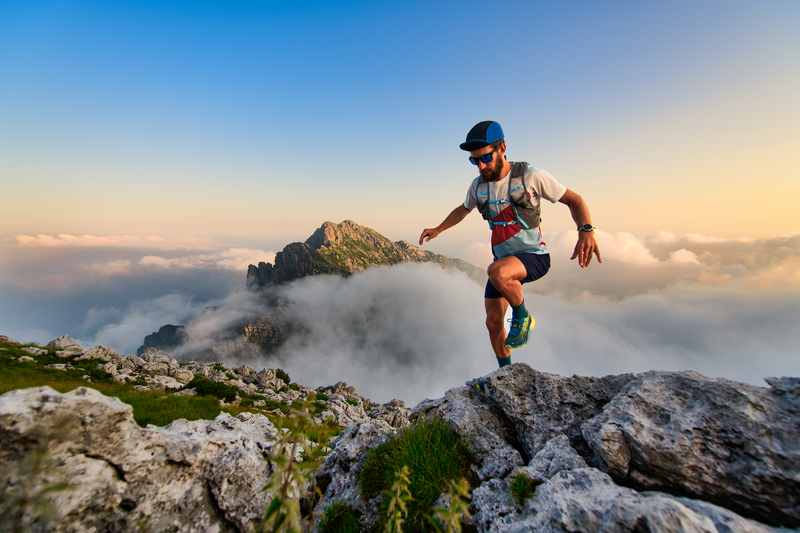
95% of researchers rate our articles as excellent or good
Learn more about the work of our research integrity team to safeguard the quality of each article we publish.
Find out more
ORIGINAL RESEARCH article
Front. Chem. , 19 September 2018
Sec. Nanoscience
Volume 6 - 2018 | https://doi.org/10.3389/fchem.2018.00396
This article is part of the Research Topic Nonferrous Nanomaterials & Composites for Energy Storage and Conversion View all 48 articles
NaTi2(PO4)3 has attracted great interest as anode material for sodium ion batteries owing to its open three-dimensional framework structure and limited volume changes during the charge and discharge process. However, the poor intrinsic electronic conductivity of NaTi2(PO4)3 needs to be improved for high rate capability. In this work, porous NaTi2(PO4)3 nanocubes anchored on porous carbon nanosheets (NaTi2(PO4)3/C) are designed and developed. This material exhibits a large discharge capacity and good rate capacity including a first discharge capacity of 485 mAh g−1 at a current density of 0.1 A g−1, and 98 mAh g−1 retained at a high rate of 4 A g−1 even after 2,000 cycles. These results suggest that NaTi2(PO4)3/C is a promising anode material for sodium-ion batteries.
Sodium-ion batteries (SIBs), as an alternative energy storage system for lithium-ion batteries (LIBs), have attracted increasing attention due to their low cost and the abundant resource of sodium (Gao et al., 2017; Cui et al., 2018; Liang et al., 2018c). The electrochemical performance of SIBs is closely related to the properties of electrode materials, especially anode materials (Chen et al., 2018; Fan et al., 2018; Hu A. J. et al., 2018; Liang et al., 2018b,d; Wan et al., 2018; Wei et al., 2018). Recently, Na super ion conductor (NASICON) type NaTi2(PO4)3 has been considered as one of promising anode materials for SIBs owing to its “zero-stress” three-dimensional (3D) framework, high Na+ conductivity, and good thermal stability (Kabbour et al., 2011; Wu et al., 2013; Sun et al., 2016; Ye et al., 2017).
However, the poor intrinsic electrical conductivity of NTP leads to poor rate capability (Pang et al., 2014b; Roh et al., 2017). To improve the Na+ ions insertion-extraction kinetics, two common approaches used include synthesis of various nanostructures and fabrication of carbon composites. Morphology control of NaTi2(PO4)3 has been applied to realizeexcellent electrochemical performance. Different nanostructures such as hollow nanocubes, nanoparticles, and hierarchical microspheres have been demonstrated (Wu et al., 2015; Fang et al., 2016; Ye et al., 2017). Among them, porous structures have gained great attention owing to the afforded large surface areas and improved kinetics (Dirican et al., 2015; Zhang et al., 2017; Zhao et al., 2017; Zhou et al., 2017). Moreover, the electronic conductivity of anodes can be largely enhanced by hybridizing them with conductive materials. For example, the coating of carbon or graphene on NaTi2(PO4)3 micro/nanostructures can effectively improve their properties and higher quality of the conductive materials could result in better electrochemical performance. Nevertheless, the contents of carbon or graphene in the previously reported composites were only 3.4–6.8 wt% (Pang et al., 2014b; Fang et al., 2016; Geng et al., 2017; Hu Q. et al., 2018; Liang et al., 2018a). Thus, to obtain better electrochemical properties, the contents of conduction materials should be increased. It has been found that the embedding of anode materials in carbon/graphene matrixes can realize high content of conductive carbon materials for enhanced electrochemical properties (Fu et al., 2015; Guo et al., 2015; Choi et al., 2016; Sun et al., 2016). Motivated by the above potentials, we have prepared porous NaTi2(PO4)3 nanocubes anchored on porous carbon nanosheets (NaTi2(PO4)3/C) through ultrasonic treatment. To the best of our knowledge, it is the first report that NaTi2(PO4)3 nanocubes with porous structures have been embedded in a porous carbon matrix. This NaTi2(PO4)3/C material exhibited a high discharge capacity, good rate performance, and excellent long-time cycling stability.
