio.2014.05.002
Aureliano, M., Ohlin, C. A., Vieira, M. O., Marques, M. P. M., Casey, W. H., and De Carvalho, L. (2016). Characterization of decavanadate and decaniobate solutions by Raman spectroscopy. Dalton Trans. 45, 7391–7399. doi: 10.1039/C5DT04176G
Baruah, B., Roden, J. M., Sedgwick, M., Correa, N. M., Crans, D. C., and Levinger, N. E. (2006). When is water not water? Exploring water confined in large reverse micelles using a highly charged inorganic molecular probe. J. Am. Chem. Soc. 128, 12758–12765. doi: 10.1021/ja0624319
Bashan, A., and Yonath, A. (2008). The linkage between ribosomal crystallography, metal ions, heteropolytungstates and functional flexibility. J. Mol. Struct. 890, 289–294. doi: 10.1016/j.molstruc.2008.03.043
Bbltm, D. (2018). “Middlebrook 7H9 Broth • Middlebrook 7H9 Broth with Glycerol • Middlebrook 7H9 Broth with Polysorbate 80 • Middlebrook ADC Enrichment.” 2nd ed).
Beresford, N., Mulhearn, D., Szczepankiewicz, B., Liu, G., Johnson, M. E., Fordham-Skelton, A., et al. (2009). Inhibition of MptpB phosphatase from Mycobacterium tuberculosis impairs mycobacterial survival in macrophages. J. Antimicrob. Chemother. 63. 928–936. doi: 10.1093/jac/dkp031
Boulmier, A., Feng, X. X., Oms, O., Mialane, P., Riviere, E., Shin, C. J., et al. (2017). Anticancer activity of Polyoxometalate-Bisphosphonate complexes: synthesis, characterization, in vitro and in vivo results. Inorg. Chem. 56, 7558–7565. doi: 10.1021/acs.inorgchem.7b01114
Carsella, J. S., Sánchez-Lombardo, I.;, Bonetti, S. J., and Crans, D. C. (2017). Selenium speciation in the Fountain Creek Watershed correlates with water hardness, Ca and Mg levels Molecules 22, 708, 1–16. doi: 10.3390/molecules22050708
Chasteen, N. D. (1983). “The biochemistry of vanadium,” in Copper, Molybendum, and Vanadium in Biological Systems, eds M. J. Clarke, J. B. Goodenough, J. A. Ibers, C. K. Jørgensen, D. M. P. Mingos, J. B. Neilands, G. A. Palmer, D. Reinen, P. J. Sadler, R. Weiss, and R.J.P. Williams (New York, NY: Springer-Verlag), 105–138. doi: 10.1007/BFb0111304
Chen, C. Y., Chen, M. L., Chen, H. B., Wang, H. X., Cramer, S. P., and Zhou, Z. H. (2014). alpha-Hydroxy coordination of mononuclear vanadyl citrate, malate and S-citramalate with N-heterocycle ligand, implying a new protonation pathway of iron-vanadium cofactor in nitrogenase. J. Inorg. Biochem. 141, 114–120. doi: 10.1016/j.jinorgbio.2014.08.003
Chen, C. Y., Zhou, Z. H., Mao, S. Y., and Wan, H. L. (2007). Asymmetric dinuclear hydroxyl and ethoxyl citrato dioxovanadates(V). J. Coord. Chem. 60, 1419–1426. doi: 10.1080/00958970601029420
Clare, B. W., Kepert, D. L., and Watts, D. W. (1973a). Acid decompositon of decavanadate-specific salt effects. J. Chem. Soc., Dalton Trans. 22, 2481–2487.
Clare, B. W., Kepert, D. L., and Watts, D. W. (1973b). Kinetic study of the acid decomposition of decavanadate. J. Chem. Soc. Dalton Trans. 22, 2479–2487. doi: 10.1039/dt9730002479
Comba, P., and Helm, L. (1988). The solution structure and reactivity of decavanadate. Helv. Chim. Acta 71, 1406–1420. doi: 10.1002/hlca.19880710605
Correia, I., Adão, P., Roy, S., Wahba, M. C M, Maurya, M. R., Marques, F., et al. (2014). Hydroxyquinoline derived vanadium(IV and V) and copper(II) complexes as potential anti-tuberculosis and anti-tumor agents. J. Inorg. Biochem. 141, 83–93. doi: 10.1016/j.jinorgbio.2014.07.019
Correia, I., Roy, S., Matos, C. P., Borovic, S., Butenko, N., Cavaco, I., et al. (2015). Vanadium(IV) and copper(II) complexes of salicylaldimines and aromatic heterocycles: Cytotoxicity: DNA binding and DNA cleavage properties. J. Inorg. Biochem. 147, 134–146. doi: 10.1016/j.jinorgbio.2015.02.021
Costello, R. L., and Hedgecock, L. W. (1959). Effect of metavanadate ion on the growth in vitro of mycobacterium tuberculosis. J. Bacteriol. 77, 794–799.
