- 1Laboratório de Ciências Químicas, Universidade Estadual do Norte Fluminense Darcy Ribeiro, Campos dos Goytacazes, Brazil
- 2Anorganisch-Chemisches Institut and Interdisciplinary Center of Scientific Computing, Universität Heidelberg, Heidelberg, Germany
- 3School of Chemistry and Molecular Biosciences, The University of Queensland, Brisbane, QLD, Australia
A dinickel(II) complex of the ligand 1,3-bis(bis(pyridin-2-ylmethyl)amino)propan-2-ol (HL1) has been prepared and characterized to generate a functional model for nickel(II) phosphoesterase enzymes. The complex, [Ni2(L1)(μ-OAc)(H2O)2](ClO4)2·H2O, was characterized by microanalysis, X-ray crystallography, UV-visible, and IR absorption spectroscopy and solid state magnetic susceptibility measurements. Susceptibility studies show that the complex is antiferromagnetically coupled with the best fit parameters J = −27.4 cm−1, g = 2.29, D = 28.4 cm−1, comparable to corresponding values measured for the analogous dicobalt(II) complex [Co2(L1)(μ-OAc)](ClO4)2·0.5 H2O (J = −14.9 cm−1 and g = 2.16). Catalytic measurements with the diNi(II) complex using the substrate bis(2,4-dinitrophenyl)phosphate (BDNPP) demonstrated activity toward hydrolysis of the phosphoester substrate with Km ~10 mM, and kcat ~0.025 s−1. The combination of structural and catalytic studies suggests that the likely mechanism involves a nucleophilic attack on the substrate by a terminal nucleophilic hydroxido moiety.
Introduction
Our understanding of the bioinorganic significance of nickel can be traced to the discovery that the specific activity of the soluble jack bean urease, after partial EDTA-promoted inactivation, was a linear function of the nickel content, consistent with the presence of two nickel(II) ions per subunit of the pure enzyme (Dixon et al., 1980; Blakeley et al., 1982; Blakeley and Zerner, 1984). Previous to this discovery the importance of metal ions in general for the activity of urease was known, although the specific requirement for Ni(II) ions was not (Jacoby, 1933; Shaw, 1954; Shaw and Raval, 1961; Spears et al., 1977). Subsequently it was recognized that nickel is also required for the enzymatic activity of carbon monoxide dehydrogenase, (Ensign et al., 1989, 1990; Shin and Lindahl, 1992; Gencic and Grahame, 2003) and plays an important role in other bioinorganic systems, (Ashwini, 2006) including [NiFe]-hydrogenase (Przybyla et al., 1992; Sargent, 2016; Vaissier and Van, 2017) and a nickel dependent superoxide dismutase (Barondeau et al., 2004). In contrast to other bioinorganic systems Ni(II) complexes have received less attention. A limited number of studies have focussed on di-Ni(II) model complexes for urease (Meyer, 2009) and FeNi complexes for hydrogenases (Vaissier and Van, 2017), and Neves and colleagues have developed several Ni(II) models for phosphatases, in particular purple acid phosphatases (PAPs) (Greatti et al., 2008; Piovezan et al., 2012; Xavier and Neves, 2016). PAPs present an ideal system to study biomimetics, in parts because of the wealth of sequence (Flanagan et al., 2006), structural and functional data available (Schenk et al., 2013), but also because these enzymes occur in homo- and hetero-bimetallic form in nature, using a range of metal ions (Fe, Zn, Mn; Mitić et al., 2009, 2010). Furthermore, the Fe(III)Ni(II) form of PAP is catalytically active, one of the few known metallohydrolases that can accommodate Ni(II) and maintain functionality (Schenk et al., 2008). This flexibility of PAPs with respect to their use of metal ions may be a reflection of their dual function as a phosphatase and peroxidase; indeed, in its di-Fe(III) form PAP is easily and reversibly reduced to the heterovalent Fe(III)Fe(II) form (redox potential ~340 mV), a process that allows the enzyme to act as a Fenton catalyst (Sibille et al., 1987; Bernhardt et al., 2004).
The metal ion composition of PAP may also influence its reaction mechanism; in particular, the identity of the hydrolysis-initiating nucleophile may be affected by the identity of the metal ions (Mitić et al., 2010; Selleck et al., 2017). Here, we selected ligand 1,3-bis(bis(pyridin-2-ylmethyl)amino)propan-2-ol (Figure 1) to probe the possibility that a di-Ni(II) biomimetic may promote phosphatase activity. This ligand, previously used to generate a di-Mn(II) system (Suzuki et al., 1989; Sato et al., 1992), offers an opportunity to investigate the hydrolytic mechanism as it offers a limited number of possible pathways for the nucleophile.
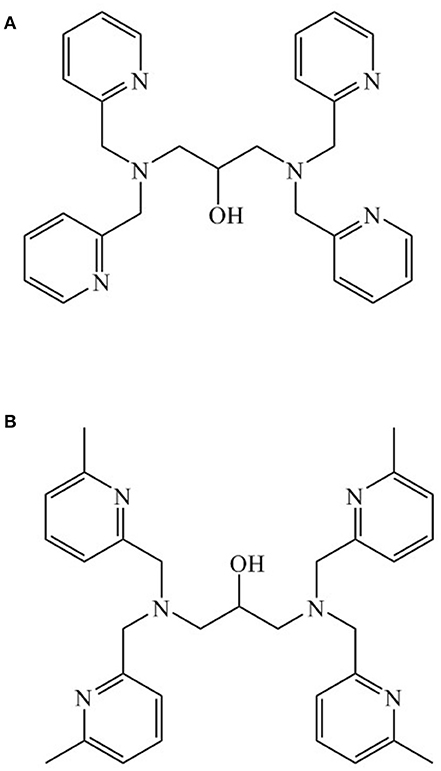
Figure 1. (A) 1,3-bis(bis(pyridin-2-ylmethyl)amino)propan-2-ol (HL1); (B) N,N,N',N'-tetrakis[(6-methyl-2-pyridyl)methyl]-1,3-diaminopropan-2-ol (Me4tpdpH).
Experimental Section
General Methods
Chemicals were purchased from Sigma-Aldrich, Merck, ABCR, Acros or Alfa Aeser and used without further purification. Reactions requiring the exclusion of moisture and/or oxygen were carried out under nitrogen atmosphere using standard Schlenk techniques. TLC was performed on TLC Silica gel 60 F254 TLC plates purchased from Merck and visualization of the spots was carried out by fluorescence quenching with 254 nm UV light. Purification of raw products by column chromatography were performed using silica gel (grade 9385, 60 Å, 230–400 mesh size) purchased from Sigma-Aldrich. NMR spectra were recorded with a Bruker Avance III 300 system at 300 K. Chemical shifts (δ) are given in ppm and coupling constants (J) in Hz. 1H and 13C spectra were referenced to the protio impurity or the 13C signal of the deuterated solvent. Abbreviations used for observed multiplicities are d for doublet, dd for doublet of doublets, td for triplet of doublets and m for multiplet. IR spectra were measured with a Perkin Elmer Frontier FT-IR spectrometer, transmittance data are given in wave number (cm−1). Abbreviations used for observed intensities are w for weak, m for medium and s for strong. UV-Vis absorption spectra were recorded with an Agilent Technologies Cary 60 UV-Vis spectrophotometer. Elemental analyses were performed by the elemental microanalysis service at the School of Chemistry & Molecular Biosciences of the University of Queensland. The synthesis of the cobalt(II) complex, [Co2(L1)(μ-OAc)](ClO4)2·0.5 H2O, is described in the Supplementary Material.
Hydrolysis Studies
Kinetic studies were conducted using a Varian Cary50 Bio UV/Visible spectrophotometer with a Peltier temperature controller (25°C) and 10-mm quartz cuvettes, and employing bis-(2,4-dinitrophenol)phosphate (BDNPP) as substrate. Assays were measured in a solvent system composed of 50:50 acetonitrile:buffer. An aqueous multicomponent buffer was employed made up of 50 mM 2-(N-morpholino)ethanesulfonic acid (MES), 4-(2-hydroxyethyl)-1-piperazineethanesulfonic acid (HEPES), 2-(N-cyclohexylamino)ethane sulfonic acid (CHES) and N-cyclohexyl-3-aminopropanesulfonic acid (CAPS), with ionic strength controlled with LiClO4 (250 mM). The pH values reported for the buffers are those of the aqueous component (Kaminskaia et al., 2000). The initial-rate method was employed and assays were measured such that the initial linear portion of the data was used for analysis. Product formation was determined by monitoring the formation of 2,4-dinitrophenolate; the extinction coefficient of this product at 400 nm varies from 7,180 at pH 4.5, 10,080 at pH 5.0; 11,400 at pH 5.5 to 12,000 at 6.0 and 12,100 at pH 6.5–11 (Smith et al., 2007). Assays to evaluate the pH dependence of the reaction contained 40 μM complex and 5 mM BDNPP; to evaluate the effect of [substrate], 0.5 mM complex was mixed with 1–11.5 mM BDNPP. Catalytic rates could be measured reliably up to pH 11.0 and were fit to the simplest possible model to describe the pH dependence of the observed catalytic rates (Kantacha et al., 2011). The model invokes one relevant protonation equilibrium and is described by an equation of the form
Here, a and b represent fitting parameters (i.e., Vmax and Ka, respectively), while x is the variable ([H+] in this case) for a function of y (representing the measurable catalytic rate v). At very high [H+] (low pH; x/b>>0) the denominator is >> Vmax and hence the rate is approximating 0. At very low [H+] (high pH; x/b~0) the denominator approximates 1 and hence the rate approaches Vmax. The fitting parameter b, representing the relevant acid dissociation constant Ka, is thus the [H+] where the rate v reaches half of its maximum value Vmax.
The catalytic rates were measured as a function of substrate concentration. Experimental limitations (imposed by the solubility of the substrate) prevented accurate measurements above 6 mM. The data displayed hyperbolic behavior but saturation was not achieved. Consequently, the data were analyzed with a combination of non-linear regression and double-reciprocal linear fits, using the Michaelis-Menten equation
where V0 is the initial rate, Vmax is the maximum rate, KM is the Michaelis constant, and [S] is the substrate concentration.
Susceptibility Measurements
The magnetic data were collected using an MPMS-XL 5T (Quantum Design) SQUID magnetometer. Fixed powder samples were prepared by pressing the powder into PTFE tape to prevent field-induced reorientation. Data were corrected for contributions of the sample holders and, using Pascal's constants (Bain and Berry, 2008), for the diamagnetic contributions of the samples. Effective magnetic moments were calculated using the relationship μeff = 2.828(χMT).
Crystallographic Measurements
Crystallographic data for the complex were collected at 190(2) K using an Oxford Diffraction Gemini Ultra dual source (Mo and Cu) CCD diffractometer with Mo (λKα = 0.71073 Å) radiation. The structure was solved by direct methods (SIR-92) and refined by full matrix least squares methods (SHELXL 97) based on F2 (Sheldrick, 1997), accessed through the WINGX 1.70.01 crystallographic collective package (Farrugia, 1999). Hydrogen atoms were fixed geometrically and not refined. X-ray data of the published structure was deposited with the Cambridge Crystallographic Data Centre, CCDC 1844565.
