- 1Science and Technology on Plasma Physics Laboratory, Research Centre of Laser Fusion, China Academy of Engineering Physics, Mianyang, China
- 2School of Materials Science and Engineering, Southwest University of Science and Technology, Mianyang, China
- 3College of Materials Science and Engineering, Chongqing University, Chongqing, China
In this study, we described a facile process for the fabrication of tungsten oxide dihydrate/bamboo charcoal hybrids (WO3·2H2O/BC) by the γ-irradiation method. The structural, morphological, and electrochemical properties of WO3·2H2O/BC hybrids were investigated using X-ray diffraction (XRD), field emission scanning electron microscopy (FESEM), transmission electron microscopy (TEM), cyclic voltammetry (CV), galvanostatic charge/discharge (GCD), and electrochemical impedance spectroscopy (EIS) techniques. The combination of BC (electrical double layer charge) and WO3·2H2O (pseudocapacitance) created a combined effect, which enhanced the specific capacitance and superior cyclic stability of the WO3·2H2O/BC hybrid electrode. The WO3·2H2O/BC hybrids showed the higher specific capacitance (391 F g−1 at 0.5 A g−1 over the voltage range from −1 to 0 V), compared with BC (108 F g−1) in 6 M KOH solution. Furthermore, the hybrid electrode showed superior long-term performance with 82% capacitance retention even after 10,000 cycles. The experimental results demonstrated that the high performance of WO3·2H2O/BC hybrids could be a potential electrode material for supercapacitors.
Introduction
For the rapid increase of global energy demand and the depletion risk of fossil fuels, developing alternative sustainable, affordable, efficient, and clean energy has become very important (Zhao Y. et al., 2016, 2017; Xu et al., 2017; Yi et al., 2017; Zhao B. et al., 2017). Among energy storage devices, supercapacitors (or ultracapacitors) are promising, owing to their safe operation, super-high service life, and great power density (Wang K. et al., 2014; Dai et al., 2017; Zhao B. et al., 2017; Zhao et al., 2018). They have wide application areas such as electric vehicles, pulse power systems and portable devices (Wang et al., 2018). Depending on the charge storage mechanism, supercapacitors are generally classified into electrical double layer charge (EDLC) and pesudoprocess charge storage. The former stores charges electrostatically in double layers, whereas the latter stores charges on the surface of the electrode active materials as faradaic redox reactions (Zhang and Park, 2017). In general, carbon-based materials are EDLC type (Pang et al., 2016), whereas transition metal oxides and conducting polymers are pseudocapacitor-type materials (Zhang et al., 2012; Yao C. et al., 2017; Yao S. et al., 2017).
The charge storage of pesodocapacitors is much higher compared to those of EDLCs. More recently, transition metal oxides (i.e., RuO2, V2O5, NiO, MnO2, SnO2, and WO3) have been widely investigated in the applications for supercapacitors, and their charge storage originates from fast superficial redox reactions (Zhu and He, 2012; Zhang et al., 2015; Qiu et al., 2016; Zeng et al., 2017; Zhang and Park, 2017; Liu et al., 2018a). Among many of the reported transition metal oxides, RuO2 has been considered as the proper material with excellent capacitive performance. However, RuO2 is expensive and rare, constraining the wide practical applications in electrode materials (Cai et al., 2014). WO3 is a promising electrode material owing to its various morphologies, high theoretical specific capacitance, environmental friendliness, and low cost (Qiu et al., 2016). Nevertheless, its low electrical conductivity (10−5–10−6 S cm−1) has limited the wide applications. If we can improve the conductivity of WO3, higher specific capacitances could be achieved as expected. Therefore, many researchers have focused on incorporating WO3 with highly conductive carbon materials to establish a hybrid-type material that combines advantages of each component (Lu et al., 2012; Xiao et al., 2012; Reddy et al., 2015; Sun et al., 2015; Wang et al., 2015; Yuksel et al., 2016). Wang et al. fabricated the WO3/carbon aerogel hybrids with outstanding long-term stability, and the specific capacitance was ~700 F g−1 (at a scan rate of 25 mV s−1 in 0.5 M H2SO4 over a voltage window of −0.3 to 0.5 V; Wang Y. H. et al., 2014). Huang et al. also designed graphene-WO3 hybrids with enhanced supercapacitor capacitance (Xing et al., 2016). Wang et al. fabricated the graphene nanosheets-tungsten oxides with high supercapacitor performance (Wang et al., 2015). Ma et al. fabricated a hybrid based on graphene and WO3 via the hydrothermal method, which possessed high specific capacitance and superior rate capability (Ma et al., 2015).
Among the conductive materials, activated carbon-based materials are the most promising candidates for supercapacitor applications, due to their unique characteristics of large surface areas, and high electrochemical stability and conductivity (Yang et al., 2014; Li and Wu, 2015; Wang et al., 2016; Boyjoo et al., 2017; Dai et al., 2018). In carbon materials, bamboo charcoal draws research attention for its extraordinarily porous microstructure, cost efficiency, and high absorptive capacity (Wang et al., 2012, 2013, 2016; Zhang et al., 2013; Yang et al., 2014; Li and Wu, 2015; Yu et al., 2015). Li et al. studied the water bamboo-derived porous carbon with a maximum specific capacitance of 268 F g−1 at a current density of 1 A g−1 in 6 M KOH electrolyte and good capacity retention of 97.28% even over 5,000 cycles at a current density of 10 A g−1 (Li and Wu, 2015). Yang et al. also synthesized BC by KOH activation, and the specific capacity retention was more than 91% after 3,000 cycles (Yang et al., 2014). Impressively, BC has long-lasting life, whereas WO3 has high theoretical specific capacity. For this respect, combining each advantage of BC and WO3·2H2O to improve the performance might bring novel and excellent properties. However, so far, to the best of our knowledge, nearly no works have been done in this aspect.