The synthesis of porous carbon nanosheets was firstly conducted from the uniform mixture of Zn(CH3COO)2·2H2O (5 g) and oleic acid (5 g) in an agate mortar for 30 min. Then the above mixture was transferred into a tubular furnace and calcined at 700°C for 2 h with a ramping rate of 2°C min−1 in Ar atmosphere to form ZnO/C slices. The ZnO/C slices were washed using 6 mol L−1 aqueous HCl solution to form porous carbon nanosheets. These carbon nanosheets were washed by deionized water and absolute ethanol, then dried in vacuum at 50°C for 8 h. Similar process was used to prepare the MnO/graphene composite using oleic acid as carbon sources (Guo et al., 2015).
The synthesis of NaTi2(PO4)3 nanocubes was conducted following the reported procedures (Wu et al., 2015). Briefly, sodium acetate (0.16 g) was added in a mixed solvent glacial acetic acid (0.7 mL), phosphoric acid (4 ml) and ethylene glycol (25 ml), followed by the addition of tetrabutyl titanate (1.36 g). The resultant mixture was heated at 180°C for 12 h. Finally, the white precipitate NaTi2(PO4)3 was obtained.
For synthesizing NaTi2(PO4)3/C, 0.16 g precursor NaTi2(PO4)3, 0.04 g porous carbon nanosheets, and 3.6 g cetyltrimethylammonium bromide (CTAB) were added into 30 mL absolute ethanol. After being stirred for 2 h and ultrasonically dispersed for 2 h, the precursor NaTi2(PO4)3/C was collected by centrifugation, washed with deionized water and anhydrous ethanol, and dried at 60°C for 12 h. Subsequently, the precursor NaTi2(PO4)3/C was further calcined at 700°C for 2 h with a ramping rate of 2°C min−1 in Ar atmosphere to form NaTi2(PO4)3/C. For comparison, porous NaTi2(PO4)3 cubes were prepared after annealing without the porous carbon nanosheets.
Rigaku D/max-2500 X-ray diffractometer (Cu Kα, λ = 1.54056 Å) was used to investigate the crystal structures. The morphology and nanostructure were observed by Hitachi S4800 scanning electron microscopy and JEOL 2010 transmission electron microscopy. The Brunauer-Emmett-Teller special (BET) surface area and pore size were tested at 77 K on a Nova 2000e volumetric adsorption analyzer. The thermogravimetric (TG) analysis was performed with a WCT-1D instrument over a range of 30–800°C at a heating rate of 10°C·min−1 in air atmosphere.
Active materials, acetylene black and carboxymethylcellulose sodium with a weight ratio of 80:10:10 were uniformly mixed, and the obtained slurry was coated on Cu foil. Then, the electrodes were assembled into CR2025 coin cell in the glove box. The glass microfiber filter membrane (Whatman, grade GF/A) was used as the separator. Metallic sodium film was used as counter/reference electrodes. The electrolyte was 1 mol L−1 NaClO4 dissolved in a mixture of ethylene carbonate and diethyl carbonate (1:1 vol%) with 5 wt% fluoroethylene carbonate. Galvanostatic tests were evaluated by Neware Battery Testing System. Cyclic voltammetry (CV) tests and impedance measurement were carried out on a CHI660C Electrochemical Workstation.