Computational Details
Geometry optimizations of the cations of [Ni2(L1)(μ-OAc)(H2O)2](ClO4)2·H2O and [Co2(L1)(μ-OAc)](ClO4)2·0.5H2O were undertaken with the Gaussian 09 set of programs (Frisch et al., 2013) starting from the X-ray structural data. The B3LYP functional (Becke, 1993), Noodleman's broken symmetry, (Noodleman et al., 1988) the TZV basis set (Schaefer et al., 1992). Orca 2.6.04 (Neese, 2012) was used for the magnetic coupling constant calculation essentially as described previously (Comba et al., 2009).
Synthesis
1,3-Bis(Bis(Pyridin-2-ylmethyl)Amino)Propan-2-ol (HL1)
2-(Chloromethyl)pyridine hydrochloride (4.00 eq, 6.01 g, 36.62 mmol) was dissolved in 3 mL distilled water and 15 mL of an aqueous 5 M NaOH solution was added while stirring. 1,3-diaminopropan-2-ol (1.00 eq, 825 mg, 9.15 mmol), 15 mL of 5 M NaOH solution and tetraoctylammonium bromide (0.02 eq, 100 mg, 183 μmol) were then added. The resulting red mixture was stirred at room temperature overnight. The reaction mixture was transferred into a separatory funnel with 40 mL chloroform, 40 mL of brine, the aqueous phase was extracted twice with 10 mL chloroform, and the combined organic layers washed with 50 mL water. The separated organic layer was dried over magnesium sulfate, filtered and concentrated under reduced pressure. The crude product was purified using a MeOH-equilibrated silica column (Merck) according to the manufacturer's instructions. After removal of the solvent under reduced pressure the product was obtained as orange oil (88%, 3.66 g, 8.05 mmol). 1H NMR (300 MHz, CDCl3): δ 8.49–8.45 (4H, m), 7.54 (4H, td, J = 11.5 Hz, J = 1.8 Hz), 7.37–7.31 (4H, m), 7.12–7.05 (4H, m), 4.03–3.92 (1H, m), 3.89 (4H, d, 2J = 14.7 Hz), 3.84 (4H, d, 2J = 14.7 Hz), 2.69 (2H, dd, 2J = 13.3 Hz, 3J = 4.1 Hz), 2.59 (2H, dd, 2J = 13.3 Hz, 3J = 7.8 Hz) ppm. 13C NMR (75 MHz, CDCl3): δ 159.3, 149.0, 136.5, 123.2, 122.1, 67.2, 60.8, 59.1 ppm.
[Ni2(L1)(μ-OAc)(H2O)2](ClO4)2·H2O
Nickel(II) acetate tetrahydrate (2.00 eq, 109.5 mg, 440 μmol) was dissolved in 6 mL MeOH. Lithium perchlorate trihydrate (4.00 eq, 153.4 mg, 880 μmol) and 4 mL of a methanol solution of 1,3-bis(bis(pyridin-2-ylmethyl)amino)propan-2-ol (HL1) (1.00 eq, 100.0 mg, 220 mmol) were added and the reaction mixture stirred under reflux for 1 h. Upon cooling to room temperature, the solvent was removed under reduced pressure and the resulting product was recrystallized in a water/acetone mixture resulting in blue crystals (46%, 89.6 mg). IR: = 3489 (w), 3221 (w), 2801 (w), 1651 (m), 1606 (s), 1548 (s), 1482 (m), 1445 (s), 1427 (s), 1287 (m), 1161 (m), 1070 (s), 1056 (s), 1037 (s), 1023 (s), 990 (m), 928 (m), 886 (m), 760 (s), 620 (s) cm−1. UV/vis λmax (ε), 950 nm (57 M−1cm−1), 590 nm (43 M−1cm−1). Calc. for C29H38Cl2N6 Ni2O14: C, 39.45; H, 4.34; N, 9.52 %. Found: C, 39.49; H, 4.13; N, 9.37%.
Results and Discussion
Syntheses
The ligand 1,3-bis(bis(pyridin-2-ylmethyl)amino)propan-2-ol (HL1) was prepared by a modification of previously described procedures (Suzuki et al., 1989; Sato et al., 1992). The nomenclature HL1 indicates that the ligand is protonated at the hydroxido moiety and on formation of the complex the ligand is deprotonated and coordinates as the monoanion L1−. We have reported previously a diZn(II) complex with L1− as a functional model for zinc(II) phosphoesterase enzymes (Mendes et al., 2016).
In this work the dinuclear nickel complex [Ni2(L1)(μ-OAc)(H2O)2](ClO4)2.H2O was synthesized from a reaction of HL1 with two equivalents of nickel(II) acetate in methanol solution in the presence of LiClO4. The nickel complex was obtained as bright blue crystals after recrystallization in a water/acetone mixture.
A complex with the same ligand and formulated as [Ni2(L1)(μ-OAc)2](PF6).MeOH has been reported previously (Moffat et al., 2014). In that case the synthesis involved the reaction of the ligand HL1, nickel(II) acetate in methanol, in the presence of triethylamine and NaPF6 under reflux. On standing at −18°C the pink crystals which initially formed were removed, the mother liquor collected and concentrated under vacuum and upon slow diffusion of diethylether on standing the deep blue crystals of [Ni2(L1)(μ-OAc)2](PF6). MeOH were collected and structurally characterized (Moffat et al., 2014). In addition, the Ni(II) complex of a similar ligand N,N,N',N'-tetrakis((6-methyl-2-pyridyl)methyl)-1,3-diaminopropan-2-ol (Me4tpdpH), prepared by reaction of nickel(II) acetate, NaClO4 with Me4tpdpH in methanol at room temperature, and crystallized from a methanol/diethyl ether solution as light green crystals, has also been reported. The complex was formulated as [Ni2(Me4tpdp)(μ-OAc)(ClO4)(CH3OH)](ClO4) (Yamaguchi et al., 1997, 2001). The synthesis and characterization of the cobalt complex [Co2(L1)(μ-OAc)](ClO4)2 has been reported previously (Siluvai and Murthy, 2009).
Spectroscopy
The infrared spectrum of [Ni2(L1)(μ-OAc)(H2O)2](ClO4)2 displayed bands attributed to the asymmetric and the symmetric acetate stretch (vas = 1,548 cm−1, vs = 1,445 cm−1), indicating the presence of a bridging acetate anion (Deacon and Phillips, 1980). Furthermore, characteristic bands at 1,606 cm−1 and 1,576 cm−1 were assigned to vC=N and vC=C of the pyridyl groups of the ligand, and those 1,070 cm−1 to the perchlorate counter ion.
The electronic spectrum of [Ni2(L1)(μ-OAc)(H2O)2](ClO4)2 was measured in acetonitrile. For octahedrally coordinated Ni(II) ions there are three spin allowed d-d transitions from the 3A2g ground state to the higher excited triplet states 3T2g, 3T1g, and 3T1g (P). Spectral bands with maxima at 950 and 590 nm were assigned to the 3A2g→3T2g and 3A2g→3T1g transitions, respectively. The 3A2g→3T1g (P) transition is probably hidden under the intense band of the pyridyl groups with a maximum at 255 nm; a small shoulder around 400 nm could arise from this spin-allowed transition. A shoulder around 800 nm is assigned to the spin-forbidden d-d 3A2g→1E1g transition. The Racah parameter B and the ligand field splitting energy Dq of the d8 Ni(II) system were determined using the bands of the 3A2g→3T2g and 3A2g→3T1g transitions and determined to be 877 and 1,053 cm−1, respectively.
X-Ray Crystal Structure
Selected crystallographic data for [Ni2(L1)(μ-OAc)(H2O)2](ClO4)2·H2O are shown in Table 1 and selected bond lengths and angles are displayed in Table 2. An ORTEP plot is shown in Figure 2. The structure is composed of the ligand mono-anion, two metal(II) ions and a bridging acetate with the Ni(II) structure containing two coordinated aqua ligands completing the hexacoordinate coordination sphere. The charge is balanced by perchlorate ions. In the diNi(II) complex there is disorder around the carbon atom of the bridging hydroxide. The coordination symmetry around each Ni(II) site for [Ni2(L1)(μ-OAc)(H2O)2](ClO4)2·H2O is octahedral and the donor set of both nickel atoms is N3O3, which is composed of a tertiary amino-N atom (Ni(1)-N(2): 2.110(4) Å), two pyridyl-N atoms (Ni(1)-N(1): 2.078(3) Å; Ni(1)-N(3): 2.080(4) Å), a bridging alkoxo-O atom (Ni(1)-O(1): 1.990(2) Å), an μ-acetato O atom (Ni(1)-O(2): 2.058(3) Å) and an O atom of a coordinating water molecule (Ni(1)-O(3): 2.132(3) Å). The bridging angle Ni-O(1)-Ni is 131.6° and the two nickel atoms are separated by a distance of 3.63 Å. The C(8) atom is disordered between two different positions.
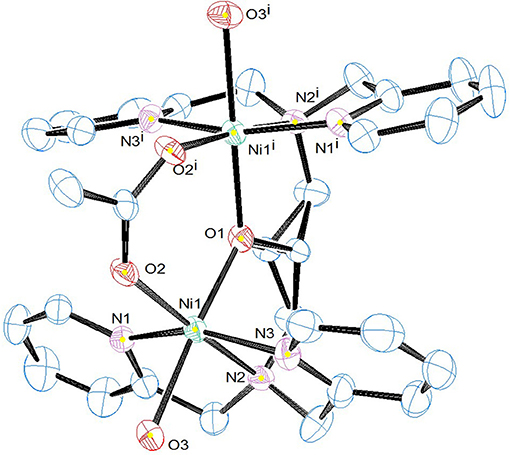
Figure 2. ORTEP plot of the cation [Ni2(L1)(μ-OAc)(H2O)2]2+. Counter ions and hydrogen atoms have been omitted for clarity (25% ellipsoid probability in ORTEP plot).
For the previously reported complex [Ni2(L1)(μ-OAc)2](PF6).MeOH, the two Ni(II) octahedral sites are composed of two μ-acetate ligands, the three N donors of the L1 ligand, and the μ-O of the ligand (Figure 3, top; Moffat et al., 2014). The differences in the two diNi(II) structures of L1− involve the absence of the second bridging acetate ligand and the presence of the two terminal water molecules in [Ni2(L1)(μ-OAc)(H2O)2](ClO4)2·H2O, as well as the presence of the ClO4 − anions. Furthermore, the coordinating groups present in the ligand arms show a meridional coordination mode, which induces the coordination of the water molecules in positions anti to each other.