Crans, D., Yang, L., Haase, A., and Yang, X. (2018). Health benefits of Vanadium and its potential as an anticancer agent. Met. Ions Life Sci. 18, 251–279. doi: 10.1515/9783110470734-015
Crans, D. C. (2000). Chemistry and insulin-like properties of vanadium(IV) and vanadium(V) compounds. J. Inorg. Biochem. 80, 123–131. doi: 10.1016/S0162-0134(00)00048-9
Crans, D. C. (2005). Fifteen years of dancing with vanadium. Pure Appl. Chem. 77, 1497–1527. doi: 10.1351/pac200577091497
Crans, D. C. (2015). Antidiabetic, chemical, and physical properties of organic Vanadates as Presumed transition-state inhibitors for Phosphatases. J. Org. Chem. 80, 11899–11915. doi: 10.1021/acs.joc.5b02229
Crans, D. C., Bedi, H. S., Li, S., Zhang, B., Nomiya, K., Kasuga, N. C., Willsky, G.R., et al. (2002). “Tetravanadate, decavanadate, Keggin and Dawson oxotungstates inhibit growth of S. cerevisiae” in Polyoxometalate Chemistry for Nano-Composite Design, [Symposium] (Honolulu, HI), 181–195.
Crans, D. C., Peters, B. J., Wu, W., and Mclauchlan, C. C. (2017). Does Anion-Cation Organization in Na+-containing X-ray crystal structures relate to solution interactions in inhomogeneous nanoscale environments: Sodium-decavanadate in solid state materials, minerals and microemulsions. Coord. Chem. Rev. 344, 115–130. doi: 10.1016/j.ccr.2017.03.016
Crans, D. C., Rithner, C. D., Baruah, B., Gourley, B. L., and Levinger, N. E. (2006). Molecular probe location in reverse micelles determined by NMR dipolar interactions. J. Am. Chem. Soc. 128, 4437–4445. doi: 10.1021/ja0583721
Crans, D. C., Rithner, C. D., and Theisen, L. A. (1990). Application of time-resolved 51V 2D NMR for quantitation of kinetic exchange pathways between vanadate monomer, dimer, tetramer, and pentamer. J. Am. Chem. Soc. 112, 2901–2908. doi: 10.1021/ja00164a009
Crans, D. C., Smee, J. J., Gaidamauskas, E., and Yang, L. Q. (2004). The chemistry and biochemistry of vanadium and the biological activities exerted by vanadium compounds. Chem. Rev. 104, 849–902. doi: 10.1021/cr020607t
Crans, D. C., Trujillo, A. M., Pharazyn, P. S., and Cohen, M. D. (2011). How environment affects drug activity: localization, compartmentalization and reactions of a vanadium insulin-enhancing compound, dipicolinatooxovanadium(V). Coord. Chem. Rev. 255, 2178–2192. doi: 10.1016/j.ccr.2011.01.032
Crans, D. C., Woll, K. A., Prusinskas, K., Johnson, M. D., and Norkus, E. (2013). Metal speciation in health and medicine represented by iron and vanadium. Inorg. Chem. 52, 12264–12275. doi: 10.1021/ic4007873
Crans, D. C., Yang, L. Q., Jakusch, T., and Kiss, T. (2000). Aqueous chemistry of ammonium (dipicolinato)oxovanadate(V): the first organic vanadium(V) insulin-mimetic compound. Inor. Chem. 39, 4409–4416. doi: 10.1021/ic9908367
Crans, D. C., Zhang, B., Gaidamauskas, E., Keramidas, A. D., Willsky, G. R., and Roberts, C. R. (2010). Is Vanadate reduced by thiols under biological conditions? Changing the redox potential of V(V)/V(IV) by complexation in aqueous solution. Inor. Chem. 49, 4245–4256. doi: 10.1021/ic100080k
David, S., Barrosa, V., Cruzb, C., and Delgado, R. (2005). In vitro effect of free and complexed indium(III) against Mycobacterium tuberculosis. FEMS Microbiol. Lett. 251, 119–124. doi: 10.1016/j.femsle.2005.07.044
Delgado, T. C., Tomaz, A. I., Correia, I., Pessoa, J. C., Jones, J. G., Geraldes, C. F., et al. (2005). Uptake and metabolic effects of insulin mimetic oxovanadium compounds in human erythrocytes. J. Inorg. Biochem., 99, 2328–2339. doi: 10.1016/j.jinorgbio.2005.08.014
Dorsey, B. M., McLauchlan, C. C., and Jones, M. A. (2018). Evidence that speciation of oxovanadium complexes does not solely account for inhibition of Leishmania Acid Phosphatases. Front. Chem. 8:109. doi: 10.3389/fchem.2018.00109