For decades, researchers have reported many ways to fabricate WO3/carbon materials, such as hydrothermal method, impregnation methods, and sol-gel method. Compared with these methods, as we previously reported, the radiation method can improve the contact between the doped materials and pristine carbon, and this method has been successfully applied in H2 storage (Zhong et al., 2015, 2016). Obviously, good adhesion may improve the stability of hybrids and optimize the conduction of electrons, which will enhance the capacitive properties of the hybrids. But so far, no studies have been conducted on the preparation of metal oxides and carbon hybrid materials for supercapacitor applications by the irradiation method. To widen application of this method and further improve the capacitive performance, it is important to develop this method.
In this work, novel WO3·2H2O/BC hybrids were designed and fabricated by a facile γ-irradiation strategy. Morphologies and microstructures of the samples were investigated by XRD, SEM, TEM, and XPS, whereas CV, GCD, and EIS were carried out to study capacitive properties. The electrochemical results demonstrated that WO3·2H2O/BC hybrids delivered a high specific capacity (391 F g−1 at 0.5 A g−1) and superior long-term stability (82% retention even after 10,000 cycles). The combination of bamboo (EDLC) and WO3·2H2O (pseudocapacitance) provided short ion diffusion path and fast electron transport, leading to a great supercapacitor.
Experimental Details
Synthesis of WO3·2H2O/BC Hybrids
All the reagents and solvents were analytical grade and used without further purification. In a typical process, WO3·2H2O/BC hybrids were prepared as follows. WCl6 (3 mg) was added into isopropyl alcohol (20 mL) under stirring for 20 min in a glass vial at room temperature. Then, the BC monoliths (0.2 g) were slowly impregnated with 10 ml of WCl6 solution. After 30 min of continuous stirring, 2-propanol was added with proper amount to scavenge H* and OH* radicals, which were generated during irradiation. The mixture was irradiated at room temperature with a 60Co γ-ray source at a dose rate of 200 Gy min−1, and the total dose was 500 kGy. The product was collected by centrifugation and rinsed several times with DI water and ethanol, and then dried at 60°C for 12 h. Figure 1 shows the schematic diagram for the synthesis of WO3·2H2O/BC hybrids and their supercapacitor performance. For comparison, bamboo charcoal was prepared by carbonization of natural bamboo with the KOH-modified method, as described elsewhere (Yang et al., 2014; Li and Wu, 2015).
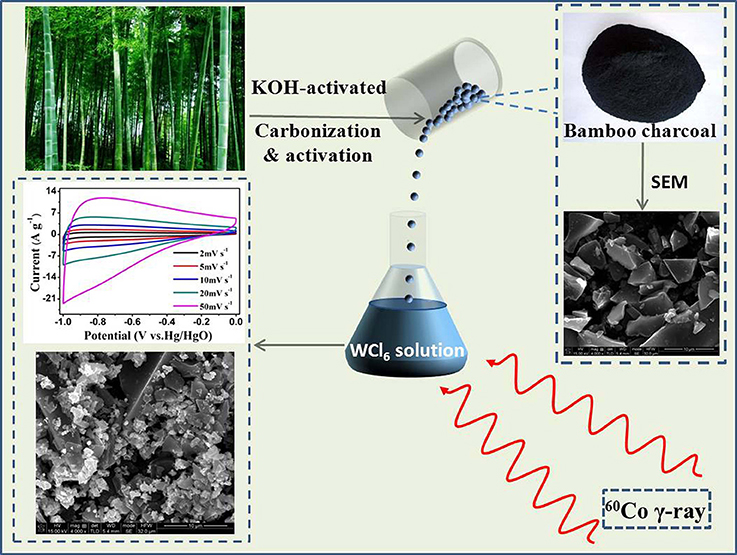
Figure 1. Schematic diagram for the synthesis of WO3·2H2O/BC hybrids and their supercapacitor performance.
Material Characterization
The crystalline phase of WO3·2H2O/BC hybrids was examined by X-ray powder diffraction (XRD) employing monochromatized CuKα incident radiation. The morphology and microstructure were analyzed by using a field emission scanning electron microscope (FESEM, Nova 600i) with an attached energy dispersive X-ray spectroscopy (EDS) analysis and transmission electron microscopy (TEM). X-ray photoelectron spectroscopy (XPS) was studied for detecting chemical composition and oxidation states of WO3·2H2O/BC hybrids.
Electrochemical Measurements
Electrochemical tests were carried out at room temperature in a conventional three-electrode configuration with 6 M KOH as an electrolyte using an electrochemical workstation (CHI 660E), WO3·2H2O/BC as a working electrode, platinum foil as a counter electrode, and Hg/HgO as a reference electrode. In electrochemical tests, WO3·2H2O/BC (80%) was mixed with acetylene black (10%) and polyvinylidene fluoride (PVDF, 10%) in N-methyl-2-pyrrolidone (NMP) to form slurry. Then the slurry was coated on glassy carbon to fabricate the working electrode. The potential range for CV tests is from −1 to 0 V, and the measurement range for EIS tests is between 0.1 Hz and 100 kHz with an AC amplitude of 5 mV.
Results and Discussion
Characterization of WO3·2H2O/BC Hybrids
Crystal structures of the as-prepared BC and WO3·2H2O/BC hybrids were first studied by XRD. As shown in Figure 2, two broad peaks near 23 and 43° correspond to (002) and (100), respectively, which can be identified for the amorphous forms of BC. For WO3·2H2O/BC hybrids, two broad peaks of BC also appeared, and all the peaks exclusively assigned to the characteristic structure of WO3·2H2O (JCPDS-00-18-1420). Furthermore, no other impurity phase peak can be detected, and the existence of strong and sharp peaks also indicates that WO3·2H2O/BC hybrids have high crystallinity. The structure of WO3·2H2O has been reported to be attractive as electrode materials (Ma et al., 2015; Li et al., 2016; Mitchell et al., 2017). Crystalline WO3 is much more stable than amorphous WO3 due to the denser structure and lower dissolution rate in electrolytes, which is a very important point in terms of practical applications (Liu et al., 2017). The diffraction pattern of WO3·2H2O/BC hybrids is the combination of the peaks from BC and WO3·2H2O, demonstrating the successful composite. However, for the amorphous forms of BC, no distinct peaks of BC can be observed in WO3·2H2O/BC hybrids. Nevertheless, the presence of BC in the WO3·2H2O/BC hybrids can be confirmed by the results of SEM, TEM, and XPS.