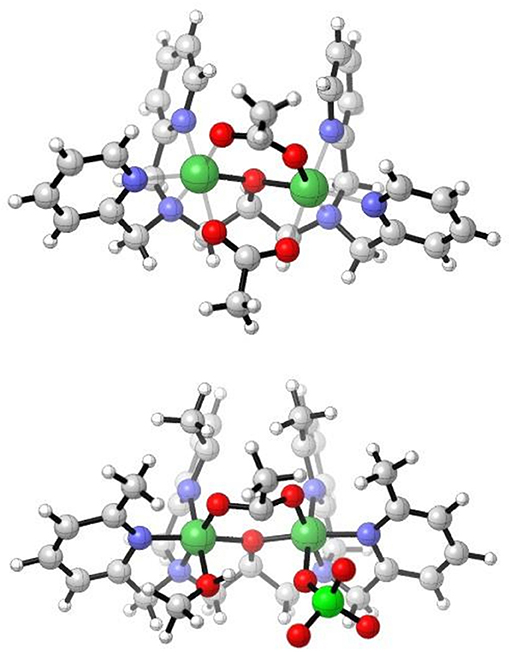
Figure 3. Cations of (Top) [Ni2L1(μ-OAc)2]+ (Moffat et al., 2014), and (Bottom) [Ni2(Me4tpdp)(μ-OAc)(ClO4)(CH3OH)]+ from the X-ray structures (Yamaguchi et al., 1997, 2001).
The X-ray crystal structure of [Ni2(Me4tpdp)(μ-OAc)(ClO4)(CH3OH)](ClO4) has been reported (Figure 3, bottom; Yamaguchi et al., 1997, 2001). The structure shows that both Ni(II) sites are six coordinate, the two metal ions separated by 3.62 Å and bridged by the ligand μ-O alkoxide and μ-acetato ligands. The sixth coordination site of one Ni(II) is made up of the O from a perchlorato ligand, whilst for the other metal ion a CH3OH ligand completes the sixth coordination (Yamaguchi et al., 1997, 2001). Whereas for the complex [Ni2(L1)(μ-OAc)(H2O)2](ClO4)2·H2O the coordinated aqua ligands are trans to the μ-alkoxo-O atom, effectively bisecting the two pyridyl rings of the ligand, this arrangement is not observed in the structure of the analogous [Ni2(Me4tpdp)(μ-OAc)(ClO4)(CH3OH)](ClO4) complex. Here, the methanol and perchlorato ligands are located cis to the μ-alkoxo-O atom (Yamaguchi et al., 1997), and presumably replaced by aqua ligands in solution, an important consideration in subsequent hydrolytic studies (Yamaguchi et al., 2001).
The X-ray crystal structure of [Co2(L1)(μ-OAc)](ClO4)2 has been reported (Siluvai and Murthy, 2009). The structure shows a pseudo C2-axis of symmetry with the μ-acetate ligand bridging the two Co(II) ions in a symmetric μ-1,3 mode. The two Co(II) sites display slightly distorted trigonal bipyramidal geometry with τav, the index of trigonality, equal to 0.93; a τ value of unity would perfect trigonal bipyramidal geometry (Addison et al., 1984; Siluvai and Murthy, 2009).
Magnetic Susceptibility
The magnetic susceptibility of the diNi(II) and diCo(II) complexes was measured over the temperature range 300 to 7 K in an applied field of 500 G. The χM vs. T, and χMT vs. T plots are presented in Figure 4 for [Ni2(L1)(μ-OAc)(H2O)2](ClO4)2·H2O and Figure 5 for [Co2(L1)(μ-OAc)](ClO4)2·0.5H2O. For both complexes the susceptibility data were fitted using the program PHI (Chilton et al., 2013) with the isotropic exchange Hamiltonian = −2J.
For [Ni2(L1)(μ-OAc)(H2O)2](ClO4)2·H2O the χMT vs. T plot indicates antiferromagnetic coupling between the two Ni(II) centers, with χMT gradually decreasing from 3.63 cm3 K mol−1 (μeff = 5.39 μB) at 300 K to 0.075 cm3 K mol−1 (μeff = 0.77 μB) at 8 K. The room temperature value of χMT is larger than the spin-only value for two non-interacting high-spin nickel(II) ions (χMT = 2.00 cm3 K mol−1, μSO = 4.00 μB, g = 2.00, S = 1). While no orbital angular momentum is expected for the 3A2g ground state of the octahedral d8 centers, it is expected for the excited 3T2g and 3T1g states. The theoretical χMT value with orbital angular momentum included is 4.99 cm3 K mol−1 (μSL = 6.32 μB, L = 3), which suggests some orbital contributions are present in this case. The best fit gave parameters J = −27.4 cm−1, g = 2.29, D = 28.4 cm−1 and χTIP = 4.75 × 10−9 m3 mol−1. The inclusion of neither a rhombic zero-field splitting (ZFS) parameter E nor an intermolecular magnetic exchange parameter zJ led to an improvement of the fit and were thus omitted.
For [Co2(L1)(μ-OAc)](ClO4)2·0.5H2O the magnetic moment has been reported as 4.09 μB/Co(II), measured in d3-acetonitrile at room temperature (Siluvai and Murthy, 2009). In the study reported herein, the variable temperature magnetic susceptibility of the complex was measured from 300–7 K. The χMT value at 300 K is 4.23 cm3 K mol−1 (μeff = 5.82 μB), which is within the range of similar binuclear cobalt(II) complexes (Zeng et al., 2004; Tian et al., 2007, 2008; Massoud et al., 2008; Jung et al., 2009; Daumann et al., 2013a; Khandar et al., 2015; Li et al., 2015; Alam et al., 2016). The value is higher than the expected spin-only value for two non-interacting high-spin cobalt(II) ions (3.74 cm3 K mol−1, μSO = 5.47 μB, g = 2.00, S = 3/2) but significantly lower than expected with the inclusion of orbital angular momentum (6.76 cm3 K mol−1, μSL = 7.35 μB, L = 3), suggesting only very minor orbital contributions. The value of χMT shows a gradual decrease with decreasing temperature and reaches 0.025 cm3 K mol−1 at 7 K, indicative of antiferromagnetic coupling between the two centers. The best fit to the data gave parameters J = −14.9 cm−1, g = 2.16 and TIP = 2.22 × 10−9 m3 mol−1. The inclusion of intermolecular magnetic exchange zJ was found to have little effect on the fit and, therefore, was not included. The fitted g value is larger than the free ion g value (ge = 2.00) and is explained by second-order effects. While the 4A2′ ground state arising from the trigonal bipyramidal coordination of a d7 ion has no orbital angular momentum, admixture of the excited 4E″ state with the orbital angular momentum introduces second-order orbital momentum, resulting in a larger g value and magnetic moment (Hempel and Miller, 1981; Hossain and Sakiyama, 2002; Bai et al., 2005).
A computational study was undertaken employing the B3LYP functional, Noodleman's broken symmetry, the TZV basis set and the Orca set of programs (Noodleman et al., 1988; Schaefer et al., 1992; Becke, 1993; Neese, 2012; Frisch et al., 2013) in order to calculate the magnitude of the coupling in both complexes (Comba et al., 2009). The calculations were performed based on the X-ray structural parameters for the respective complexes. For the [Ni2(L1)(μ-OAc)(H2O)2](ClO4)2·H2O complex the computed value of 2J was similar to the experimentally determined value (−26.4 cm−1 compared with −27.4 cm−1, respectively). For [Co2(L1)(μ-OAc)](ClO4)2·0.5H2O the difference in the calculated and experimental values was greater (−20.1 cm−1 compared with −14.9 cm−1, respectively).
Attempts have been made to correlate structural parameters with the strength of coupling for both diNi(II) and diCo(II) complexes (Nanda et al., 1994a,b,c; Schultz et al., 1997; Johansson et al., 2008; Tomkowicz et al., 2012; Daumann et al., 2013b). In the case of diNi(II) complexes, an initial study reported a linear correlation between the magnitude and sign of J with the Ni-O-Ni angle for five diNi(II) complexes of the macrocyclic ligand 15,95-dimethyl-3,7,11,15-tetraaza-1,9(1,3)-dibenzenacyclohexadecaphane-12,92-diol, with aqua, thiocyanate, methanol, imidazole, and pyridine ligands completing the coordination sphere (Nanda et al., 1994c). The authors proposed that at an Ni-O-Ni angle of 97° a cross over from antiferro- to ferro-magnetic coupling occurred (Nanda et al., 1994c). The study was subsequently expanded to include examples of other diNi(II) complexes with phenoxido-bridged ligands and it was concluded that, with the availability of more structural data, a more definitive correlation between J and the Ni-O-Ni angle could be expected (Nanda et al., 1994a,b). In line with the previous analysis of the relationship between J and Ni-X bonds lengths it was suggested that, in hexacoordinate complexes, the antiferromagnetic coupling was amplified with an increase in bond lengths of one of the axial bonds (Nanda et al., 1994a). Further, the studies suggested that a significant increase in the magnitude of -J occurred in the situation where a tetragonally elongated hexacoordinate complex transformed to a five-coordinate square pyramidal complex (Nanda et al., 1994a). Subsequently, a series of studies reported and expanded on the correlations between the sign and magnitude of J and both the Ni-O-Ni and Ni-X bond lengths (Allen et al., 1978; Wages et al., 1993; Halcrow and Christou, 1994; Adams et al., 2001; Bu et al., 2001; Mochizuki et al., 2004; Prushan et al., 2007; Greatti et al., 2008; Pawlak et al., 2008; Mandal et al., 2009; Chattopadhyay et al., 2010; Ren et al., 2011; Biswas et al., 2012, 2017; Botana et al., 2014; Mahapatra et al., 2016; Massoud et al., 2016; Sanyal et al., 2016; Xavier and Neves, 2016). As the number of studies, and hence the number of examples of diNi(II) complexes with various bridging ligand types, has increased the relationship between J and a structural parameter (Ni-O-Ni angle; Ni-X distance) has been described in terms of both linear, albeit with considerable scatter (Mahapatra et al., 2016), and polynomial functions (Bu et al., 2001). Clearly, the relationship is dependent on a set of parameters and even on the type of bridging ligands (Krupskaya et al., 2010; Botana et al., 2014). The sign and magnitude of J (−27.4 cm−1) for [Ni2(L1)(μ-OAc)(H2O)2](ClO4)2·H2O are consistent with the NiII-μO-NiII angle of 131.6(2)° (Table S1 and Figure S1) (Nanda et al., 1994c; Mochizuki et al., 2004; Biswas et al., 2012, 2017; Massoud et al., 2016).