Druskovich, D. M., and Kepert, D. L. (1975). Base decomposition of decavanadate. J. Chem. Soc. Dalton Trans. 22, 947–851. doi: 10.1039/dt9750000947
Dutta, N. K., He, R., Pinn, M. L., He, Y., Burrows, F., Zhang, Z.-Y., et al. (2016). Mycobacterial protein tyrosine phosphatase A and B inhibitors augment the bactericidal activity of the standard anti-tuberculosis regimen. ACS Infect. Dis. 2, 231–239. doi: 10.1021/acsinfecdis.5b00133
Ehde, P., Andersson, I., and Pettersson, L. (1989). Multicomponent polyanions. 43. A study of aqueous equilibria in the vanadocitrate system. Acta Chem. Scand. 43, 136–143. doi: 10.3891/acta.chem.scand.43-0136
Esbak, H., Enyedy, E. A., Kiss, T., Yoshikawa, Y., Sakurai, H., Garribba, E., et al. (2009). Aminoacid-derivatised picolinato-oxidovanadium(IV) complexes: characterisation, speciation and ex vivo insulin-mimetic potential. J. Inorg. Biochem. 103, 590–600. doi: 10.1016/j.jinorgbio.2008.11.001
Evans, H. T. Jr. (1966). The molecular structure of the isopoly complex ion, decavanadate (V10). Inorg. Chem. 5, 967–977. doi: 10.1021/ic50040a004
Fraqueza, G., De Carvalho, L., Marques, M. P. M., Maia, L., Ohlin, C. A., Casey, W. H., et al. (2012). Decavanadate, decaniobate, tungstate and molybdate interactions with sarcoplasmic reticulum Ca2+-ATPase: quercetin prevents cysteine oxidation by vanadate but does not reverse ATPase inhibition. Dalton Trans. 41, 12749–12758. doi: 10.1039/c2dt31688a
Fu, D. Y., Zhang, S. M., Qu, Z. Y., Yu, X. H., Wu, Y. Q., and Wu, L. X. (2018). Hybrid assembly toward enhanced thermal stability of virus-like particles and antibacterial activity of Polyoxometalates. ACS Appl. Mater. Interf. 10, 6137–6145. doi: 10.1021/acsami.7b17082
Gajera, S. B., Mehta, J. I., and Patel, M. N. (2015). DNA interaction, cytotoxicity, antibacterial and antituberculosis activity of oxovanadium(IV) complexes derived from ?uoroquinolones and 4-hydroxy-5-((4-hydroxyphenyl)diazenyl)thiazole-2(3H)-thione †. RSC Adv. 5, 21710–21719. doi: 10.1039/C5RA01222H
Garner, M., Reglinski, J., Smith, W. E., Mcmurray, J., Abdullah, I., and Wilson, R. (1997). A 1H spin echo and 51V NMR study of the interaction of vanadate with intact erythrocytes. J. Biol. Inorg. Chem. 2, 235–241. doi: 10.1007/s007750050129
Goldfine, A. B., Patti, M.-E., Zuberi, L., Goldstein, B. J., Leblanc, R., Landaker, E. J., et al. (2000). Metabolic effects of vanadyl sulfate in humans with non—insulin-dependent diabetes mellitus: in vivo and in vitro studies. Metabolism 49, 400–410. doi: 10.1016/S0026-0495(00)90418-9
Gresser, M. J., Tracey, A. S., and Parkinson, K. M. (1986). Vanadium(V) oxyanions: the interaction of vanadate with pyrophosphate, phosphate, and arsenate. J. Am. Chem. Soc. 108, 6229–6234. doi: 10.1021/ja00280a020
Gumerova, N., Krivosudsky, L., Fraqueza, G., Breibeck, J., Al-Sayed, E., Tanuhadi, E., et al. (2018). The P-type ATPase inhibiting potential of polyoxotungstates. Metallomics 10, 287–295. doi: 10.1039/C7MT00279C
Hadzibrahimovic, M., Suznjevic, D., Pastor, F., Antic, T. C., Zizic, M., Zakrzewska, J., et al. (2017). The interactions of vanadate monomer with the mycelium of fungus Phycomyces blakesleeanus: reduction or uptake? Anton. V. Leeuw. Inter. J. Gen. Mol. Microb. 110, 365–373. doi: 10.1007/s10482-016-0808-0
Hider, R. C., and Kong, X. (2010). Chemistry and biology of siderophores. Nat. Prod. Rep. 27, 637–657. doi: 10.1039/b906679a
Hill, C. L., Hartnup, M., Faraj, M., Weeks, M., Prosser-Mccartha, C. M., Brown, R. B. Jr., et al. (1990). Polyoxometalates as inorganic anti-HIV-1 compounds. Structure-activity relationships. Adv. Chemotherapy AIDS, 33–41.