The phase of NaTi2(PO4)3 and NaTi2(PO4)3/C were confirmed by XRD, as shown in Figure 1A. All diffraction peaks are in accordance with the standard pattern of NaTi2(PO4)3 (JCPDS No. 84-2008). No peaks for impurities can be detected, suggesting the high purity of these two samples. Moreover, the diffraction peaks of carbon material is not clearly discerned due to the sharp and strong diffraction of NaTi2(PO4)3, implying the high crystalline nature. In addition, according to the TGA curve of NaTi2(PO4)3 (Figure 1B), the relative weight fraction of carbon for NaTi2(PO4)3/C was determined to be ~18.3%.
The morphology of porous carbon nanosheets, NaTi2(PO4)3, and NaTi2(PO4)3/C was characterized by SEM. Figure 2A shows the low-magnified SEM image of porous carbon nanosheets. It is clear that the sample is exclusively nanosheets with irregular morphologies. The high-resolution SEM image (Figure 2B) shows that the carbon nanosheets are curved and have an average thickness of ~10 nm. SEM images of NaTi2(PO4)3 (Figures 2C,D) show that the products have uniform cubic shapes and their sizes are in the range between 50 and 100 nm, similar with the results reported in literatures (Liang et al., 2018a).
Figure 2. (A) SEM image and (B) high-resolution SEM image of porous carbon nanosheets; (C) SEM image and (D) high-magnification SEM image of NaTi2(PO4)3.
It was observed that the NaTi2(PO4)3 were uniformly anchored on the porous carbon nanosheets for NaTi2(PO4)3/C sample (Figures 3A,B). The detailed structural characteristics of NaTi2(PO4)3/C was further investigated by TEM. The TEM image in Figure 3C illustrates that NaTi2(PO4)3 in a size range of 50 and 100 nm were scattered over the carbon nanosheets. This is consistent with the SEM results in Figure 3B. Notably, both nanocubes and carbon nanosheets have obvious porous structure, which is beneficial for the transport of Na+ (Wu et al., 2015). Additionally, the HR-TEM image of a representative nanocube (Figure 3D) implies that the interplanar spacing is ca. 0.365 nm, in good agreement with the (113) plane of NASICON-type phase (Ye et al., 2017).
Figure 3. (A) SEM image, (B) high-resolution SEM image, and (C) TEM image of NaTi2(PO4)3/C; (D) HR-TEM images of a typical NaTi2(PO4)3 particle anchored on carbon nanosheets.
BET analysis was performed to study the pore size and specific surface area of NaTi2(PO4)3/C. The Nitrogen adsorption-desorption isotherm of NaTi2(PO4)3/C (Figure 4A) reveals a type-IV isotherm with an obvious H1-type hysteretic loop in the range of 0.4–1.0 (P/P0), indicating that the products possess porous structures (Takashima et al., 2015). The BET analysis indicates that the specific surface area of NaTi2(PO4)3/C was ca. 103.1 m2 g−1. Moreover, as shown in Figure 4B, the sample possessed a broad pore-size distribution and the pore-size distribution maximum was centered at 15.4 nm. The large surface area and porous structure of NaTi2(PO4)3/C is beneficial to improve the sodium-ion storage properties (Wang H. et al., 2016; Wang G. et al., 2018).
Figure 4. (A) N2 adsorption-desorption isotherm and (B) pore-size distribution curve of NaTi2(PO4)3/C.
The electrochemical properties of NaTi2(PO4)3/C were studied as anode material for SIBs. The cyclic voltammogram (CV) of NaTi2(PO4)3/C at a scan rate of 0.1 mV s−1 was analyzed to investigate their redox kinetic properties. In Figure 5A, at the 1st cycle, a pair of redox peaks at 1.97/2.29 V can be attributed to conversion reaction of Ti4+/Ti3+ (Pang et al., 2014a; Fang et al., 2016; Ye et al., 2017). Moreover, another pair of cathodic/anodic peaks located at 0.27/0.57 V can be attributed to the redox reaction between Ti3+ and Ti2+ (Senguttuvan et al., 2013; Wang D. et al., 2016). That is, Ti4+ in the reduction process was firstly reduced to Ti3+ (NaTi2(PO4)3 + 2Na+ + 2e− → Na3Ti2(PO4)3) and then formed into Ti2+ (Na3Ti2(PO4)3+ Na+ + e− → Na4Ti2(PO4)3). In the following cycles, the anodic peaks shift to higher potentials (1.97 vs. 2.09 V; 0.27 vs. 0.30 V), which was probably caused by the stress/strain change, similar to other NASICON-type anodic materials (Li et al., 2014). More importantly, in the following 2nd, 3rd, and 5th cycles, two pairs of reduction/oxidation peaks almost remained unchanged, indicating the excellent reversibility.