The surface morphologies of BC and WO3·2H2O/BC hybrids were characterized by SEM and TEM. As shown in Figure 3a, we can clearly see that the BC has smooth surface and irregular forms. Figure 3b shows the morphology of WO3·2H2O/BC hybrids, and the skeleton of BC can be clearly seen with a random distribution of WO3·2H2O. EDS mapping was further used to demonstrate the formation of WO3·2H2O/BC hybrids, and is shown in Figure 3c. Obviously, the C, W, and O elements exist in WO3·2H2O/BC hybrids, and this result is in accord with the EDS spectrum shown in Figure 3d. As shown in Figures 3e,f, TEM and high-resolution TEM (HRTEM) micrographs further indicate microscopic structures of WO3·2H2O/BC hybrids. The TEM image of WO3·2H2O/BC hybrids clearly reveals that WO3·2H2O is successfully connected to BC. The HRTEM image of WO3·2H2O/BC (Figure 3f) shows that the spacing between adjacent lattice planes is 0.37 nm, corresponding to the (001) plane of WO3·2H2O, indicating that WO3·2H2O grows preferentially along (001) (Mitchell et al., 2017). Therefore, WO3·2H2O/BC hybrids can be successfully synthesized via a simple γ-irradiation method, in which WO3·2H2O is sufficiently connected to BC. And the robust contact between WO3 and BC can be maintained by ultrasound for 30 min without WO3 shed (see Figure 3e). This great interfacial contact between WO3·2H2O and BC may be possibly favorable for the electronic transport process, thus resulting in the enhanced supercapacitor performance (Cai et al., 2014; Chu et al., 2017; Liu et al., 2018a,b). To further check the surface chemical composition of WO3·2H2O/BC hybrids, XPS measurements were carried out.
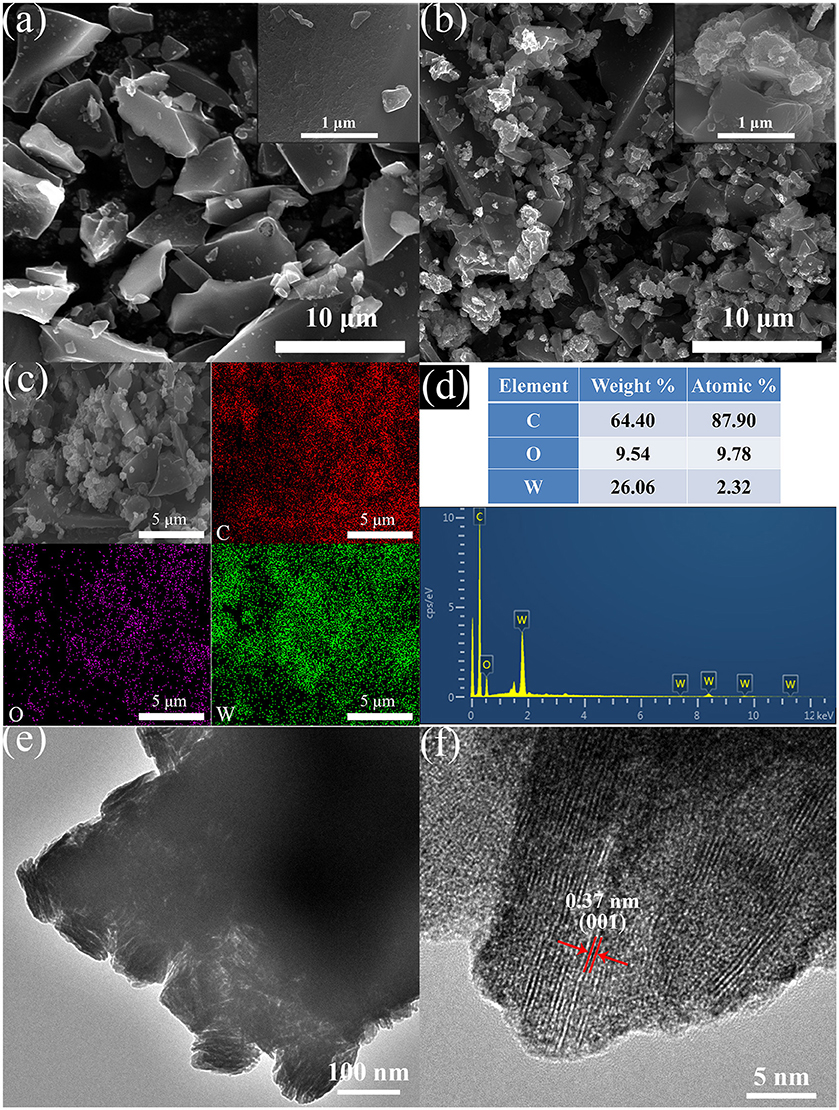
Figure 3. Typical SEM images of (a) BC and (b) WO3·2H2O/BC hybrids. (c) The EDS mapping results and (d) the corresponding spectrum for the WO3·2H2O/BC hybrids. (e) TEM and (f) HRTEM images of the WO3·2H2O/BC hybrids.