Jakusch, T., Enyedy, É. A., Kozma, K., Paár, Z., Bényei, A., and Kiss, T. (2014). Vanadate complexes of 3-hydroxy-1, 2-dimethyl-pyridinone: Speciation, structure and redox properties. Inorg. Chim. Acta 420, 92–102. doi: 10.1016/j.ica.2013.12.034
Jakusch, T., and Kiss, T. (2017). In vitro study of the antidiabetic behavior of vanadium compounds. Coord. Chem. Rev. 351, 118–126. doi: 10.1016/j.ccr.2017.04.007
Jakusch, T., Pessoa, J. C., and Kiss, T. (2011). The speciation of vanadium in human serum. Coord. Chem. Rev. 255, 2218–2226. doi: 10.1016/j.ccr.2011.02.022
Kaliva, M., Kyriakakis, E., and Salifoglou, A. (2002). Reactivity investigation of dinuclear vanadium(IV,V)-citrate complexes in aqueous solutions. A closer look into aqueous vanadium-citrate interconversions. Inorg. Chem. 41, 7015–7023. doi: 10.1021/ic020323r
Kaliva, M., Raptopoulou, C. P., Terzis, A., and Safifoglou, A. (2003). Systematic studies on pH-dependent transformations of dinuclear vanadium(V)-citrate complexes in aqueous solutions - A perspective relevance to aqueous vanadium(V)-citrate speciation. J. Inorg. Biochem. 93, 161–173. doi: 10.1016/S0162-0134(02)00563-9
Kioseoglou, E., Gabriel, C., Petanidis, S., Psycharis, V., Raptopoulou, C. P., Terzis, A., et al. (2013). Binary Decavanadate-Betaine composite materials of potential anticarcinogenic activity. Z. Anorg. Allg. Chem. 639, 1407–1416. doi: 10.1002/zaac.201300144
Kioseoglou, E., Petanidis, S., Gabriel, C., and Salifoglou, A. (2015). The chemistry and biology of vanadium compounds in cancer therapeutics. Coord. Chem. Rev. 301, 87–105. doi: 10.1016/j.ccr.2015.03.010
Kiss, T., Jakusch, T., Hollender, D., Dornyei, A., Enyedy, E. A., Pessoa, J. C., et al. (2008). Biospeciation of antidiabetic VO(IV) complexes. Coord. Chem. Rev. 252, 1153–1162. doi: 10.1016/j.ccr.2007.09.011
Kustin, K. (2015). Aqueous vanadium ion dynamics relevant to bioinorganic chemistry: a review. J. Inorg. Biochem. 147, 32–38. doi: 10.1016/j.jinorgbio.2014.12.009
Le, M., Rathje, O., Levina, A., and Lay, P. A. (2017). High cytotoxicity of vanadium (IV) complexes with 1, 10-phenanthroline and related ligands is due to decomposition in cell culture medium. J. Biol. Inorg. Chem. 22, 663–672. doi: 10.1007/s00775-017-1453-4
Leon, I. E., Cadavid-Vargas, J. F., Di Virgilio, A. L., and Etcheverry, S. B. (2017). Vanadium, Ruthenium and Copper compounds: a new class of Nonplatinum Metallodrugs with Anticancer activity. Curr. Med. Chem. 24, 112–148. doi: 10.2174/0929867323666160824162546
Leon, I. E., Porro, V., Astrada, S., Egusquiza, M. G., Cabello, C. I., Bollati-Fogolin, M., et al. (2014). Polyoxometalates as antitumor agents: bioactivity of a new polyoxometalate with copper on a human osteosarcoma model. Chem. Biol. Interact. 222, 87–96. doi: 10.1016/j.cbi.2014.10.012
Levina, A., Crans, D. C., and Lay, P. (2017a). Speciation of metal drugs, supplements and toxins in media and bodily fluids controls in vitro activities. Coord. Chem. Rev. 352, 473–498. doi: 10.1016/j.ccr.2017.01.002
Levina, A., and Lay, P. A. (2017b). Stabilities and Biological Activities of Vanadium Drugs: What is the Nature of the Active Species?. Chem.Asian J. 12, 1692–1699. doi: 10.1002/asia.201700463