The relationship between the structural parameters and the magnitude of the observed magnetic coupling for dicobalt(II) complexes has been reviewed (Arora et al., 2012, p. 703; Tomkowicz et al., 2012; Daumann et al., 2013a). It was concluded that for complexes with a μ-Obridge/bis(μ2-RCOO-κ2O:O') core, the variations in magnitude of J could be related to the type of μ-Obridge, the Co-Obridge-Co angle and the type of R-group (Tomkowicz et al., 2012). Further, it was proposed that the strength of the coupling varied according to bridge type (μ-O2−>μ-OH−>μ-H2O) (Schultz et al., 1997), and that Co(II)-O-Co(II) bond angles around 96° in some examples resulted in ferromagnetic coupling via orthogonal magnetic orbitals (Tudor et al., 2008; Fabelo et al., 2009; Tomkowicz et al., 2012), and it was also suggested that bis(μ2-syn,syn-CH3COO-κ2O:O') bond angles were important (Arora et al., 2012). Extremely weak antiferro- or ferro-magnetic coupling was proposed for diCo(II) complexes with the μ-O(phenoxido);bis(μ2-OAc-κ2O:O') bridge, although exceptions occurred (Arora et al., 2012; Daumann et al., 2013a). Complexes with the μ-H2O;bis(μ2-RCOO-κ2O:O') core appear to promote weak antiferromagnetic coupling, although stronger than that seen with the μ-O(phenoxido) analog; the bis(μ2-RCOO-κ2O:O');μ2-O;κ2O,O'-CH3COO core appeared to promote ferromagnetic coupling (Daumann et al., 2013a). The relationship between Co(II)-X bond distances and the magnitude and sign of J was extremely weak, and both the Co(II).Co(II) distance and extent of distortion around the Co(II) center seemingly having little bearing on the coupling (Daumann et al., 2013a). Of the structural parameters considered in this analysis, the Co(II)-X-Co(II) bridge angles appear to have some influence on the sign and magnitude of J (Daumann et al., 2013a), in agreement with earlier studies, but none of the structural relationships appears to be particularly strong (Table S2 and Figure S2; Johansson et al., 2008; Tudor et al., 2008; Fabelo et al., 2009; Tomkowicz et al., 2012).
Phosphoesterase Activity
The pH dependence of BDNPP hydrolysis by [Ni2(L1)(μ-OAc)(H2O)2](ClO4)2·H2O was analyzed between pH 4.75 and pH 11.0; the rate enhances sharply at pH values ≥9.0, reaching a maximum at pH ≥11.0. Data were fit as described in the Experimental section and resulted in an estimate of the pKa value (9.7 ± 0.1) of the catalytically relevant protonation equilibrium (Figure 6A). Since the measurements of the catalytic rates as a function of [S] did not reach full saturation obtained catalytic parameters need to be viewed with some caution. A combination of non-linear and linear regression analyses indicate that plausible values for Vmax and Km are around 6–7 × 10−5 M.min−1 and 10 mM, respectively (Figure 6B). For the following comparison of the catalytic efficiencies of a number of Ni-dependent biomimetics we will use the rate of hydrolysis of 6 mM [S] by 40 μM [Ni2(L1)(OAc)(H2O)2](ClO4)2·H2O, i.e., 2.50 × 10−5 M.min−1. This rate corresponds to a kcat of ~0.01 s−1.
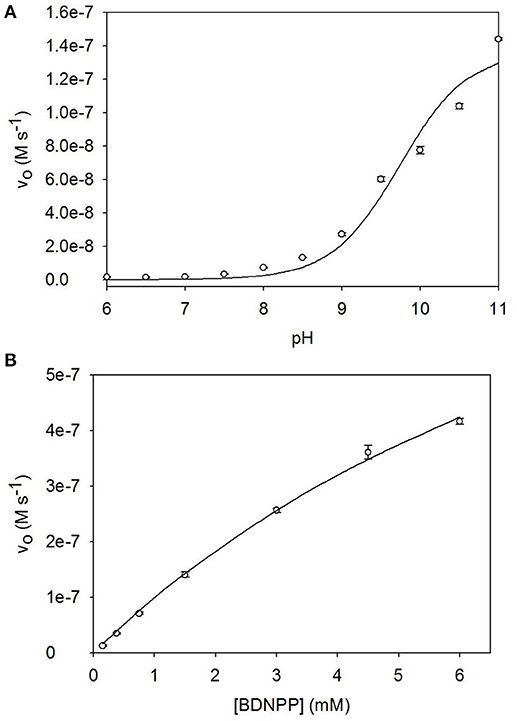
Figure 6. (A) pH dependence profile, and (B) Michaelis-Menten plot for the hydrolysis of BDNPP catalyzed by [Ni2(L1)(μ-OAc)(H2O)2](ClO4)2·H2O.
Several previous studies focused on the phosphatase-like activity of di-Ni(II) complexes (Table 3 and Scheme 1; Yamaguchi et al., 1997, 2001; Yamane et al., 1997, 2001; Parimala and Kandaswamy, 2003; Jikido et al., 2005; Greatti et al., 2008; Ren et al., 2011; Piovezan et al., 2012; Wu and Wang, 2014; Massoud et al., 2016; Xavier and Neves, 2016). In a number of cases the experimental conditions in terms of solvent (acetonitrile/aqueous buffer) and substrate (BDNPP) were similar to those employed in the present work, although the pH at which the kcat and Km values were determined did vary, making direct comparisons difficult (Greatti et al., 2004, 2008; Piovezan et al., 2012; Massoud et al., 2016; Xavier and Neves, 2016). The nucleophilic agent in these reactions has been proposed as either a terminal (Yamaguchi et al., 2001; Vichard and Kaden, 2002; Parimala and Kandaswamy, 2003; Jikido et al., 2005; Greatti et al., 2008; Massoud et al., 2016; Sanyal et al., 2016) or a bridging (Ren et al., 2011; Piovezan et al., 2012; Wu and Wang, 2014) hydroxido moiety.
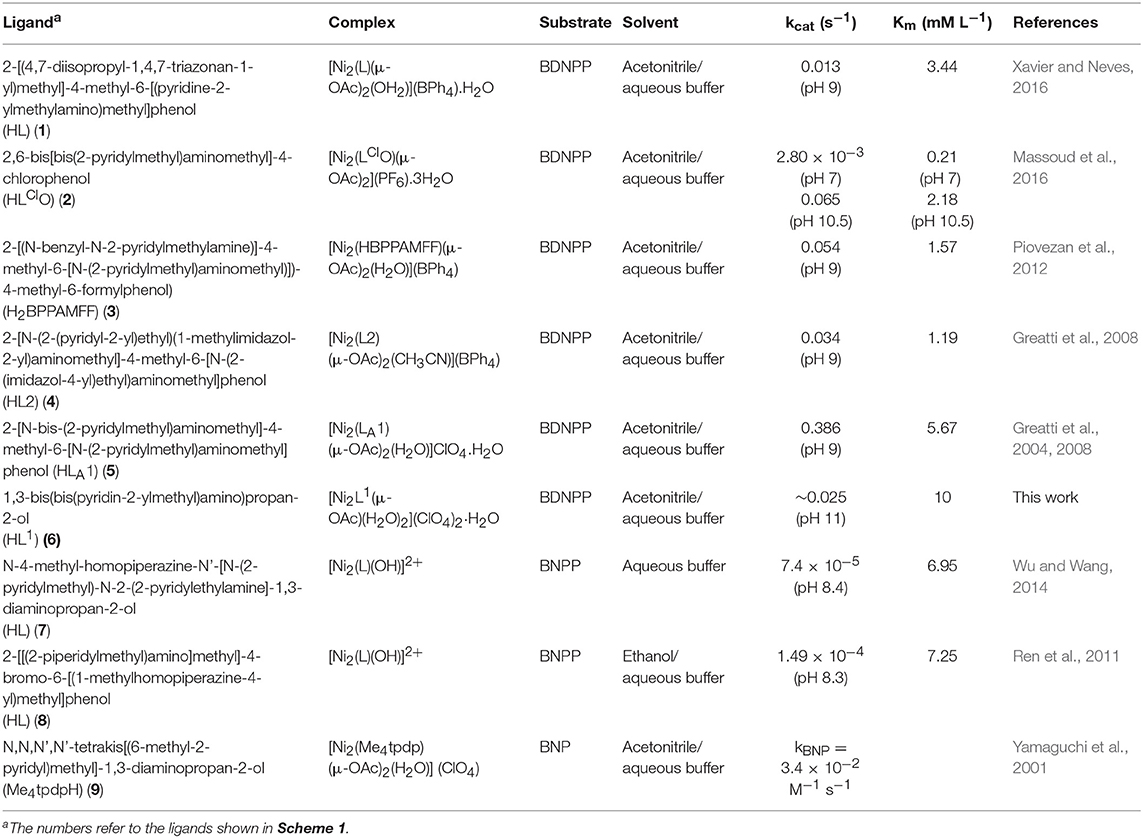
Table 3. Michaelis Menten kinetic data of dinuclear Ni(II) complexes that mimic metallophosphatases.
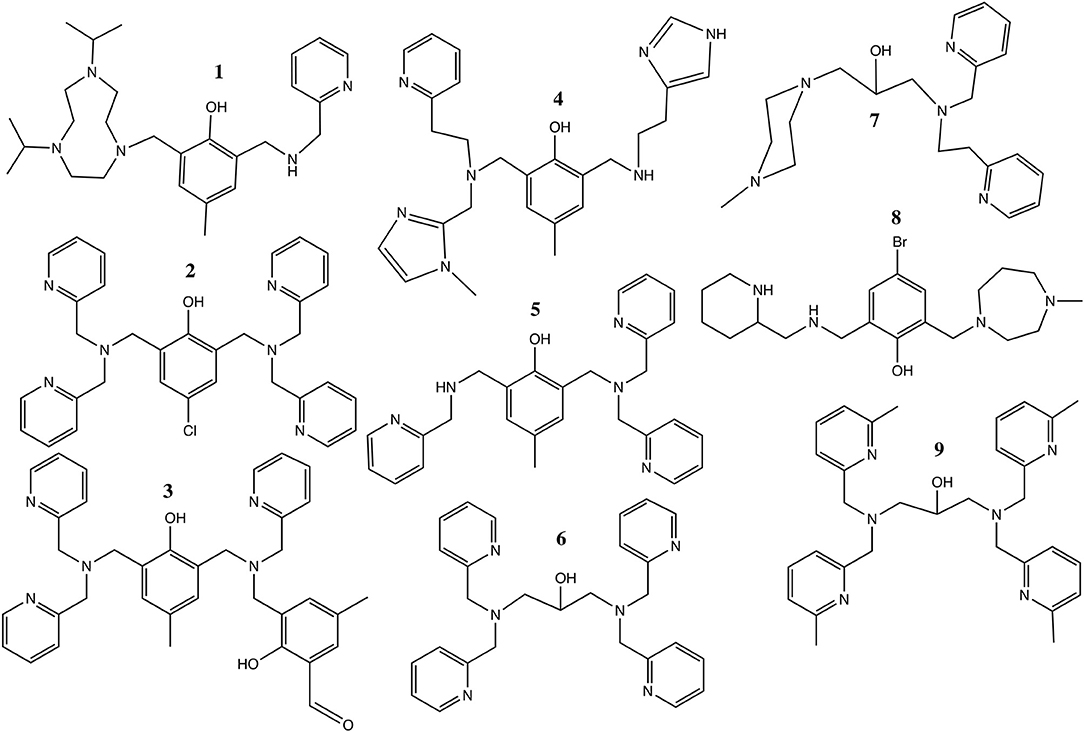
Scheme 1. The ligands listed in Table 3.