Figure 5. (A) Cyclic voltammogram curves of NaTi2(PO4)3/C for the initial five cycles in the voltage range of 0.01–3.0 V (vs. Na+/Na); (B) discharge-charge curves of NaTi2(PO4)3/C at a current density of 0.1 A g−1; (C) cycling performances of NaTi2(PO4)3/C and NaTi2(PO4)3 at 0.1 A g−1; (D) rate capacity of NaTi2(PO4)3/C; (E) long-term cycling performance of NaTi2(PO4)3/C at a high rate of 4 A g−1.
Figure 5B showed the galvanostatic discharge-charge curves of NaTi2(PO4)3/C electrode in the voltage window between 0.01 and 3.0 V. The initial discharge capacity was 485 mAh g−1, which was higher than the theoretical capacity (133 mAh g−1). However, the initial charge capacity was 227 mAh g−1 with an unsatisfied Coulombic efficiency of 46.8%. Such a large capacity loss is mostly ascribed to the formation of solid electrolyte interface (SEI) layers for the existence of carbon substrates, as well as the decomposition of electrolyte (Hasegawa et al., 2016; Wang D. et al., 2016). On the contrary, the first discharge capacity of the NaTi2(PO4)3 electrode was only 229 mAh g−1 (Figure S1, Supporting Information). In the subsequent cycles, the NaTi2(PO4)3/C electrode possessed good cycle stability and excellent reversibility for Na+ ion insertion and extraction. For example, at the 5th and 10th cycles, the discharge capacity retained to be 221 and 203 mAh g−1 with the coulombic efficiency of 94 and 96%, respectively. Figure 5C displayed the cycling behavior of the NaTi2(PO4)3/C and NaTi2(PO4)3 electrodes at a current density of 0.1 A g−1. It can be seen thatafter 100 cycle NaTi2(PO4)3/C still delivered a discharge capacity of 172 mAh g−1, which was much larger than that of NaTi2(PO4)3 (20 mAh g−1). Accordingly, the NaTi2(PO4)3/C electrode exhibited a capacity retention of 69% (relative to the 2nd cycle), higher than that of NaTi2(PO4)3 (30%). In addition, the long-term cycling performance for the NaTi2(PO4)3/C electrode at a relatively high rate of 4 A g−1 was further studied. In Figure 5E, it can be clearly found that the Coulombic efficiency could exceed 98% since the 10th cycle, and the electrode can still maintain a discharge capacity of 98 mAh g−1 even after 2,000 cycles. All these results indicate that NaTi2(PO4)3/C afforded improved electrochemical stability compared with that of NaTi2(PO4)3.
Furthermore, the rate capability of NaTi2(PO4)3/C electrode was also investigated by increasing rate from 0.02 to 4 A g−1 and back to 0.2 A g−1. As illustrated in Figure 5D, the discharge capability of NaTi2(PO4)3/C was 280 mAh g−1 at 0.02 A g−1, and then it slowly decreased with the increasing current density. When the current density was reversed to 0.2 A g−1, a capacity of 164 mAh g−1 could be restored. Obviously, NaTi2(PO4)3/C has excellent rate capacity.
Lastly, EIS measurements were carried out to further study the surface reaction activities of NaTi2(PO4)3/C and NaTi2(PO4)3. Before the EIS tests, the coin cells were cycled three times in the voltage range of 1.0–2.5 V, and the corresponding Nyquist plots are shown in Figure 6. It can be seen that each Nyquist plot exhibited a semicircle at high frequency region and a straight line at low frequency region. The surface charge-transfer resistance (Rct) of NaTi2(PO4)3/C was found to be smaller than that of NaTi2(PO4)3, suggesting that the diffusion of Na+ in NaTi2(PO4)3/C is faster than NaTi2(PO4)3 (Lu et al., 2014; Longoni et al., 2016). In addition, the Na+ diffusion coefficient (D) can be calculated by the following equations (Ko et al., 2017):
in which R is the ideal gas constant, T is the ambient temperature, A is the surface area of the electrode, n is the number of electrons per molecule during intercalation, F is the Faraday constant, C is the concentration of Na+ in the active material, σ is the Warburg coefficient, Z' is the real part of the impedance, ω is the angular frequency. The σ value can be calculated by the slope of the plot of Z' vs. ω−0.5 and presented in Figure S2. The σ value of NaTi2(PO4)3/C was 254 Ω s−0.5, much lower than that of NaTi2(PO4)3 (1264 Ω s−0.5). Accordingly D of NaTi2(PO4)3/C was larger than that of NaTi2(PO4)3. Summarily, NaTi2(PO4)3/C can effectively restrain the increasing of charge-transfer resistance after multiple discharge and charge cycles, which can improve the rate capability and enhance the cyclic performance at high rate (Song et al., 2014; Roy and Srivastava, 2015).