Li, M., Ding, W., Smee, J. J., Baruah, B., Willsky, G. R., and Crans, D. C. (2009). Anti-diabetic effects of vanadium (III, IV, V) - chlorodipicolinate complexes in streptozotocin-induced diabetic rats, BioMetals 103, 585–905. doi: 10.1007/s10534-009-9241-4
Lodyga-Chruscinska, E., Sanna, D., Garribba, E., and Micera, G. (2008). Potentiometric, spectroscopic, electrochemical and DFT characterization of oxovanadium(IV) complexes formed by citrate and tartrates in aqueous solution at high ligand to metal molar ratios: the effects of the trigonal bipyramidal distortion in bis-chelated species and biological implications. Dalton Trans. 28, 4903–4916. doi: 10.1039/b803520b
Lu, L. P., and Zhu, M. L. (2011). Metal-based inhibitors of protein Tyrosine Phosphatases. Anti Cancer Agents Med. Chem. 11, 164–171. doi: 10.2174/187152011794941271
Lyonnet, B., Martz, S., and Martin, E. (1899). L'emploi therapeutique des derives du vanadium. La Presse Méd. 1, 191–192.
Maiti, A., and Ghosh, S. (1989). Synthesis and reactivity of the Oxovanadium(IV) Complexes of Two N-O donors and potentiation of the antituberculosis activity of one of them on Chelation to metal ions: part, I. V. J. Inorg. Biochem. 36, 131–139. doi: 10.1016/0162-0134(89)80020-0
Martins, P. G., Mori, M., Chiaradia-Delatorre, L. D., Menegatti, A. C., Mascarello, A., Botta, B., et al. (2015). Exploring oxidovanadium (IV) complexes as YopH inhibitors: mechanism of action and modeling studies. ACS Med. Chem. Lett. 6, 1035–1040. doi: 10.1021/acsmedchemlett.5b00267
Mclauchlan, C. C., Peters, B. J., Willsky, G. R., and Crans, D. C. (2015). Vanadium-phosphatase complexes: Phosphatase inhibitors favor the trignonal bipyramidal transition state geometries. Coord. Chem. Rev. 301–302, 163–199. doi: 10.1016/j.ccr.2014.12.012
Medina, J. J. M., Naso, L. G., Pérez, A. L., Rizzi, A., Ferrer, E. G., and Williams, P. A. (2017). Antioxidant and anticancer effects and bioavailability studies of the flavonoid baicalin and its oxidovanadium (IV) complex. J. Inorg. Biochem. 166, 150–161. doi: 10.1016/j.jinorgbio.2016.11.005
Moskovitz, B. L., and Group, A. T. H.-C. S. (1988). Clinical trial and tolerance of HPA-23 in patients with acquired immunodeficiency syndrome. Antimicrob. Agents Chemother. 32, 1300–1303. doi: 10.1128/AAC.32.9.1300
Noeske, J., Wasserman, M. R., Terry, D. S., Altman, R. B., Blanchard, S. C., and Cate, J. H. D. (2015). High-resolution structure of the Escherichia coli ribosome. Nat. Struct. Mol. Biol. 22, 336–341. doi: 10.1038/nsmb.2994
Pessoa, J. C., Etcheverry, S., and Gambino, D. (2015). Vanadium compounds in medicine. Coord. Chem. Rev. 301, 24–48. doi: 10.1016/j.ccr.2014.12.002
Pessoa, J. C., and Tomaz, I. (2010). Transport of therapeutic Vanadium and Ruthenium complexes by blood plasma components. Curr. Med. Chem. 17, 3701–3738. doi: 10.2174/092986710793213742
Pettersson, L., Andersson, I., and Hedman, B. (1985). Multicomponent polyanions. 37. A potentiometric and 51V-NMR study of equilibria in the H+-H system in 3.0 M-Na(ClO4) medium covering the range 1£-1g[H+]£10. Chem. Scr. 25, 309–317.