Of the examples listed in Table 3, the complex with the ligand 2-[N-bis-(2-pyridylmethyl)aminomethyl]-4-methyl-6-[N-(2-pyridylmethyl)aminomethyl]phenol, [Ni2(LA1) (μ-OAc)2(H2O)]ClO4.H2O, was the most effective and efficient catalyst for the hydrolysis of BDNPP with kcat = 0.386 s−1 at pH 9 (Greatti et al., 2008). The rate enhancement was rationalized in terms of the fact that in solution at pH 9.00, the active species [Ni2(LA1)(H2O)2(μ-OH)]2+ made up approximately 85% of the complexes present in solution (Greatti et al., 2008). The proposed mechanism for the reaction involved initial loss of the bridging acetate ligands and coordination of BDNPP after replacement of one aqua ligand (Greatti et al., 2008). The substrate is thus proposed to coordinate in a monodentate manner and orient cis to a terminal Ni-OH moiety, the latter promoting a nucleophilic attack on the phosphorus atom with release of the nitrophenolate anion and formation of a bridging DNP molecule. A subsequent intramolecular nucleophilic attack by a μ-OH moiety on the μ-DNP was proposed to result in loss of a second nitrophenolate anion and formation of coordinated phosphate anion which was subsequently replaced by H2O/OH− to complete the cycle (Greatti et al., 2008). In the case of the complex [Ni2(Me4tpdp)(μ-OAc)2(H2O)](ClO4), a mechanism was proposed whereby the substrate, bis(4-nitrophenyl)phosphate (BNP) was coordinated in a μ-1,3 manner to the diNi(II) complex, after loss of the acetate ligands, with subsequent nucleophilic attack by a terminal Ni-OH moiety at the phosphorus center (Yamaguchi et al., 2001). In that case the reaction is facilitated by the fact that the relevant hydroxido ligand is arranged cis, and thus adjacent, to the substrate (Yamaguchi et al., 2001). A variation of the above mechanisms was proposed for [Ni2(LClO)(μ-OAc)2](PF6).3H2O by Massoud et al.; in the initial phase of the catalytic cycle one of two metal-bridging acetate groups is displaced and the substrate BDNPP binds to one of the Ni(II). Subsequently, dependent on pH, the attack of a terminal hydroxide that either resides on the same (high pH) or the opposite (low pH) metal as the substrate attacks the phosphorus moiety, leading to the DNPP product being coordinated either bidentately to one metal or to both metals, respectively (Massoud et al., 2016).
For [Ni2(L1)(μ-OAc)(H2O)2](ClO4)2.H2O both Ni(II) sites are six-coordinate. Loss of the μ-acetato ligand is thus a prerequisite for catalytic activity as it generates vacant positions for the substrate to bind (Figure 7A). However, according to the crystal structure (Figure 2) none of the available aqua/hydroxido ligands are positioned suitably to act as nucleophiles for the reaction. We thus propose that the release of the μ-acetato group enables the monodentate coordination of the substrate to one of the Ni(II) ions and a water molecule (with a pKa of ~9.7) to the other metal ion (Figure 7B). The subsequent attack by the deprotonated water ligand on the phosphorus moiety of the substrate triggers catalysis (Figure 8). Insofar, the model resembles that of the lower pH mechanism proposed by Massoud et al. (2016). In the latter complex the presence of two metal-bridging acetate groups provides the basis for an enhanced mechanistic flexibility (i.e., low and high pH pathways), where the nucleophile can be either bound to the same or the opposite metal ion as the substrate.
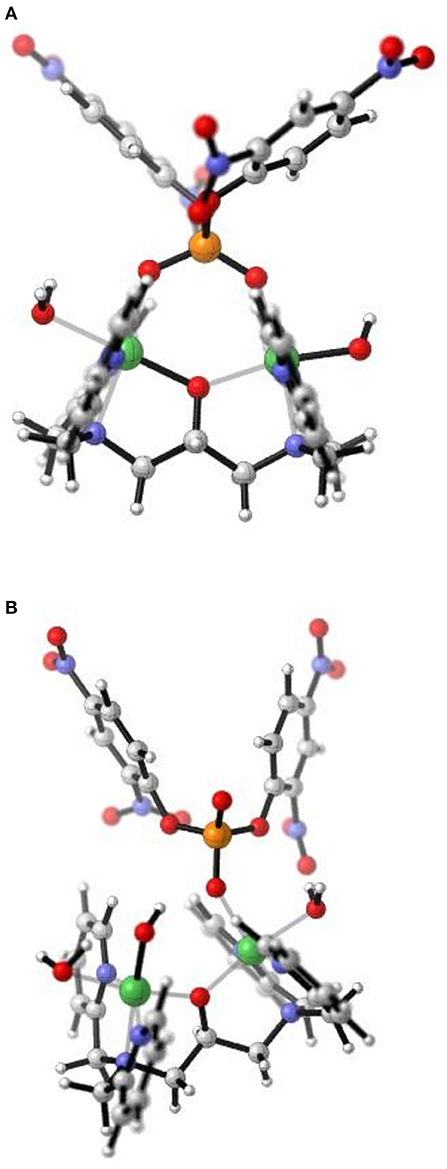
Figure 7. After loss of the μ-acetato ligands in the complex [Ni2(L1)(μ-OAc)(H2O)2] 2+ these structures represent (A) μ-1,3 coordination of BDNPP− to the two Ni(II) sites with two aqua ligands positioned trans to the μ-alkoxo moiety; and (B) monodentate coordination of BDNPP- with a OH/H2O ligand occupying the vacant site on the second Ni(II) and poised as the intramolecular terminal nucleophile. Structures generated from DFT calculations.
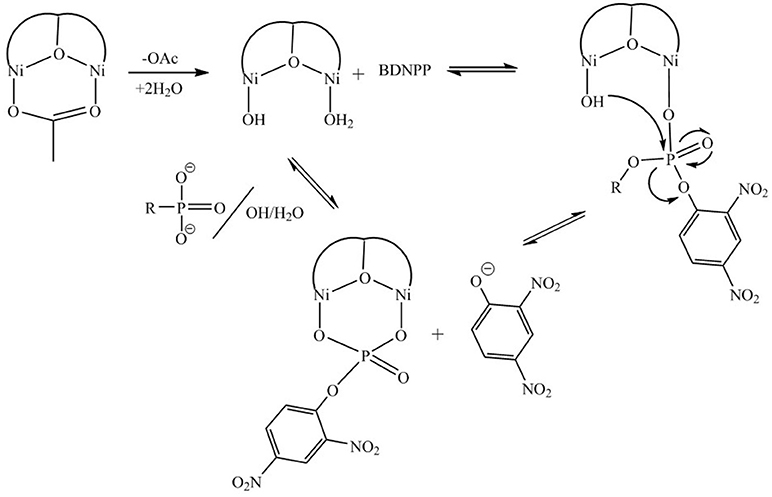
Figure 8. Proposed mechanism of reaction between [Ni2(L1)(μ-OAc)(H2O)2]2+ and BDNPP− adapted from (Vichard and Kaden, 2004). The proposed mechanism resembles that of the lower pH mechanism proposed by Massoud et al. (2016).
The phosphatase-like activity of the analogous [Co2L1(μ-OAc)](ClO4)2·0.5.H2O could not be investigated under corresponding experimental conditions since the initially red colored solution turned yellow after the addition of the aqueous buffer solution. The absorbance of the mixture at 400 nm increased upon standing, making any attempt to measure the formation of the dinitrophenolate anion difficult. The change of color of the solution may be explained by the oxidation of cobalt(II) ions (Suzuki et al., 1990).
Conclusions
The dinickel(II) and dicobalt(II) complexes of 1,3-bis(bis(pyridin-2-ylmethyl)amino)propan-2-ol have been prepared and some of their properties were compared. Magnetic susceptibility studies confirmed that the two metal ions in both complexes are antiferromagnetically coupled and computational studies verified the experimental magnetic coupling constants. Attempted correlation of the relationship between structural parameters, particularly the M-O-M angles, with the strength of the magnetic coupling were only partially successful. Kinetic analysis with the activated substrate BDNPP suggested that for the diNi(II) complex a terminal water is the nucleophile with a kinetically relevant pKa of 9.7 ± 0.1 and a kcat value as high as 0.025 s−1 (Table 3). The complex is thus at the higher end of the range for catalytic efficiency for similar diNi(II) complexes with this substrate (the corresponding diCo(II) complex was found to oxidize readily in the buffer solution). Thus, although no suitable nucleophile (OH−) is present in the original molecule the replacement of the two acetate bridges by water and/or substrate molecules (Figure 8) may not be rate-limiting. The complex is proposed to employ a similar mechanism as proposed for a series of analogous model systems for enzymes such as PAPs (Smith et al., 2009; Comba et al., 2012a,b; Bernhardt et al., 2015; Roberts et al., 2015; Bosch et al., 2016). The majority of complexes listed in Table 3 attain optimal catalytic efficiency under alkaline conditions (>pH 9.0), somewhat higher than the pH optimum of the di-Ni(II) enzyme urease (pH 7.4). This difference may be due to the fact that the majority of model systems use a terminally bound nucleophile to initiate the hydrolytic reaction, whereas urease employs a metal ion-bridging hydroxide (Zambelli et al., 2011). Nonetheless, it is apparent that the complexes listed in Table 3 represent suitable functional models for biological catalysts such as ureases and PAPs.
Author Contributions
AH designed the project and supervised the synthetic chemistry and hydrolytic experiments, contributed to the writing of the manuscript. DE undertook the syntheses of the ligand and the metal complex and undertook the spectroscopic characterization. AR undertook the magnetochemical study and analyzed the results. PC in association with AR supervised the magnetochemical study and data analysis. GS contributed to the design of the project, supplied the funding for the work, and contributed to the writing of the manuscript. EK contributed to the writing of the DFT section of the manuscript and assisted with DFT calculations. LG undertook the X-ray structure analysis, contributed to the analysis of the kinetic data, undertook the DFT studies, and contributed to the writing of the manuscript.
Conflict of Interest Statement
The authors declare that the research was conducted in the absence of any commercial or financial relationships that could be construed as a potential conflict of interest.
Acknowledgments
AR gratefully acknowledges financial support from the Heidelberg Graduate School of Mathematical and Computational Methods for the Sciences (HGS MathComp), founded by DFG grant GSC 220 in the German Universities Excellence Initiative. Computer resources were provided by the National Facility of the Australian National Computational Infrastructure and by the University of Queensland Research Computing Centre. We are grateful for funding support from the Australian Research Council (DP150104358). AH thanks the financial support received from CAPES-Brazil (99999.006336/2014-00). We also thank Dr. Bodo Martin, Anorganisch-Chemisches Institut, Universität Heidelberg, Germany, for assistance with the computations.