According to the above results, NaTi2(PO4)3/C has high discharge capacity, good rate capacity, and excellent long-term cycling stability. In addition, compared to other previously reported NaTi2(PO4)3@C composites, the obtained NaTi2(PO4)3/C electrode exhibits excellent properties (Table S1, Supporting Information). The good properties of NaTi2(PO4)3/C could be ascribed to the following reasons: (i) The crystal structure of NASICON-type NaTi2(PO4)3 is an open 3D framework of PO4 tetrahedra corner-shared with TiO6 octahedra, which can not only provide large spaces for Na+ insertion but also supply open tunnels for Na+ transport (Boilot et al., 1983; Pang et al., 2014b; Zhao et al., 2015). (ii) The porous structure of nanostructured NaTi2(PO4)3 and carbon matrix can decrease the diffusion length of Na+ (Gibaud et al., 1996; Huang et al., 2015; Rui et al., 2016). (iii) The embedding of NaTi2(PO4)3 nanocubes in carbon nanosheets can effectively inhibit the aggregation of the nanocubes, leading to the electrolyte easily penetrating to the active sites.
In summary, the composition of NaTi2(PO4)3 porous nanocubes and carbon porous nanosheet are successfully developed. The as-obtained NaTi2(PO4)3/C electrodes have good electrochemical properties, including large energy density, excellent rate capacity, and good cycling performance, owing to their special structures and components. The results demonstrate that such NaTi2(PO4)3/C anode is a promising anode for SIBs.
ZW, XL, CW, and JM design the whole experiment, and write the paper. JL and KF conduct some electrochemical analysis.
This work is supported by the National Natural Science Foundation of China (No. 21501101), the China Postdoctoral Science Foundation (No. 2017M622564), the Program for Science and Technology Innovation Talents in Universities of Henan Province (No. 15HASTIT007), and the Natural Science Foundation of Hunan Province (2017JJ1008).
The authors declare that the research was conducted in the absence of any commercial or financial relationships that could be construed as a potential conflict of interest.
The Supplementary Material for this article can be found online at: https://www.frontiersin.org/articles/10.3389/fchem.2018.00396/full#supplementary-material
Boilot, J. P., Collin, G., and Comes, R. (1983). Zirconium deficiency in nasicon-type compounds: crystal structure of Na5Zr(PO4)3. J. Solid State Chem. 50, 91–99. doi: 10.1016/0022-4596(83)90236-0
Chen, K. N., Wang, Q. R., Niu, Z. Q., and Chen, J. (2018). Graphene-based materials for flexible energy storage devices. J. Energy Chem. 27, 12–24. doi: 10.1016/j.jechem.2017.08.015
Choi, J. H., Ha, C. W., Choi, H. Y., Shin, H. C., Park, C. M., and Lee, S. M. (2016). Sb2S3 embedded in amorphous P/C composite matrix as high-performance anode material for sodium ion batteries. Electrochim. Acta 210, 588–595. doi: 10.1016/j.electacta.2016.05.190
Cui, C. Y., Wei, Z. X., Xu, J. T., Zhang, Y. Q., Liu, S. H., and Dou, S. X. (2018). Three-dimensional carbon frameworks enabling MoS2 as anode for dual ion batteries with superior sodium storage properties. Energ. Storage Mater. 15, 22–30. doi: 10.1016/j.ensm.2018.03.011
Ge, Y., Yildiz, O., and Zhang, X. (2015). Carbon-confined SnO2-electrodeposited porous carbon nanofiber composite as high-capacity sodium-ion battery anode material. ACS Appl. Mater. Inter 7, 18387–18396. doi: 10.1021/acsami.5b04338
Fan, H. S., Yu, H., Zhang, Y. F., Guo, J., Wang, Z., and Xu, J. (2018). 1D to 3D hierarchical iron selenide hollow nanocubes assembled from FeSe2@C core-shell nanorods for advanced sodium ion batteries. Energ. Storage Mater. 10, 48–55. doi: 10.1016/j.ensm.2017.08.006