The detailed composition and surface valence state of WO3·2H2O/BC hybrids was probed by XPS measurements. Figure 4A shows the survey scan spectrum of the WO3·2H2O/BC hybrids, and only W, O, and C elements exist in the hybrids without evidence of any other impurity atoms. Figure 4B shows the XPS spectrum of the W 4f doublet peak in the high-resolution scan. The peaks located at 37.87 and 35.73 eV are attributable to the W 4f5/2 and W 4f7/2, respectively. The observed energy position of the doublet is in accord with the previous report for the W6+ oxidation state (Shinde et al., 2016; Xu et al., 2016; Liu et al., 2017; Wu and Yao, 2017). The splitting between W 4f7/2 and W 4f5/2 is 2.14 eV, demonstrating a typical state of W6+ in WO3·2H2O/BC hybrids, which is well analogous to the XRD study. The XPS spectrum of C 1s from the WO3·2H2O/BC hybrids (see Figure 4C) is also decomposed into three peaks at 284.57 eV (C–C), 285.68 eV (C–O), and 287.81 eV (C = O), suggesting that the bonding between carbon atoms of BC and oxygen atoms of WO3·2H2O improves the conductivity and accelerates charge transport for the hybrids (Cai et al., 2014; Nayak et al., 2017). And the deconvolution peak of the O 1s spectrum can be resolved into two components of 530.98 and 533.02 eV (see Figure 4D). The low binding energy component at 530.98 eV is attributed to the O2− bond with wolfram, and the latter peak is assigned to OH− (Xing et al., 2016; Nayak et al., 2017).
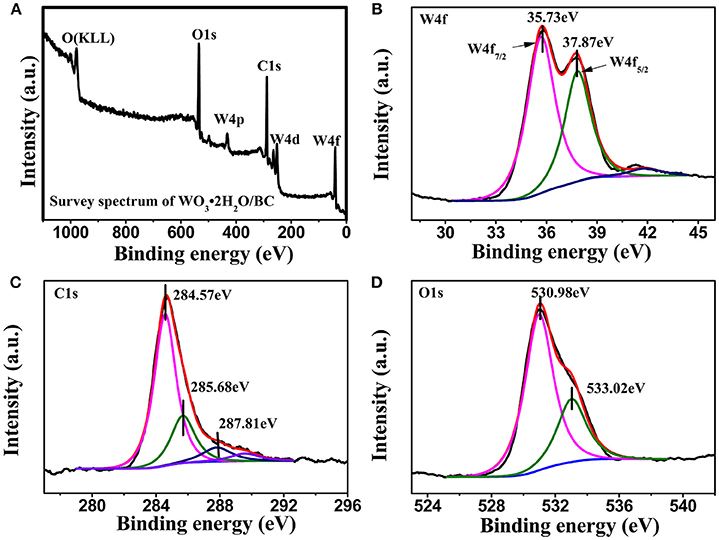
Figure 4. The XPS spectra of WO3·2H2O/BC (A) survey scan spectrum, (B) W 4f core level spectrum, (C) C 1s core level spectrum, and (D) O 1s core level spectrum.
Electrochemical Performance of WO3·2H2O/BC Hybrids
The electrochemical performance of WO3·2H2O/BC hybrids was first investigated by the CV test. The CV curves of the BC and WO3·2H2O/BC electrodes are shown in Figure 5A. Compared with those of BC, the CV curves of WO3·2H2O/BC changed from the rectangular shape to the “dolphin-like” shape, and nearly no obvious redox peaks detected are characteristic among various WO3 (Reddy et al., 2015; Thind et al., 2016; He et al., 2017; Nayak et al., 2017). Moreover, the stored charge of the hybrids can be calculated by the enclosed area of the CV curve. The observed integrated area of the WO3·2H2O/BC electrode at the same current density is much larger than that of the BC electrode, suggesting the contribution of WO3·2H2O incorporation to the enhanced specific capacitance of WO3·2H2O/BC, and the combined effect of WO3·2H2O and BC is significant (Cai et al., 2014; Liu et al., 2018a). The results obtained here are also consistent with the SEM and TEM morphologies, suggesting that the WO3·2H2O/BC hybrid morphology provides good contact fascinating the fast charge intercalation/deintercalation process, and the enhanced electronic conductivity after carbon incorporation contributes to the superior electrochemical performance. Figure 5B shows the CV curves of WO3·2H2O/BC in 6 M KOH at different scan rates (2, 5, 10, 20, and 50 mV s−1) over a potential window of −1 to 0 V. The corresponding CV curves of BC are provided in Figure S1a. The increased area under the curve with scan rate is clearly observed, indicating an excellent capacitance behavior and high-rate capability of the electrode.