Pettersson, L., Hedman, B., Andersson, I., and Ingri, N. (1983). Multicomponent polyanions. 34. P potentiometric and 51V NMR study of equilibria in the H+-HVO42- system in the 0.6 M Na(Cl) medium covering the range 1£-1g[H+]£10. Chem. Scrip. 22, 254–264.
Pluskey, S., Mahroof-Tahir, M., Crans, D. C., and Lawrence, D. S. (1996). Vanadium oxoanions and cAMP-dependent protein kinase: an anti-substrate inhibitor. Biochem. J. 321, 333–339. doi: 10.1042/bj3210333
Pope, M. T., and Müller, A. (1991). Polyoxometalate chemistry: an old field with new dimensions in several disciplines. Angew. Chem. Int. Ed. Engl. 30, 34–48. doi: 10.1002/anie.199100341
Postal, K., Maluf, D. F., Valdameri, G., Rüdiger, A. L., Hughes, D. L., de Sá, E. L., et al. (2016). Chemoprotective activity of mixed valence polyoxovanadates against diethylsulphate in E. coli cultures: insights from solution speciation studies. RSC Adv. 6, 114955–114866. doi: 10.1039/c6ra15826a
Rehder, D. (1991). The bioinorganic chemistry of vanadium. Angew. Chem. Int. Ed. Engl. T 30, 148–167. doi: 10.1002/anie.199101481
Rehder, D., Pessoa, J. C., Geraldes, C., Castro, M., Kabanos, T., Kiss, T., et al. (2002). In vitro study of the insulin-mimetic behaviour of vanadium(IV, V) coordination compounds. J. Biol. Inorg. Chem. 7, 384–396. doi: 10.1007/s00775-001-0311-5
Rhule, J. T., Hill, C. L., Judd, D. A., and Schinazi, R. F. (1998). Polyoxometalates in medicine. Chem. Rev. 98, 327–358. doi: 10.1021/cr960396q
Sakurai, H. (2002). A new concept: the use of vanadium complexes in the treatment of diabetes mellitus. Chem. Rec. 2, 237–248. doi: 10.1002/tcr.10029
Sakurai, H., Tsuchiya, K., Nukatsuka, M., Kawada, J., Ishikawa, S., Yoshida, H., et al. (1990). Insulin-mimetic action of vanadyl complexes. J. Clin. Biochem. Nutr. 8, 193–200. doi: 10.3164/jcbn.8.193
Samart, N., Saeger, J., Haller, K., Manuel Aureliano, M., and Crans, D. (2014). Interaction of decavanadate with interfaces and biological model membrane systems: characterization of soft oxometalate systems. J. Mol. Eng. Mat. 2, 1–21. doi: 10.1142/S2251237314400073
Sambandamurthy, V. K., Derrick, S. C., Hsu, T., Chen, B., Larsen, M. H., Jalapathy, K. V., et al. (2006). Mycobacterium tuberculosis ΔRD1 ΔpanCD: a safe and limited replicating mutant strain that protects immunocompetent and immunocompromised mice against experimental tuberculosis. Vaccine 24, 6309–6320. doi: 10.1016/j.vaccine.2006.05.097
Sanchez-Lombardo, I., Baruah, B., Alvarez, S., Werst, K. R., Segaline, N. A., Levinger, N. E., et al. (2016). Size and shape trump charge in interactions of oxovanadates with self-assembled interfaces: application of continuous shape measure analysis to the decavanadate anion. New J. Chem. 40, 962–975. doi: 10.1039/C5NJ01788B
Sanna, D., Serra, M., Micera, G., and Garribba, E. (2014). Interaction of antidiabetic vanadium compounds with hemoglobin and red blood cells and their distribution between plasma and erythrocytes. Inorg. Chem. 53, 1449–1464. doi: 10.1021/ic402366x
Sanna, D., Ugone, V., Micera, G., Bugly,ó, P., Bír,ó, L., and Garribba, E. (2017). Speciation in human blood of Metvan, a vanadium based potential anti-tumor drug. Dalton Trans. 46, 8950–8967. doi: 10.1039/C7DT00943G
Selling, A., Andersson, I., Pettersson, L., and Schramm, C. M., S.L., D., and J.H., G (1994). Multicomponent Polyanions. 47. The aqueous Vanadophosphate system. J. Inorg. Chem. 33, 3141–3150. doi: 10.1021/ic00092a021
Selman, M., Rousso, C., Bergeron, A., Son, H., Krishnan, R., El-Sayes, N., et al. (2018). Multi-modal potentiation of oncolytic virotherapy by Vanadium compounds. Mol. Ther. 26, 56–69. doi: 10.1016/j.ymthe.2017.10.014
Shah, H. S., Joshi, S. A., Haider, A., Kortz, U., Ur-Rehman, N., and Iqbal, J. (2015). Synthesis of chitosan-coated polyoxometalate nanoparticles against cancer and its metastasis. RSC Adv. 5, 93234–93242. doi: 10.1039/C5RA18489D