Fang, Y., Xiao, L., Qian, J., Cao, Y., Ai, X., Huang, Y., et al. (2016). Three-dimensional graphene decorated NaTi2(PO4)3 microspheres as a superior high-rate and ultra cycle-stable anode material for sodium ion batteries. Adv. Energ. Mater. 6:1502197. doi: 10.1002/aenm.201502197
Fu, Y., Zhang, Z., Yang, X., and Gan, Y. (2015). ZnS nanoparticles embedded in porous carbon matrices as anode materials for lithium ion batteries. RSC Adv. 5, 86941–86944. doi: 10.1039/C5RA15108B
Gao, Y., Chen, K., Chen, H. M., Hu, X. H., Deng, Z. H., and Wei, Z. D. (2017). Surfactant assisted solvothermal synthesis of LiFePO4 nanorods for lithium-ion batteries. J. Energy Chem. 26, 564–568. doi: 10.1016/j.jechem.2016.10.016
Geng, H., Yang, J., Yu, H., Li, C., and Dong, X. (2017). Carbon intercalated porous NaTi2(PO4)3 spheres as high-rate and ultralong-life anodes for rechargeable sodium-ion batteries. Mater. Chem. Front. 1, 1435–1440. doi: 10.1039/C7QM00048K
Gibaud, A., Xue, J.S., and Dahn, J. R. (1996). Influence of the carbonization heating rate on the physical properties of activated carbons from a sub-bituminous coal. Carbon 34, 499–503. doi: 10.1016/0008-6223(95)00207-3
Guo, W., Li, X., Ng, D. H. L., and Ma, J. M. (2015). Integration of MnO@graphene with graphene networks towards Li-ion battery anodes. RSC Adv. 5, 96681–96684. doi: 10.1039/C5RA18927F
Hasegawa, G., Kanamori, K., Kannari, N., Ozaki, J. I., Nakanishi, K., and Abe, T. (2016). Studies on electrochemical sodium storage into hard carbons with binder-free monolithic electrodes. Power Sourc. J. 318, 41–48. doi: 10.1016/j.jpowsour.2016.04.013
Hu, A. J., Jin, S., Du, Z. Z., Jin, H. C., and Ji, H. X. (2018). NS codoped carbon nanorods as anode materials for high-performance lithium and sodium ion batteries. J. Energ. Chem. 27, 203–208. doi: 10.1016/j.jechem.2017.11.022
Hu, Q., Yu, M., Liao, J., Wen, Z., and Chen, C. (2018). Porous carbon-coated NaTi2(PO4)3 with superior rate and low-temperature properties. J. Mater. Chem. A 6, 2365–2370. doi: 10.1039/C7TA10207K
Huang, L., Cheng, J., Li, X., and Wang, B. (2015). Electrode nanomaterials for room temperature sodium-ion batteries: a review. J. Nanosci. Nanotechno. 15, 6295–6307. doi: 10.1166/jnn.2015.11122
Kabbour, H., Coillot, D., Colmont, M., Masquelier, C., and Mentré, O. (2011). α-Na3M2(PO4)3 (M = Ti, Fe): Absolute cationic ordering in NASICON-type phases. J. Am. Chem. Soc. 133, 11900–11903. doi: 10.1021/ja204321y
Ko, J. S., Doan-Nguyen, V. V., Kim, H. S., Petrissans, X., DeBlock, R. H., and Dunn, B. S. (2017). High-rate capability of Na2FePO4F nanoparticles by enhancing surface carbon functionality for Na-ion batteries. J. Mater. Chem. A 5, 18707–18715. doi: 10.1039/C7TA05680J
Li, S., Dong, Y., Xu, L., Xu, X., He, L., and Mai, L. (2014). Effect of carbon matrix dimensions on the electrochemical properties of Na3V2(PO4)3 nanograins for high-performance symmetric sodium-ion batteries. Adv. Mater. 26, 3545–3553. doi: 10.1002/adma.201305522
Liang, J., Fan, K., Wei, Z., Gao, X., Song, W., and Ma, J. (2018a). Porous NaTi2(PO4)3@C nanocubes as improved anode for sodium-ion batteries. Mater. Res. Bull. 99, 343–348. doi: 10.1016/j.materresbull.2017.11.030
Liang, J. J., Gao, X., Guo, J., Chen, C. M., Fan, K., and Ma, J. M. (2018b). Electrospun MoO2@NC nanofibers with excellent Li+/Na+ storage for dual applications. Sci. China. Mater. 61, 30–38. doi: 10.1007/s40843-017-9119-2
Liang, J. J., Wei, Z. X., Wang, C. Y., and Ma, J. M. (2018c). Vacancy-induced sodium-ion storage in N-doped carbon nanofiber@MoS2 nanosheet arrays. Electrochim. Acta 285, 301–308. doi: 10.1016/j.electacta.2018.07.230