Supplementary Material
The Supplementary Material for this article can be found online at: https://www.frontiersin.org/articles/10.3389/fchem.2018.00441/full#supplementary-material
References
Adams, H., Bradshaw, D., and Fenton, D. E (2001). Dinuclear nickel(II) and zinc(II) complexes of 2,6-[N,N'-bis(2-hydroxyphenylmethyl)-N,N'-bis(2-pyridylmethyl)aminomethyl]-4-methyl-phenol. Supramol. Chem. 13, 513–519. doi: 10.1080/10610270108028297
Addison, A. W., Rao, T. N., Reedijk, J., Van Rijn, J., and Verschoor, G. C. (1984). Synthesis, structure, and spectroscopic properties of copper(II) compounds containing nitrogen-sulfur donor ligands: the crystal and molecular structure of aqua[1,7-bis(N-methylbenzimidazol-2'-yl)-2,6-dithiaheptane]copper(II) perchlorate. J. Chem. Soc. Dalton Trans. 1349–1356. doi: 10.1039/DT9840001349
Alam, R., Pal, K., Shaw, B. K., Dolai, M., Pal, N., and Ali, M. (2016). Synthesis, structure, catalytic and magnetic properties of a pyrazole based five coordinated di-nuclear cobalt(II) complex. Polyhedron 106, 84–91. doi: 10.1016/j.poly.2015.12.062
Allen, K. G., Stewart, J. A., Johnson, P. E., and Wettlaufer, D. G. (1978). Identification of the ionic groups of papain by pH/rate profile analysis. Eur. J. Biochem. 87, 575–582. doi: 10.1111/j.1432-1033.1978.tb12409.x
Arora, H., Barman, S. K., Lloret, F., and Mukherjee, R. (2012). Isostructural dinuclear phenoxo/acetato bridged mangnaese(II), cobalt(II) and zinc(II) complexes with labile sites: kinetics of transesterification of 2-hydroxypropyl-p-nitrophenylphosphate. Inorg. Chem. 51, 5539–5553. doi: 10.1021/ic201971t
Bai, S.-Q., Gao, E.-Q., He, Z., Fang, C.-J., and Yan, C.-H. (2005). Crystal structures and magnetic behavior of three new azido-bridged dinuclear cobalt(II) and copper(II) complexes. New J. Chem. 29, 935–941. doi: 10.1039/b418239a
Bain, G. A., and Berry, J. F. (2008). Diamagnetic corrections and Pascal's constants. J. Chem. Educ. 85, 532–536. doi: 10.1021/ed085p532
Barondeau, D. P., Kassmann, C. J., Bruns, C. K., Tainer, J. A., and Getzoff, E. D. (2004). Nickel superoxide dismutase structure and mechanism. Biochemistry 43, 8038–8047. doi: 10.1021/bi0496081
Becke, A. D. (1993). Density-functional thermochemistry. III. the role of exact exchange. J. Chem. Phys. 98, 5648–5652. doi: 10.1063/1.464913
Bernhardt, P. V., Bosch, S., Comba, P., Gahan, L. R., Hanson, G. R., Wadepohl, H., et al. (2015). An approach to more accurate model systems for purple acid phosphatases (PAPs). Inorg. Chem. 54, 7249–7263. doi: 10.1021/acs.inorgchem.5b00628
Bernhardt, P. V., Schenk, G., and Wilson, G. J. (2004). Direct electrochemistry of porcine purple acid phosphatase (Uteroferrin). Biochemistry 43:10387–10392. doi: 10.1021/bi0490338
Biswas, A., Das, L. K., Drew, M. G., Aromí, G., Gamez, P., and Ghosh, A. (2012). Synthesis, crystal structures, magnetic properties and catecholase activity of double phenoxido-bridged penta-coordinated dinuclear nickel(II) complexes derived from reduced schiff-base ligands: mechanistic inference of catecholase activity. Inorg. Chem. 51, 7993–8001. doi: 10.1021/ic202748m
Biswas, A., Drew, M. G. B., Gomez-Garcia, C. J., and Ghosh, A. (2017). Formation of a dinuclear and a trinuclear Ni(II) complex on slight variation of experimental conditions: Structural analysis and magnetic properties. Polyhedron 121, 80–87. doi: 10.1016/j.poly.2016.09.050
Blakeley, R. L., Treston, A., Andrews, R. K., and Zerner, B. (1982). Nickel(II)-promoted ethanolysis and hydrolysis of N-(2-pyridylmethyl)urea. a model for urease. J. Am. Chem. Soc. 104, 612–614. doi: 10.1021/ja00366a040
Blakeley, R. L., and Zerner, B. (1984). Jack bean urease: the first nickel enzyme. J. Mol. Catal. 23, 263–292. doi: 10.1016/0304-5102(84)80014-0
Bosch, S., Comba, P., Gahan, L. R., and Schenk, G. (2016). Asymmetric mono- and dinuclear GaIII and ZnII complexes as models for purple acid phosphatases. J. Inorg. Biochem. 162, 343–355. doi: 10.1016/j.jinorgbio.2015.12.028
Botana, L., Ruiz, J., Mota, A. J., Rodriguez-Dieguez, A., Seco, J. M., Colacio, E., et al. (2014). Anion controlled structural and magnetic diversity in unusual mixed-bridged polynuclear NiII complexes with a versatile bis(2-methoxy phenol)diamine hexadentate ligand. an experimental and theoretical magneto-structural study. Dalton Trans. 43, 13509–13524. doi: 10.1039/C4DT01253D
Bu, X.-H., Du, M., Zhang, L., Liao, D.-Z., Tang, J.-K., Shionoya, R.-M., et al. (2001). Novel nickel(II) complexes with diazamesocyclic ligands functionalized by additional phenol donor pendant(s): synthesis, characterization, crystal structures and magnetic properties. J. Chem. Soc. Dalton Trans. 593–598. doi: 10.1039/b008297j
Chattopadhyay, T., Mukherjee, M., Mondal, A., Maiti, P., Banerjee, A., Banu, K., et al. (2010). A unique nickel system having versatile catalytic activity of biological significance. Inorg. Chem. 49, 3121–3129. doi: 10.1021/ic901546t
Chilton, N. F., Anderson, R. P., Turner, L. D., Soncini, A., and Murray, K. S. (2013). PHI: a powerful new program for the analysis of anisotropic monomeric and exchange-coupled polynuclear d- and f-block complexes. J. Comput. Chem. 34, 1164–1175. doi: 10.1002/jcc.23234
Comba, P., Gahan, L. R., Hanson, G. R., Mereacre, V., Noble, C. J., Powell, A. K., et al. (2012a). Monoesterase activity of a purple acid phosphatase mimic with a cyclam platform. Chem. Eur. J. 18, 1700–1710. doi: 10.1002/chem.201100229
Comba, P., Gahan, L. R., Mereacre, V., Hanson, G. R., Powell, A. K., Schenk, G., et al. (2012b). Spectroscopic characterization of the active FeIIIFeIII and FeIIIFeII forms of a purple acid phosphatase model system. Inorg. Chem. 51, 12195–12209. doi: 10.1021/ic301347t
Comba, P., Hausberg, S., and Martin, B. (2009). Calculation of exchange coupling constants of transition metal complexes with DFT. J. Phys. Chem. A 113, 6751–6755. doi: 10.1021/jp900752p
Daumann, L. J., Comba, P., Larrabee, J. A., Schenk, G., Stranger, R., Gahan, L. R., et al. (2013a). Synthesis, magnetic properties, and phosphoesterase activity of dinuclear cobalt(II) complexes. Inorg. Chem. 52, 2029–2043. doi: 10.1021/ic302418x
Daumann, L. J., Larrabee, J. A., Comba, P., Schenk, G., and Gahan, L. R. (2013b). Dinuclear cobalt(II) complexes as metallo-β-lactamase mimics. Eur. J. Inorg. Chem. 3082–3089. doi: 10.1002/ejic.201300280
Deacon, G. B., and Phillips, R. J. (1980). Relationships between the carbon-oxygen stretching frequencies of carboxylato complexes and the type of carboxylate coordination. Coord. Chem. Rev. 33, 227–250. doi: 10.1016/S0010-8545(00)80455-5
Dixon, N. E., Gazzola, C., Asher, C. J., Lee, D. S., Blakeley, R. L., and Zerner, B. (1980). Jack been urease (EC 3.5.1.5). II. the relationship between nickel, enzymatic activity, and the “abnormal” ultraviolet spectrum. the nickel content of jack beans. Can. J. Biochem. 58, 474–480. doi: 10.1139/o80-063
Ensign, S. A., Bonam, D., and Ludden, P. W. (1989). Nickel is required for the transfer of electrons from carbon monoxide to the iron-sulfur center(s) of carbon monoxide dehydrogenase from Rhodospirillum rubrum. Biochemistry 28, 4968–4973. doi: 10.1021/bi00438a010
Ensign, S. A., Campbell, M. J., and Ludden, P. W. (1990). Activation of the nickel-deficient carbon monoxide dehydrogenase from Rhodospirillum rubrum: kinetic characterization and reductant requirement. Biochemistry 29, 2162–2168. doi: 10.1021/bi00460a029
Fabelo, O., Cañadillas-Delgado, L., Pasán, J., Delgado, F. S., Lloret, F., Ruiz-Perez, C., et al. (2009). Study of the influence of the bridge on the magnetic coupling in cobalt(II) complexes. Inorg. Chem. 48, 11342–11351. doi: 10.1021/ic901843r
Farrugia, L. J. (1999). WinGX suite for small-molecule single-crystal crystallography. J. Appl. Crystallogr. 32, 837–838. doi: 10.1107/S0021889899006020
Flanagan, J. U., Cassady, A. I., Schenk, G., Guddat, L. W., and Hume, D. A. (2006). Identification and molecular modelling of a novel, plant-like, human purple acid phosphatase. Gene 377, 12–20. doi: 10.1016/j.gene.2006.02.031
Frisch, M. J., Trucks, G. W., Schlegel, H. B., Scuseria, G. E., Robb, M. A., Fox, D. J., et al. (2013). Gaussian 09, Revision D.01. Wallingford CT: Gaussian, Inc.