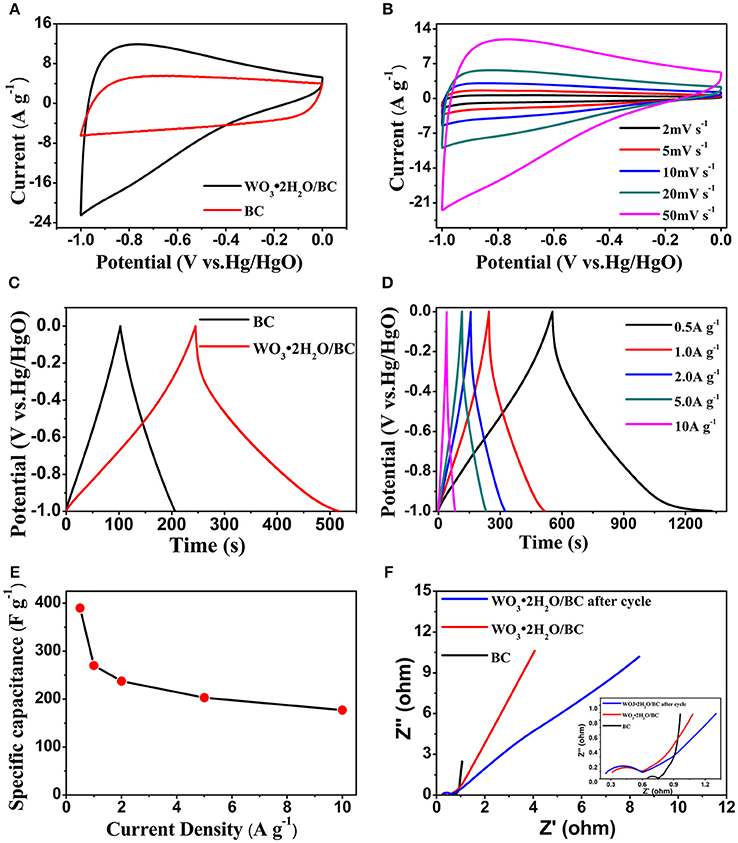
Figure 5. (A) The CV curves of BC and WO3·2H2O/BC electrodes at a scan rate of 50 mV s−1. (B) The CV curves of the WO3·2H2O/BC electrode at different scan rates. (C) GCD curves of BC and WO3·2H2O/BC electrodes at a current density of 1 A g−1. (D) GCD curves of the WO3·2H2O/BC electrode at different current densities. (E) Specific capacitance of the WO3·2H2O/BC electrode at different current densities. (F) EIS of the BC and WO3·2H2O/BC electrodes.
Charge-discharge measurements were conducted under galvanostatic conditions at different applied current densities. The GCD plots of the BC and WO3·2H2O/BC electrodes at a current density of 1 A g−1 are presented in Figure 5C. According to the galvanostatic discharge curves, the specific capacitance (Cs, F g−1) of the electrode is calculated according to the following equation:
where I (mA) represents the applied current, Δt (s) the discharge time, ΔV (V) the potential window, and m (mg) the weight of the active material. In addition, the discharge time of WO3·2H2O/BC is much larger than that of BC, showing higher capacitance. And this result is also consistent with the CV tests. GCD curves of WO3·2H2O/BC and BC (Figure S1b) electrodes recorded at 0.5, 1, 2, 5, and 10 A g−1 are shown in Figure 5D. With the increasing charging and discharging currents, a highly linear and nearly symmetric relationship between the potential and time was also observed, suggesting the desired fast charging and discharging property of the materials. No obvious internal resistance (IR) drop of the BC (Figure S1b) and WO3·2H2O/BC electrodes was observed for any of the curves, which indicates high conductivity of the materials. The higher Cs of WO3·2H2O/BC is due to the combined effects between WO3·2H2O and BC (Chu et al., 2017), and the superior electrochemical performance may be mainly attributed to the enhanced electronic conductivity after carbon incorporation. As shown in Figure 5E, the calculated Cs of WO3·2H2O/BC are 391, 270, 227.5, 203, and 177 F g−1 at current densities of 0.5, 1, 2, 5, and 10 A g−1, respectively, demonstrating that the Cs decreases with increasing current density (Shinde et al., 2017). The coulombic efficiency of WO3.2H2O/BC hybrids is ~100%, exhibiting a good reversibility during the charge/discharge process. Furthermore, ~45% of the capacitance was retained when the current density increased from 0.5 to 10 A g−1, higher than the reported graphene nanosheets-tungsten oxides composites (34% capacitance retention from 0.1 to 5 A g−1), owing to the sluggish intercalation/deintercalation process of WO3.2H2O/BC at a high scan rate (Cai et al., 2014). These results are comparable to those of WO3-carbon-based electrodes in earlier reports (Table S1). To sum up, the enhancement in the electrochemical performance for WO3·2H2O/BC hybrids is mainly explained as follows: (i) the great interfacial contact between WO3·2H2O and BC provides short ion diffusion paths and the rapid electronic transports; (ii) the superior electrochemical performance may be mainly attributed to the enhanced electronic conductivity after carbon incorporation.
The EIS study was conducted to elucidate electrical conductivity and ion transfer features of BC and WO3·2H2O/BC electrodes. Electrochemical impedance characteristics of the electrode were investigated in a frequency range from 100 kHz to 0.1 Hz with an AC amplitude of 5 mV in 6 M KOH electrolyte. Figure 5F displays the typical Nyquist plots of these BC and WO3·2H2O/BC samples and the inset figure shows the magnified plots. For all the Nyquist plots, a semicircle can be seen in the high-frequency region, whereas in the low-frequency region, a straight line can be found. According to the previous reports (Sun et al., 2015; Chu et al., 2017; Yao C. et al., 2017), a straight line should relate to the ion diffusion into the active electrode (Zw), whereas the semicircle can be assigned to the charge transfer resistance (Rct) owing to the faradic and non-faradic reactions at the electrode/electrolyte interface. After doped with WO3·2H2O, the WO3·2H2O/BC electrode exhibits larger Rct and Zw than BC, mainly due to the poor electric conductivity of WO3.2H2O (Shinde et al., 2017). For comparison of first cycle and after 10,000 cycles, the Rct of WO3·2H2O/BC hybrids after the cycle is slightly larger than the value before the cycle, suggesting that WO3·2H2O/BC hybrids have good stability (Cai et al., 2014).
The cycling stability is the key factor to evaluate the practical applications of electrodes. To explore this, the cycle stability of WO3·2H2O/BC hybrids was further investigated by repeating the GCD test between −1 and 0 V at 4 A g−1 for 10,000 cycles (Figure 6). Impressively, after 10,000 cycles, the initial Cs (220.8 F g−1) of the WO3·2H2O/BC electrode slightly declined to 180.8 F g−1, and approximately 82% of the initial capacitance was retained. The inset shows the 1st, 4,000th and 10,000th GCD curves, respectively, indicating that the GCD profiles retain superior linearity and symmetry even after 10,000 cycles. Compared with other studies, to the best of our knowledge, in our work, the WO3·2H2O/BC hybrid electrode shows comparable cycling stability (Table S1); moreover the BC used in our work is much cheaper. Therefore, the combined effect of WO3·2H2O and BC accelerates the sufficient ion diffusion. This result confirms that the prepared WO3·2H2O/BC hybrids were highly stable as a novel supercapacitor electrode.