Liang, J. J., Yuan, C. C., Li, H. H., Fan, K., Wei, Z. X., and Ma, J. M. (2018d). Growth of SnO2 nanoflowers on N-doped carbon nanofibers as anode for Li- and Na-ion batteries. Nano Micro Lett. 10:21. doi: 10.1007/s40820-017-0172-2
Longoni, G., Wang, J. E., Jung, Y. H., Kim, D. K., Mari, C. M., and Ruffo, R. (2016). The Na2FeP2O7-carbon nanotubes composite as high rate cathode material for sodium ion batteries. Power Sourc J. 302, 61–69. doi: 10.1016/j.jpowsour.2015.10.033
Lu, Y., Zhang, S., Li, Y., Xue, L., Xu, G., and Zhang, X. (2014). Preparation and characterization of carbon-coated NaVPO4F as cathode material for rechargeable sodium-ion batteries. Power Sourc. J. 247, 770–777. doi: 10.1016/j.jpowsour.2013.09.018
Pang, G., Nie, P., Yuan, C., Shen, L., Zhang, X., Li, H., et al. (2014a). Mesoporous NaTi2(PO4)3/CMK-3 nanohybrid as anode for long-life Na-ion batteries. J. Mater. Chem. A 2, 20659–20666. doi: 10.1039/C4TA04732J
Pang, G., Yuan, C., Nie, P., Ding, B., Zhu, J., and Zhang, X. (2014b). Synthesis of NASICON-type structured NaTi2(PO4)3-graphene nanocomposite as an anode for aqueous rechargeable Na-ion batteries. Nanoscale 6, 6328–6334. doi: 10.1039/C3NR06730K
Roh, H. K., Kim, M. S., Chung, K. Y., Ulaganathan, M., Aravindan, V., and Kim, K. B. (2017). A chemically bonded NaTi2(PO4)3/rGO microsphere composite as a high-rate insertion anode for sodium-ion capacitors. J. Mater. Chem. A 5, 17506–17516. doi: 10.1039/C7TA05252A
Roy, P., and Srivastava, S. K. (2015). Nanostructured anode materials for lithium ion batteries. J. Mater. Chem. A, 3, 2454–2484. doi: 10.1039/C4TA04980B
Rui, X., Sun, W., Wu, C., Yu, Y., and Yan, Q. (2016). An advanced sodium-ion battery composed of carbon coated Na3V2(PO4)3 in a porous graphene network. Adv. Mater. 27, 6670–6676. doi: 10.1002/adma.201502864
Senguttuvan, P., Rousse, G., Arroyo, M., De Dompablo, Y., Vezin, H., Tarascon, J., et al. (2013). Low potential sodium insertion in NASICON-type structure through the Ti(III)/Ti(II). redox couple. J. Am. Chem. Soc. 135, 3897–3903. doi: 10.1021/ja311044t
Song, H., Li, N., Cui, H., and Wang, C. (2014). Enhanced storage capability and kinetic processes by pores- and hetero-atoms- riched carbon nanobubbles for lithium-ion and sodium-ion batteries anodes. Nano Energ. 4, 81–87. doi: 10.1016/j.nanoen.2013.12.017
Sun, D., Jin, G., Tang, Y., Zhang, R., Xue, X., Huang, X., et al. (2016). NaTi2(PO4)3 nanoparticles embedded in carbon matrix as long-lived anode for aqueous lithium ion battery. J. Electrochem. Soc. 163, A1388–A1393. doi: 10.1149/2.1181607jes
Takashima, T., Tojo, T., Inada, R., and Sakurai, Y. (2015). Novel electrical energy storage system based on reversible solid oxide cells: system design and operating conditions. Power Sourc. J. 276, 113–119. doi: 10.1016/j.jpowsour.2014.11.109
Wan, M., Zeng, R., Chen, K. Y., Liu, G. X., Chen, W. L., and Huang, Y. H. (2018). Fe7Se8 nanoparticles encapsulated by nitrogen-doped carbon with high sodium storage performance and evolving redox reactions. Energ. Storage Mater. 10, 114–121. doi: 10.1016/j.ensm.2017.08.013
Wang, D., Liu, Q., Chen, C., Li, M., Meng, X., Bie, X., et al. (2016). NASICON-structured NaTi2(PO4)3@C nanocomposite as the low operation-voltage anode material for high-performance sodium-ion batteries. ACS Appl. Mater. Interf. 8, 2238–2246. doi: 10.1021/acsami.5b11003
Wang, G., Zhang, S., Li, X., Liu, X., Wang, H., and Bai, J. (2018). Multi-layer graphene assembled fibers with porous structure as anode materials for highly reversible lithium and sodium storage. Electrochim. Acta 259, 702–710. doi: 10.1016/j.electacta.2017.11.039