Gencic, S., and Grahame, D. A. (2003). Nickel in subunit beta of the acetyl-CoA decarbonylase/synthase multienzyme complex in methanogens. catalytic properties and evidence for a binuclear Ni-Ni site. J. Biol. Chem. 278, 6101–6110. doi: 10.1074/jbc.M210484200
Greatti, A., Scarpellini, M., Peralta, R. A., Casellato, A., Bortoluzzi, A. J., Xavier, F. R., et al. (2008). Synthesis, structure, and physicochemical properties of dinuclear NiII complexes as highly efficient functional models of phosphohydrolases. Inorg. Chem. 47, 1107–1119. doi: 10.1021/ic702132t
Greatti, A. M., Aires de, Brito, Bortoluzzi, A. J., and Ceccato, A. S. (2004). Synthesis, characterization and structure of a new dinickel(II) complex as model for urease. J. Mol. Struct. 688, 185–190. doi: 10.1016/j.molstruc.2003.10.008
Halcrow, M. A., and Christou, G. (1994). Biomimetic chemistry of nickel. Chem. Rev. 94, 2421–2481. doi: 10.1021/cr00032a008
Hempel, J. C., and Miller, M. E. (1981). Ground state properties of trigonal prismatic and trigonal bipyramidal d1,9, d2,8, d3,7, and d5 systems: effects of trigonal distortion. J. Chem. Phys. 75, 2959–2970. doi: 10.1063/1.442387
Hossain, M. J., and Sakiyama, H. (2002). Two typical cases of magnetism for dinuclear high-spin cobalt(II) complexes in trigonal-bipyramidal fields. Inorg. Chim. Acta 338, 255–259. doi: 10.1016/S0020-1693(02)01053-8
Jikido, R., Shiraishi, H., Matsufuji, K., Ohba, M., Furutachi, H., Suzuki, M., et al. (2005). Mass spectrometric and spectroscopic studies on hydrolysis of phosphoesters by bis(μ-acetato)-μ-phenolato dinuclear metal(II) complexes (metal = Mn, Co, Ni, and Zn). Bull. Chem. Soc. Jpn. 78, 1795–1803. doi: 10.1246/bcsj.78.1795
Johansson, F. B., Bond, A. D., Nielsen, U. G., Moubaraki, B., Murray, K., McKenzie, C. J., et al. (2008). Dicobalt II-II, II-III, and III-III complexes as spectroscopic models for dicobalt enzyme active sites. Inorg. Chem. 47, 5079–5092. doi: 10.1021/ic7020534
Jung, M., Sharma, A., Hinderberger, D., Braun, S., Schatzschneider, U., and Rentschler, E. (2009). Nitronyl nitroxide radicals linked to exchange-coupled metal dimers - studies using X-ray crystallography, magnetic susceptibility measurements, EPR spectroscopy, and DFT calculations. Eur. J. Inorg. Chem. 1495–1502. doi: 10.1002/ejic.200801248
Kaminskaia, N. V., Spingler, B., and Lippard, S.J (2000). Hydrolysis of β-lactam antibiotics catalyzed by dinuclear zinc(II) complexes: functional mimics of metallo-β-lactamases. J. Am. Chem. Soc. 122, 6411–6422. doi: 10.1021/ja993704l
Kantacha, A., Buchholz, R., Smith, S. J., Schenk, G., and Gahan, L. R. (2011). Phosphate ester cleavage promoted by a tetrameric iron(III) complex. J. Biol. Inorg. Chem. 16, 25–32. doi: 10.1007/s00775-010-0696-0
Khandar, A. A., Kirschbaum, K., Abedi, M., Mock, K., Tracy, G., Spasojevic, V., et al. (2015). Synthesis, characterization and crystal structures of mono and dinuclear macrocyclic cobalt(II) complexes with a new tetraaza m-xylyl-based macrocyclic ligand. New J. Chem. 39, 2822–2831. doi: 10.1039/C4NJ01608D
Krupskaya, Y., Alfonsov, A., Parameswaran, A., Kataev, V., Klingeler, R., Steinfeld, G., et al. (2010). Interplay of magnetic exchange interactions and Ni-S-Ni bond angles in polynuclear nickel(II) complexes. ChemPhysChem 11, 1961–1970. doi: 10.1002/cphc.200900935
Li, J.-L., Jiang, L., Wang, B.-W., Tian, J.-L., Gu, W., Yan, P., et al. (2015). Bio-relevant cobalt(II) complexes with compartmental polyquinoline ligand: synthesis, crystal structures and biological activities. J. Inorg. Biochem. 145, 19–29. doi: 10.1016/j.jinorgbio.2014.11.003
Mahapatra, P., Ghosh, S., Giri, S., and Ghosh, A. (2016). The unusual intermediate species in the formation of Ni(II) complexes of unsymmetrical Schiff bases by Elder's method: structural, electrochemical and magnetic characterizations. Polyhedron 117, 427–436. doi: 10.1016/j.poly.2016.06.020
Mandal, S., Balamurugan, V., Lloret, F., and Mukherjee, R. (2009). Syntheses, X-ray structures, and physicochemical properties of phenoxo-bridged dinuclear nickel(II) complexes: kinetics of transesterification of 2-hydroxypropyl-p-nitrophenyl phosphate. Inorg. Chem. 48, 7544–7556. doi: 10.1021/ic8018937
Massoud, S. S., Broussard, K. T., Mautner, F. A., Vicente, R., Saha, M. K., and Bernal, I. (2008). Five-coordinate cobalt(II) complexes of tris(2-pyridylmethyl)amine (TPA): synthesis, structural and magnetic characterization of a terephthalato-bridged dinuclear cobalt(II) complex. Inorg. Chim. Acta 361, 123–131. doi: 10.1016/j.ica.2007.06.021
Massoud, S. S., Ledet, C. C., Junk, T., Bosch, S., Comba, P., Mautner, F. A., et al. (2016). Dinuclear metal(II)-acetato complexes based on bicompartmental 4-chlorophenolate: syntheses, structures, magnetic properties, DNA interactions and phosphodiester hydrolysis. Dalton Trans. 45, 12933–12950. doi: 10.1039/C6DT02596J
Mendes, L. L., Englert, D., Fernandes, C., Gahan, L. R., Schenk, G., and Horn, A. (2016). Metallohydrolase biomimetics with catalytic and structural flexibility. Dalton Trans. 45, 18510–18521. doi: 10.1039/C6DT03200A
Meyer, F. (2009). Synthetic models for the urease active site. Prog. Inorg. Chem. 56, 487–542. doi: 10.1002/9780470440124.ch6
Mitić, N, Hadler, K. S., Gahan, L. R., Hengge, A. C., and Schenk, G. (2010). The divalent metal ion in the active site of uteroferrin modulates substrate binding and catalysis. J. Am. Chem. Soc. 132, 7049–7054. doi: 10.1021/ja910583y
Mitić, N, Noble, C. J., Gahan, L. R., Hanson, G. R., and Schenk, G. (2009). Metal ion mutagenesis – conversion of a purple acid phosphatase from sweet potato to a neutral phosphatase with the formation of an unprecedented catalytically competent MnIIMnII active site. J. Am. Chem. Soc. 131, 8173–8179. doi: 10.1021/ja900797u
Mochizuki, K., Hasegawa, A., and Weyhermuller, T. (2004). Synthesis, structures, and magnetic properties of dicopper(II) and dinickel(II) complexes with a new bis(macrocycle), 7,7'-(2-hydroxypropane-1,3-diyl)bis{3,7,11,17-tetraazabicyclo[11.3.1]- heptadeca-1(17),13,15-triene}. Inorg. Chim. Acta 357, 3245–3250. doi: 10.1016/j.ica.2004.01.047
Moffat, C. D., Weiss, D. J., Shivalingam, A., White, A. J., Salaün, P., and Vilar, R. (2014). Molecular recognition and scavenging of arsenate from aqueous solution using dimetallic receptors. Chem. Eur. J. 20, 17168–17177. doi: 10.1002/chem.201404723
Nanda, K. K., Das, R., Thompson, L., Venkatsubramanian, K., Paul, P., and Nag, K. (1994b). Magneto-structural correlations in macrocyclic dinickel(II) complexes: tuning of spin exchange by varying stereochemistry and auxiliary ligands. Inorg. Chem. 33, 1188–1193. doi: 10.1021/ic00084a036
Nanda, K. K., Das, R., Thompson, L. K., Venkatsubramanian, K., and Nag, K. (1994a). Combined Effect of phenoxy and carboxylate bridges on magnetic properties of a series of macrocyclic dinickel(II) complexes. Inorg. Chem. 33, 5934–5939. doi: 10.1021/ic00103a048
Nanda, K. K., Thompson, L., Bridson, J., and Nag, K. (1994c). Linear dependence of spin exchange coupling constant on bridge angle in phenoxy-bridged dinickel(II) complexes. J. Chem. Soc. Chem. Commun. 1337–1338. doi: 10.1039/c39940001337
Neese, F. (2012). The ORCA program system. wiley Interdiscip. Rev. Comput. Mol. Sci. 2, 73–78. doi: 10.1002/wcms.81
Noodleman, L., Case, D. A., and Aizman, A. (1988). Broken symmetry analysis of spin coupling in iron-sulfur clusters. J. Am. Chem. Soc. 110, 1001–1005. doi: 10.1021/ja00212a003
Parimala, S., and Kandaswamy, M. (2003). Mononuclear, dinuclear nickel(II) and heterodinuclear Zn(II)Ni(II) complexes as models for the active site of phosphatase. Inorg. Chem. Commun. 6, 1252–1254. doi: 10.1016/S1387-7003(03)00243-0
Pawlak, P. L., Malkhasian, A. Y. S., Sjlivic, B., Tiza, M. J., Kucera, B. E., Chavez, F. A., et al. (2008). Synthesis, structure, and magnetic properties of a new binuclear Ni(II) complex supported by 1,4,8-triazacycloundecane. Inorg. Chem. Commun. 11, 1023–1026. doi: 10.1016/j.inoche.2008.05.014
Piovezan, C., Silva, J. M. R., Neves, A., Bortoluzzi, A. J., Haase, W., Rossi, L. M., et al. (2012). Design of a dinuclear nickel(II) bioinspired hydrolase to bind covalently to silica surfaces: synthesis, magnetism, and reactivity studies. Inorg. Chem. 51, 6104–6115. doi: 10.1021/ic300018t
Prushan, M. J., Tomezsko, D. M., Lofland, S., Zeller, M., and Hunter, A. D. (2007). A nickel(II) di-μ2-phenolato bridged dinuclear complex: weak antiferromagnetic interactions in nickel(II) dimers. Inorg. Chim. Acta 360, 2245–2254. doi: 10.1016/j.ica.2006.11.008
Przybyla, A. E., Robbins, J., Menon, N., and Peck, H. D. Jr (1992). Structure-function relationships among the nickel-containing hydrogenases. FEMS Microbiol. Rev. 8, 109–135. doi: 10.1111/j.1574-6968.1992.tb04960.x
Ren, Y.-W., Lu, J.-X., Cai, B.-W., Shi, D.-B., Jiang, H.-F., Chen, J., et al. (2011). A novel asymmetric di-Ni(II) system as a highly efficient functional model for phosphodiesterase: synthesis, structures, physicochemical properties and catalytic kinetics. Dalton Trans. 40, 1372–1381. doi: 10.1039/C0DT01194K
Roberts, A. E., Schenk, G., and Gahan, L. R. (2015). A heterodinuclear FeIIIZnII complex as a mimic for purple acid phosphatase with site-specific ZnII binding. Eur. J. Inorg. Chem. 2015, 3076–3086. doi: 10.1002/ejic.201500351
Sanyal, R., Zhang, X., Chakraborty, P., Giri, S., Chattopadhyay, S. K., Das, D., et al. (2016). Role of solvent in the phosphatase activity of a dinuclear nickel(II) complex of a Schiff base ligand: mechanistic interpretation by DFT studies. New J. Chem. 40, 7388–7398. doi: 10.1039/C6NJ01043A
Sargent, F. (2016). The Model [NiFe]-hydrogenases of escherichia coli. Adv. Microb. Physiol. 68, 433–507. doi: 10.1016/bs.ampbs.2016.02.008
Sato, M., Mori, Y., and Iida, T. (1992). Convenient synthesis of N,N,N',N'-tetrakis(2-pyridylmethyl)-α,ω-alkanediamines using a phase-transfer catalyst. Synthesis 539–540. doi: 10.1055/s-1992-26157
Schaefer, A., Horn, H., and Ahlrichs, R. (1992). Fully optimized contracted Gaussian basis sets for atoms lithium to krypton. J. Chem. Phys. 97, 2571–2577. doi: 10.1063/1.463096
Schenk, G., Mitić, N., Hanson, G. R., and Comba, P. (2013). Purple acid phosphatase: a journey into the function and mechanism of a colourful enzyme. Coord. Chem. Rev. 257, 473–482. doi: 10.1016/j.ccr.2012.03.020
Schenk, G., Peralta, R. A., Batista, S. C., Bortoluzzi, A. J., Szpoganicz, B., Neves, A., et al. (2008). Probing the role of the divalent metal ion in uteroferrin using metal ion replacement and a comparison to isostructural biomimetics. J. Biol. Inorg. Chem. 13, 139–155. doi: 10.1007/s00775-007-0305-z
Schultz, B. E., Ye, B.-H., Li, X.-Y., and Chan, S. I. (1997). Electronic paramagnetic resonance and magnetic properties of model complexes for binuclear active sites in hydrolase enzymes. Inorg. Chem. 36, 2617–2622. doi: 10.1021/ic960988r
Selleck, C., Clayton, D., Gahan, L. R., Mitić, N., McGeary, R. P., Schenk, G., et al. (2017). Visualization of the reaction trajectory and transition state in a hydrolytic reaction catalyzed by a metalloenzyme. Chem. Eur. J. 23, 4778–4781. doi: 10.1002/chem.201700866
Shaw, W. H. R. (1954). Inhibition of urease by various metal ions. J. Am. Chem. Soc. 76, 2160–2163. doi: 10.1021/ja01637a034
Shaw, W. H. R., and Raval, D. N. (1961). The inhibition of urease by metal ions at pH 8.9. J. Am. Chem. Soc. 83, 3184–3187. doi: 10.1021/ja01476a004
Sheldrick, G. M. (1997). SHELXL97: Program for the Refinement of Crystal Structures. Germany, University of Gottingen.