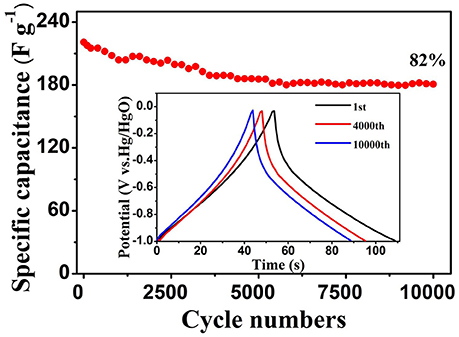
Figure 6. Cycling stability of the WO3·2H2O/BC electrode at 4 A g−1; the inset shows GCD curves at different cycles.
Figure 7 shows the storage mechanism of WO3·2H2O/BC hybrids. Briefly, the mechanism of WO3·2H2O/BC hybrids can be described as follows. First, the high conductivity of BC facilitates the rapid transfer of electrons. Secondly, after the γ-irradiation process, the good contact of BC and WO3·2H2O guarantees a low internal resistance between BC and WO3·2H2O, and also facilitates the transmission of low-loss electrons to the anchored WO3·2H2O. Thirdly, the structure of WO3·2H2O facilitates ion adsorption and insertion in the electrolyte, so an enhanced pseudocapacitor process can be formed on the surface of WO3·2H2O. In addition, the good contact of BC and WO3 also makes it difficult for WO3·2H2O to agglomerate during the charge/discharge process, and also ensures the long-term stability of WO3·2H2O/BC hybrids. All of these characteristics of WO3·2H2O/BC hybrids contribute to the high specific capacitance and good cycling stability.
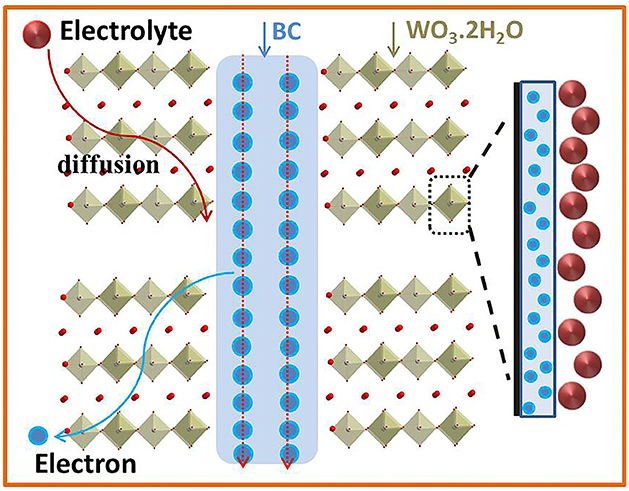
Figure 7. Anchored WO3.2H2O crystals with conductive BC; both electron and electrolyte can access WO3.2H2O surfaces, and an enhanced pseudocapacitive process can be formed on the surface of WO3.2H2O/BC.
Conclusions
In summary, we successfully synthesized WO3·2H2O/BC hybrids via a facile γ-irradiation method. The electrochemical capacitive behaviors of the WO3·2H2O/BC hybrids and BC have been demonstrated in 6 M KOH electrolyte between −1 and 0 V. In comparison with the BC electrode, the WO3·2H2O/BC hybrid electrode showed a higher Cs (391 F g−1 at 0.5 A g−1) and superior cycling performance (approximately 82% retention even after 10,000 cycles). The excellent performance achieved by the WO3·2H2O/BC hybrid electrode is owing to the combined effect of BC with good conductivity and WO3·2H2O with superior pseudocapacitive behavior. This γ-irradiation method would also pave a new way of designing other conducting semiconductors as promising electrode materials with enhanced performance for energy storage device applications.
Ethics Statement
On behalf of, and having obtained permission from all the authors, I declare that: (a) the material has not been published in whole or in part elsewhere; (b) the paper is not currently being considered for publication elsewhere; (c) all authors have been personally and actively involved in substantive work leading to the report, and will hold themselves jointly and individually responsible for its content; I testify to the accuracy of the above on behalf of all the authors.
Author Contributions
YT developed the concept. XDL designed the experiments. FY and JJ conducted the experiments. RM and XCL built the cells and carried out the performance characterizations. ZF and CW supervised the research. FY and JJ co-wrote the manuscript. All authors discussed the results and commented on the manuscript.
Funding
This study was financially supported by the Research Program of Sichuan province (grant no. 2016GZ0235) and the Development Foundation of China Academy of Engineering Physics (grant no. 2015B0302003) and the national key scientific instrument and equipment development project of China (grant no. 2014YQ090709).
Conflict of Interest Statement
The authors declare that the research was conducted in the absence of any commercial or financial relationships that could be construed as a potential conflict of interest.
Acknowledgments
We acknowledge Q. Yang, Y. Zeng, and J. Li (Science and Technology on plasma physics Laboratory, Research Centre of Laser Fusion, China Academy of Engineering physics, Mianyang, China) for the TEM, SEM, and XRD measurements, respectively.