Wang, H., Yu, W., Mao, N., Shi, J., and Liu, W. (2016). Effect of surface modification on high-surface-area carbon nanosheets anode in sodium ion battery. Micropor. Mesopor. Mater. 227, 1–8. doi: 10.1016/j.micromeso.2016.02.003
Wei, Z. X., Wang, L., Zhuo, M., Ni, W., Wang, H. X., and Ma, J. M. (2018). Layered tin sulfide and selenide anode materials for Li- and Na-ion batteries. J. Mater. Chem. A 6, 12185–12214. doi: 10.1039/C8TA02695E
Wu, C., Kopold, P., Ding, Y. L., Aken, P. A. V., Maier, J., and Yu, Y. (2015). Synthesizing porous NaTi2(PO4)3 nanoparticles embedded in 3D graphene networks for high-rate and long cycle-life sodium electrodes. ACS Nano 9, 6610–6618. doi: 10.1021/acsnano.5b02787
Wu, X., Cao, Y., Ai, X., Qian, J., and Yang, H. (2013). A low-cost and environmentally benign aqueous rechargeable sodium-ion battery based on NaTi2(PO4)3-Na2NiFe(CN)6 intercalation chemistry. Electrochem. Commun. 31, 145–148. doi: 10.1016/j.elecom.2013.03.013
Ye, S., Li, Z., Song, T., Cheng, D., Xu, Q., Liu, H., et al. (2017). Self-generated hollow NaTi2(PO4)3 nanocubes decorated with graphene as a large capacity and long lifetime anode for sodium-ion batteries. RSC Adv. 7, 56743–56751. doi: 10.1039/C7RA12291H
Zhang, F., Li, W., Xiang, X., and Sun, M. (2017). Nanocrystal-assembled porous Na3MgTi(PO4)3 aggregates as highly stable anode for aqueous sodium-ion batteries. Chem. Eur. J. 23, 12944–12948. doi: 10.1002/chem.201703044
Zhao, B., Lin, B., Zhang, S., and Deng, C. (2015). A frogspawn-inspired hierarchical porous NaTi2(PO4)3-C array for high-rate and long-life aqueous rechargeable sodium batteries. Nanoscale 7, 18552–18560. doi: 10.1039/C5NR06505D
Zhao, X., Yan, C., Gu, X., Li, L., Dai, P., Li, D., et al. (2017). Ultrafine TiO2 nanoparticles confined in N-doped porous carbon networks as anodes of high-performance sodium-ion batteries. Chem. Electro. Chem. 4, 1516–1522. doi: 10.1002/celc.201700159
Keywords: NaTi2(PO4)3, nanocubes, carbon nanosheets, anode, sodium-ion batteries
Citation: Wang Z, Liang J, Fan K, Liu X, Wang C and Ma J (2018) Porous NaTi2(PO4)3 Nanocubes Anchored on Porous Carbon Nanosheets for High Performance Sodium-Ion Batteries. Front. Chem. 6:396. doi: 10.3389/fchem.2018.00396
Received: 05 June 2018; Accepted: 16 August 2018;
Published: 19 September 2018.
Edited by:
Qiaobao Zhang, Xiamen University, ChinaReviewed by:
Chao Lai, Jiangsu Normal University, ChinaCopyright © 2018 Wang, Liang, Fan, Liu, Wang and Ma. This is an open-access article distributed under the terms of the Creative Commons Attribution License (CC BY). The use, distribution or reproduction in other forums is permitted, provided the original author(s) and the copyright owner(s) are credited and that the original publication in this journal is cited, in accordance with accepted academic practice. No use, distribution or reproduction is permitted which does not comply with these terms.
*Correspondence: Xiaodi Liu, bGl1eGlhb2RpbnlAMTI2LmNvbQ==
Caiyun Wang, Y2FpeXVuQHVvdy5lZHUuYXU=
Jianmin Ma, bmFub2VsZWNoZW1AaG51LmVkdS5jbg==
Disclaimer: All claims expressed in this article are solely those of the authors and do not necessarily represent those of their affiliated organizations, or those of the publisher, the editors and the reviewers. Any product that may be evaluated in this article or claim that may be made by its manufacturer is not guaranteed or endorsed by the publisher.
Research integrity at Frontiers
Learn more about the work of our research integrity team to safeguard the quality of each article we publish.