Smith, D. M., Pickering, R. M., and Lewith, G. T. (2008). A systematic review of vanadium oral supplements for glycaemic control in type 2 diabetes mellitus. Qjm-an Inter. J. Med. 101, 351–358. doi: 10.1093/qjmed/hcn003
Sun, T. D., Cui, W., Yan, M., Qin, G., Guo, W., Gu, H. X., et al. (2016). Target delivery of a novel Antitumor Organoplatinum(IV)-substituted Polyoxometalate Complex for Safer and more effective colorectal cancer therapy in vivo. Adv. Mater. 28, 7397–7404. doi: 10.1002/adma.201601778
Thompson, K. H., Lichter, J., Lebel, C., Scaife, M. C., Mcneill, J. H., and Orvig, C. (2009). Vanadium treatment of type 2 diabetes: a view to the future. J. Inorg. Biochem. 103, 554–558. doi: 10.1016/j.jinorgbio.2008.12.003
Thompson, K. H., and Orvig, C. (2006). Vanadium in diabetes: 100 years from Phase 0 to Phase, I. J. Inorg. Biochem. 100, 1925–1935. doi: 10.1016/j.jinorgbio.2006.08.016
Trevino, S., Sánchez-Lara, E., Sarmiento-Ortega, V. E., Sánchez-Lombardo, I., Flores-Hernández, J. A., Pérez-Benítez, A., et al. (2015). Hypoglycemic, lipid-lowering and metabolic regulation activities of metforminium decavanadate (H2Metf)3 [V10O28]·8H2O using hypercaloric-induced carbohydrate and lipid deregulation in Wistar rats as biological model. J. Inor. Biochem. 147, 85–92. doi: 10.1016/j.jinorgbio.2015.04.002
Turian, G. (1951). Action plasmogene du fer chez les Mycobacteries. Le bacille de la fleole, indicateur biologique du fer. 34,917-920. Helv. Chim. Acta 34, 917–920. doi: 10.1002/hlca.19510340325
Turner, T. L., Nguyen, V. H., Mclauchlan, C. C., Dymon, Z., Dorsey, B. M., Hooker, J. D., et al. (2012). Inhibitory effects of decavanadate on several enzymes and Leishmania tarentolae in vitro. J. Inorg. Biochem. 108, 96–104. doi: 10.1016/j.jinorgbio.2011.09.009
Upadhyay, A., Fontes, F., Gonzalez-Juarrero, M., McNeil, M. R., Crans, D. C., Jackson, M., et al. (2015). MenI, a novel menaquinone reductase in Mycobacterium tuberculosis is required for survival in macrophages. ACS Central Sci. 2015, 1, 292–302. doi: 10.1021/acscentsci.5b00212
Vilas Boas, L. V., and Costa Pessoa, J. (1987). “Vanadium,” in Comprehensive Coordination Chemistry. The Synthesis, Reactions, Properties & Applications of Coordination Compounds, eds G. Wilkinson, Sir, R. D. Gillard, and J. A. Mccleverty (New York, NY: Pergamon Press), 453–583.
Wang, L., Zhou, B. B., and Liu, J. R. (2013). Anticancer Polyoxometalates. Prog. Chem. 25, 1131–1141.
Weinstein, S., Jahn, W., Glotz, C., Schlünzen, F., Levin, I., Janell, D., Yonath, A., et al. and Yonath, A. (1999). Metal compounds as tools for the construction and the interpretation of medium-resolution maps of ribosomal particles. J. Struct. Biol. 127, 141–151. doi: 10.1006/jsbi.1999.4135
Willsky, G. R., Chi, L.-H., Godzala, M. III, Kostyniak, P. J., Smee, J. J., Trujillo, A. M., et al. (2011). Anti-diabetic effects of a series of vanadium dipicolinate complexes in rats with streptozotocin-induced diabetes. Coord. Chem. Rev. 255, 2258–2269. doi: 10.1016/j.ccr.2011.06.015
Willsky, G. R., Goldfine, A. B., Kostyniak, P. J., Mcneill, J. H., Yang, L. Q., Khan, H. R., et al. (2001). Effect of vanadium(IV) compounds in the treatment of diabetes: in vivo and in vitro studies with vanadyl sulfate and bis(maltolato)oxovandium(IV). J. Inorg. Biochem. 85, 33–42. doi: 10.1016/S0162-0134(00)00226-9
Willsky, G. R., Halvorsen, K., Godzala, M. E., Iii, C., hi, L.-H., Most, M. J., Kaszynski, P., et al. (2013). Coordination chemistry may explain pharmacokinetics and clinical response of vanadyl sulfate in type 2 diabetic patients. Metallomics 5, 1491–1502. doi: 10.1039/c3mt00162h
Willsky, G. R., Leung, J. O., Offermann, P. V. Jr., Plotnick, E. K., and Dosch, S. F. (1985). Isolation and characterization of vanadate-resistant mutants of Saccharomyces cerevisiae. J. Bacteriol. 164, 611–617.