Supplementary Material
The Supplementary Material for this article can be found online at: https://www.frontiersin.org/articles/10.3389/fchem.2018.00290/full#supplementary-material
References
Boyjoo, Y., Cheng, Y., Zhong, H., Tian, H., Pan, J., Pareek, V. K., et al. (2017). From waste Coca Cola® to activated carbons with impressive capabilities for CO2 adsorption and supercapacitors. Carbon 116, 490–499. doi: 10.1016/j.carbon.2017.02.030
Cai, Y., Wang, Y., Deng, S., Chen, G., Li, Q., Han, B., Han, R., Wang, Y., et al. (2014). Graphene nanosheets-tungsten oxides composite for supercapacitor electrode. Ceram. Int. 40, 4109–4116. doi: 10.1016/j.ceramint.2013.08.065
Chu, J., Lu, D., Wang, X., Wang, X., and Xiong, S. (2017). WO3 nanoflower coated with graphene nanosheet: synergetic energy storage composite electrode for supercapacitor application. J. Alloys Compd. 702, 568–572. doi: 10.1016/j.jallcom.2017.01.226
Dai, S., Liu, Z., Zhao, B., Zeng, J., Hu, H., Zhang, Q., et al. (2018). A high-performance supercapacitor electrode based on N-doped porous grapheme. J. Power Sources 387, 43–48. doi: 10.1016/j.jpowsour.2018.03.055
Dai, S., Zhao, B., Qu, C., Chen, D., Dang, D., Song, B., et al. (2017). Controlled synthesis of three-phase NixSy/rGO nanoflake electrodes for hybrid supercapacitors with highenergy and power density. Nano Energy 33, 522–531. doi: 10.1016/j.nanoen.2017.01.056
He, G., Ling, M., Han, X., Abou El Amaiem, D. I., Shao, Y., Li, Y., et al. (2017). Self-standing electrodes with core-shell structures for high-performance supercapacitors. Energy Storage Mater. 9, 119–125. doi: 10.1016/j.ensm.2017.07.005
Li, H., Wang, J., Shi, Q., Zhang, M., Hou, C., Shi, G., et al. (2016). Constructing three-dimensional quasi-vertical nanosheet architectures from self-assemble two-dimensional WO3·2H2O for efficient electrochromic devices. Appl. Surf. Sci. 380, 281–287. doi: 10.1016/j.apsusc.2016.01.009
Li, J., and Wu, Q. (2015). Water bamboo-derived porous carbons as electrode materials for supercapacitors. New J. Chem. 39, 3859–3864. doi: 10.1039/c4nj01853b
Liu, B., Wang, Y., Jiang, H., and Zou, B. X. (2017). WO3 nanowires on graphene sheets as negative electrode for supercapacitors. J. Nanomater. 24, 109–117. doi: 10.1155/2017/2494109
Liu, X., Sheng, G., Zhong, M., and Zhou, X. (2018a). Hybrid nanowires and nanoparticles of WO3 in a carbon aerogel for supercapacitor applications. Nanoscale 10, 4209–4217. doi: 10.1039/c7nr07191d
Liu, X., Sheng, G., Zhong, M., and Zhou, X. (2018b). Dispersed and size-selected WO3 nanoparticles in carbon aerogel for supercapacitor applications. Mater. Design 141, 220–229. doi: 10.1016/j.matdes.2017.12.038
Lu, X., Zhai, T., Zhang, X., Shen, Y., Yuan, L., Hu, B., et al. (2012). WO3−x@Au@MnO2 core-shell nanowires on carbon fabric for high-performance flexible supercapacitors. Adv. Mater. 24, 938–944. doi: 10.1002/adma.201104113
Ma, L., Zhou, X., Xu, L., Xu, X., Zhang, L., Ye, C., et al. (2015). Hydrothermal preparation and supercapacitive performance of flower-like WO3·H2O/reduced graphene oxide composite. Colloids Surf. A 481, 609–615. doi: 10.1016/j.colsurfa.2015.06.040
Mitchell, J. B., Lo, W. C., Genc, A., LeBeau, J., and Augustyn, V. (2017). Transition from battery to pseudocapacitor behavior via structural water in tungsten oxide. Chem. Mater. 29, 3928–3937. doi: 10.1021/acs.chemmater.6b05485
Nayak, A. K., Das, A. K., and Pradhan, D. (2017). High performance solid-State asymmetric supercapacitor using green synthesized graphene-WO3 nanowires nanocomposite. ACS Sustainable Chem. Eng. 5, 10128–10138. doi: 10.1021/acssuschemeng.7b02135
Pang, L., Zou, B., Zou, Y., Han, X., Cao, L., Wang, W., et al. (2016). A new route for the fabrication of corn starch-based porous carbon as electrochemical supercapacitor electrode material. Colloids Surf. A 504, 26–33. doi: 10.1016/j.colsurfa.2016.05.049
Qiu, M., Sun, P., Shen, L., Wang, K., Song, S., Yu, X., et al. (2016). WO3 nanoflowers with excellent pseudo-capacitive performance and the capacitance contribution analysis. J. Mater. Chem. 4, 7266–7273. doi: 10.1039/c6ta00237d
Reddy, B. N., Kumar, P. N., and Deepa, M. (2015). A Poly (3,4-ethylenedioxypyrrole)-Au@WO3-based electrochromic pseudocapacitor. ChemPhysChem. 16, 377–389. doi: 10.1002/cphc.201402625
Shinde, P. A., Lokhande, A. C., Chodankar, N. R., Patil, A. M., Jin, H. K., and Lokhande, C. D. (2017). Temperature dependent surface morphological modifications of hexagonal WO3 thin films for high performance supercapacitor application. Electrochim. Acta 224, 397–404. doi: 10.1016/j.electacta.2016.12.066
Shinde, P. A., Lokhande, V. C., Chodankar, N. R., Ji, T., Kimc, J. H., and Lokhande, C. D. (2016). Enhanced electrochemical performance of monoclinic WO3 thin film with redox additive aqueous electrolyte. J. Colloid Interface Sci. 483. 261–267. doi: 10.1016/j.jcis.2016.08.011