Shin, W., and Lindahl, P. A. (1992). Discovery of a labile nickel ion required for CO/acetyl-CoA exchange activity in the NiFe complex of carbon monoxide dehydrogenase from Clostridium thermoaceticum. J. Am. Chem. Soc. 114, 9718–9719. doi: 10.1021/ja00050a094
Sibille, J. C., Doi, K., and Aisen, P. (1987). Hydroxyl radical formation and iron-binding proteins. stimulation by the purple acid phosphatases. J. Biol. Chem. 262, 59–62.
Siluvai, G. S., and Murthy, N. N. (2009). X-ray structure and spectroscopic characterization of divalent dinuclear cobalt complexes containing carboxylate- and phosphodiester- auxiliary bridges. Inorg. Chim. Acta 362, 3119–3126. doi: 10.1016/j.ica.2009.02.010
Smith, S. J., Casellato, A., Hadler, K. S., Mitić, N., Riley, M. J., Gahan, L. R., et al. (2007). The reaction mechanism of the Ga(III)Zn(II) derivative of uteroferrin and corresponding biomimetics. J. Biol. Inorg. Chem. 12, 1207–1220. doi: 10.1007/s00775-007-0286-y
Smith, S. J., Riley, M. J., Noble, C. J., Hanson, G. R., Stranger, R., Gahan, L. R., et al. (2009). Structural and catalytic characterization of a heterovalent Mn(II)Mn(III) complex that mimics purple acid phosphatases. Inorg. Chem. 48, 10036–10048. doi: 10.1021/ic9005086
Spears, J. W., Smith, C. J., and Hatfield, E. E. (1977). Rumen bacterial urease requirement for nickel. J. Dairy Sci. 60, 1073–1076. doi: 10.3168/jds.S0022-0302(77)83990-8
Suzuki, M., Sugisawa, T., Senda, H., Oshio, H., and Uehara, A. (1989). Synthesis and characterization of a novel tetranuclear manganese(II,III,III,II) mixed valence complex. Chem. Lett. 1091–1094. doi: 10.1246/cl.1989.1091
Suzuki, M., Sugisawa, T., and Uehara, A. (1990). Dinuclear cobalt(II) complexes containing 1,3-(or 1,5-)bis[bis-(2-pyridylmethyl)amino]-2-propanolato (or−3-pentanolato): preparation and reaction with molecular oxygen. Bull. Chem. Soc. Jpn. 63, 1115–1120. doi: 10.1246/bcsj.63.1115
Tian, J.-L., Feng, L., Gu, W., Xu, G.-J., Yan, S.-P., Cheng, D.-P., et al. (2007). Synthesis, crystal structure, magnetic property and nuclease activity of a new binuclear cobalt(II) complex. J. Inorg. Biochem. 101, 196–202. doi: 10.1016/j.jinorgbio.2006.08.009
Tian, J.-L., Gu, W., Yan, S.-P., Liao, D.-Z., and Jiang, Z.-H. (2008). Synthesis, structures and magnetic properties of two binuclear cobalt(II) complexes bridged by bis(p-nitrophenyl) phosphate or diphenylphosphinate ion. Z. Anorgan. Allgem. Chem. 634, 1775–1779. doi: 10.1002/zaac.200800130
Tomkowicz, Z., Ostrovsky, S., Foro, S., Calvo-Perez, V., and Haase, W. (2012). Magnetooptical and structural investigations of five dimeric cobalt(II) complexes mimicking metalloenzyme active sites. Inorg. Chem. 51, 6046–6055. doi: 10.1021/ic202529p
Tudor, V., Marin, G., Lloret, F., Kravtsov, V. C., Simonov, Y. A., Andruh, M., et al. (2008). New alkoxo-bridged mixed-valence cobalt clusters: synthesis, crystal structures and magnetic properties. Inorg. Chim. Acta 361, 3446–3452. doi: 10.1016/j.ica.2008.03.007
Vaissier, V., and Van, V. T. (2017). Quantum chemical approaches to [NiFe] hydrogenase. Essays Biochem 61, 293–303. doi: 10.1042/EBC20160079
Vichard, C., and Kaden, T. A. (2002). Phosphate and phosphonate ester hydrolysis promoted by dinuclear metal complexes. Inorg. Chim. Acta 337, 173–180. doi: 10.1016/S0020-1693(02)00997-0
Vichard, C., and Kaden, T. A. (2004). Phosphate diester hydrolysis in biological relevant compounds by dinuclear metal complexes. Inorg. Chim. Acta 357, 2285–2293. doi: 10.1016/j.ica.2003.12.041
Wages, H. E., Taft, K. L., and Lippard, S. J. (1993). Tris(acetato)bis(N,N,N',N'-tetramethylethylenediamine)(urea)dinickel(1+) triflate and [Ni(OAc)(urea)2(tmen)](OTf), model complexes for the enzyme urease. Inorg. Chem. 32, 4985–4987. doi: 10.1021/ic00075a001
Wu, A.-Z., and Wang, T. (2014). Synthesis, crystal structures and phosphodiesterase activities of alkoxide-bridged asymmetric dinuclear nickel(II) complexes. Trans. Met. Chem. 39, 205–211. doi: 10.1007/s11243-013-9791-8
Xavier, F. R., and Neves, A. (2016). Synthesis, physicochemical properties and in vitro catalytic activity of a dinuclear nickel(II) complex with a N5O-hexadentate ligand: a functional model for phosphohydrolases. Polyhedron 109, 59–66. doi: 10.1016/j.poly.2016.02.013
Yamaguchi, K., Akagi, F., Fujinami, S., Suzuki, M., Shionoya, M., and Suzuki, S. (2001). Hydrolysis of phosphodiester with hydroxo- or carboxylate-bridged dinuclear Ni(II) and Cu(II) complexes. J. Chem. Soc. Chem. Commun. 375–376. doi: 10.1039/b008994j
Yamaguchi, K., Koshino, S., Akagi, F., Suzuki, M., Uehara, A., and Suzuki, S. (1997). Structures and catalytic activities of carboxylate-bridged dinickel(II) complexes as models for the metal center of urease. J. Am. Chem. Soc. 119, 5752–5753. doi: 10.1021/ja963982+
Yamane, H., Hosokawa, Y., Nakao, Y., Matsumoto, K., Takamizawa, S., Mori, W., et al. (1997). Dinuclear nickel(II) complexes as models for the active site of urease. J. Inorg. Biochem. 67:186. doi: 10.1016/S0162-0134(97)80063-3
Yamane, H., Nakao, Y., Kawabe, S., Xie, Y., Kanehisa, N., Kai, Y., et al. (2001). Synthesis, structures and characterization of the dinuclear nickel(II) complexes containing N,N,N',N'-tetrakis[(1-ethyl-2-benzimidazolyl)methyl]-2-hydroxy-1,3-diaminopropane and their urea complexes relevant to the urease active site. Bull. Chem. Soc. Jpn. 74, 2107–2112. doi: 10.1246/bcsj.74.2107
Zambelli, B., Musiani, F., Benini, S., and Ciurli, S. (2011). Chemistry of Ni2+ in Urease: Sensing, Trafficking, and Catalysis. Acc. Chem. Res. 44, 520–530. doi: 10.1021/ar200041k
Keywords: nickel, phosphoesterase, magnetism, DFT, kinetics, mechanism
Citation: Horn A Jr, Englert D, Roberts AE, Comba P, Schenk G, Krenske EH and Gahan LR (2018) Synthesis, Magnetic Properties, and Catalytic Properties of a Nickel(II)-Dependent Biomimetic of Metallohydrolases. Front. Chem. 6:441. doi: 10.3389/fchem.2018.00441
Received: 24 May 2018; Accepted: 05 September 2018;
Published: 25 September 2018.
Edited by:
Federico Cesano, Università degli Studi di Torino, ItalyReviewed by:
Jeffrey Bos, Princeton University, United StatesAkira Odani, Kanazawa University, Japan
Salah S. Massoud, University of Louisiana at Lafayette, United States
Copyright © 2018 Horn, Englert, Roberts, Comba, Schenk, Krenske and Gahan. This is an open-access article distributed under the terms of the Creative Commons Attribution License (CC BY). The use, distribution or reproduction in other forums is permitted, provided the original author(s) and the copyright owner(s) are credited and that the original publication in this journal is cited, in accordance with accepted academic practice. No use, distribution or reproduction is permitted which does not comply with these terms.
*Correspondence: Adolfo Horn Jr., adolfo@uenf.br
Lawrence R. Gahan, gahan@uq.edu.au
†Present Address: Gerhard Schenk, Australian Centre for Ecogenomics, The University of Queensland, Brisbane, QLD, Australia