Willsky, G. R., Preischel, D. A., and Mccabe, B. C. (1984a). Vanadium metabolism in S-Cerevisiae. Biophys. J. 45, A76–A76.
Willsky, G. R., White, D. A., and Mccabe, B. C. (1984b). Metabolism of added Ortho-Vanadate to Vanadyl and High- molecular-weight Vanadates by Saccharomyces-Cerevisiae. J. Biol. Chem. 259, 3273–3281.
Winkler, P. A., Huang, Y., Sun, W., Du, J., and L.ü, W. (2017). Electron cryo-microscopy structure of a human TRPM4 channel. Nature 552, 200–204. doi: 10.1038/nature24674
Zhai, F. Y., Wang, X. H., Li, D. L., Zhang, H., Li, R., and Song, L. S. (2009). Synthesis and biological evaluation of decavanadate Na(4)Co(H(2)O)(6)V(10)O(28)center dot 18H(2)O. Biomed. Pharmacother. 63, 51–55. doi: 10.1016/j.biopha.2008.01.006
Zhang, Y., Yang, X. D., Wang, K., and Crans, D. C. (2006), The permeability and cytotoxicity of insulin-mimetic vanadium (III,IV,V)-dipicolinate complexes, J. Inorg. Biochem. 100, 80–87. doi: 10.1016/j.jinorgbio.2005.10.006
Zhou, Z. H., Zhang, H., Jiang, Y. Q., Lin, D. H., Wan, H. L., and Tsai, K. R. (1999). Complexation between vanadium(V) and citrate: spectroscopic and structural characterization of a dinuclear vanadium(V) complex. Transit. Metal Chem. 24, 605–609. doi: 10.1023/A:1006947218366
Zizic, M., Miladinovic, Z., Stanic, M., Hadzibrahimovic, M., Zivic, M., and Zakrzewska, J. (2016). V-51 NMR investigation of cell-associated vanadate species in Phycomyces blakesleeanus mycelium. Res. Microbiol. 167, 521–528. doi: 10.1016/j.resmic.2016.04.012
Zizic, M., Zivic, M., Spasojevic, I., Bogdanovic Pristov, J., Stanic, M., Cvetic-Antic, T., et al. (2013). The interactions of vanadium with Phycomyces blakesleeanus mycelium: enzymatic reduction, transport and metabolic effects. Res. Microbiol. 164, 61–69. doi: 10.1016/j.resmic.2012.08.007
Keywords: vanadate, speciation, sodium metavanadate, decavanadate, growth inhibition, tuberculosis
Citation: Samart N, Arhouma Z, Kumar S, Murakami HA, Crick DC and Crans DC (2018) Decavanadate Inhibits Mycobacterial Growth More Potently Than Other Oxovanadates. Front. Chem. 6:519. doi: 10.3389/fchem.2018.00519
Received: 24 June 2018; Accepted: 09 October 2018;
Published: 20 November 2018.
Edited by:
Christian Hartinger, University of Auckland, New ZealandReviewed by:
Eugenio Garribba, University of Sassari, ItalySanja Grguric-Sipka, University of Belgrade, Serbia
Copyright © 2018 Samart, Arhouma, Kumar, Murakami, Crick and Crans. This is an open-access article distributed under the terms of the Creative Commons Attribution License (CC BY). The use, distribution or reproduction in other forums is permitted, provided the original author(s) and the copyright owner(s) are credited and that the original publication in this journal is cited, in accordance with accepted academic practice. No use, distribution or reproduction is permitted which does not comply with these terms.
*Correspondence: Debbie C. Crans, ZGViYmllLmNyYW5zQGNvbG9zdGF0ZS5lZHU=