Sun, P., Deng, Z., Yang, P., Yu, X., Chen, Y., Liang, Z., et al. (2015). Freestanding CNT-WO3 hybrid electrodes for flexible asymmetric supercapacitors. J. Mater. Chem. 3, 12076–12080. doi: 10.1039/c5ta02316e
Thind, S. S., Chang, X., Wentzell, J. S., and Chen, A. (2016). High-performance supercapacitor based on tantalum iridium oxides supported on tungsten oxide nanoplatelets. Electrochem. Commun. 67, 1–5. doi: 10.1016/j.elecom.2016.03.002
Wang, C.-H., Wen, W.-C., Hsu, H.-C., Yao, B.-Y., et al. (2016). High-capacitance KOH-activated nitrogen-containing porous carbon material from waste coffee grounds in supercapacitor. Adv. Polym. Technol. 27, 1387–1395. doi: 10.1016/j.apt.2016.04.033
Wang, F., Zhan, X., Cheng, Z., Wang, Z., Wang, Q., Xu, K., et al. (2015). Tungsten oxide@polypyrrole core-shell nanowire arrays as novel negative electrodes for asymmetric supercapacitors. Small 11, 749–755. doi: 10.1002/smll.201402340
Wang, J. G., Liu, H., Zhang, X., Li, X., Liu, X., Kang, F., et al. (2018). Green synthesis of hierarchically porous carbon nanotubes as advanced materials for high-efficient energy storage. Small 14:e1703950. doi: 10.1002/smll.201703950
Wang, K., Wu, H., Meng, Y., and Wei, Z. (2014). Conducting polymer nanowire arrays for high performance supercapacitors. Small 10, 14–31. doi: 10.1002/smll.201301991
Wang, R., Wang, P., Yan, X., Lang, J., Chao, P., and Xue, Q. (2012). Promising porous carbon derived from celtuce leaves with outstanding supercapacitance and CO2 capture performance. ACS Appl. Mater. Interfaces 4, 5800–5806. doi: 10.1021/am302077c
Wang, W., Wang, X., Wang, X., Yang, L., Wu, Z., Xia, S., et al. (2013). Cr (VI) removal from aqueous solution with bamboo charcoal chemically modified by iron and cobalt with the assistance of microwave. J. Environ. Sci. 25, 1726–1735. doi: 10.1016/s1001-0742(12)60247-2
Wang, Y. H., Wang, C. C., Cheng, W. Y., and Lu, S. Y. (2014). Dispersing WO3 in carbon aerogel makes an outstanding supercapacitor electrode material. Carbon 69, 287–293. doi: 10.1016/j.carbon.2013.12.027
Wu, X., and Yao, S. (2017). Flexible electrode materials based on WO3 nanotube bundles for high performance energy storage devices. Nano Energy 42, 143–150. doi: 10.1016/j.nanoen.2017.10.058
Xiao, X., Ding, T., Yuan, L., Shen, Y., Zhong, Q., Zhang, X., et al. (2012). WO3−x /MoO3−x core/shell nanowires on carbon fabric as an anode for all-solid-state asymmetric supercapacitors. Adv. Energy Mater. 2, 1328–1332. doi: 10.1002/aenm.201200380
Xing, L. L., Huang, K. J., and Fang, L. X. (2016). Preparation of layered graphene and tungsten oxide hybrids for enhanced performance supercapacitors. Dalton Trans. 45, 17439–17446. doi: 10.1039/c6dt03719d
Xu, J., Li, Y., Wang, L., Cai, Q., Li, Q., Gao, B., et al. (2016). High-energy lithium-ion hybrid supercapacitors composed of hierarchical urchin-like WO3/C anodes and MOF-derived polyhedral hollow carbon cathodes. Nanoscale 8, 16761–16768. doi: 10.1039/c6nr05480c
Xu, L., Zhao, Y., Lian, J., Xu, Y., Bao, J., Qiu, J., et al. (2017). Morphology controlled preparation of ZnCo2O4 nanostructures for asymmetric supercapacitor with ultrahigh energy density. Energy 123, 296–304, doi: 10.1016/j.energy.2017.02.018
Yang, C. S., Jang, Y. S., and Jeong, H. K. (2014). Bamboo-based activated carbon for supercapacitor applications. Curr. Appl. Phys. 14, 1616–1620. doi: 10.1016/j.cap.2014.09.021
Yao, C., Wei, B. B., Li, H. H., Wang, G. F., Han, Q., Ma, H., et al. (2017). Carbon-encapsulated tungsten oxide nanowires as stable and high-rate anode for flexible asymmetric supercapacitors. J. Mater. Chem. 5. 51–56. doi: 10.1010.1039/C6TA08274B
Yao, S., Zheng, X., Zhang, X., Xiao, H., Qu, F., Wu, X., et al. (2017). Facile synthesis of flexible WO3 nanofibers as supercapacitor electrodes. Mater. Lett. 186, 94–97. doi: 10.1016/j.matlet.2016.09.085
Yi, Z., Xu, X., Kang, X., Zhao, Y., Zhang, S., Yao, W., et al. (2017). Fabrication of well-aligned ZnO@Ag nanorod arrays with effective charge transfer for surface-enhanced Raman scattering. Surf. Coat. Technol. 324, 257–263, doi: 10.1016/j.surfcoat.2017.05.084
Yu, S., Liu, D., Zhao, S., Bao, B., Jin, C., Huang, W., et al. (2015). Synthesis of wood derived nitrogen-doped porous carbon–polyaniline composites for supercapacitor electrode materials. Rsc Adv. 5, 30943–30949. doi: 10.1039/C5RA01949D
Yuksel, R., Durucan, C., and Unalan, H. E. (2016). Ternary nanocomposite SWNT/WO3/PANI thin film electrodes for supercapacitors. J. Alloys Compd. 658, 183–189. doi: 10.1016/j.jallcom.2015.10.216
Zeng, Z., Liu, Y., Zhang, W., Chevva, H., and Wei, J. (2017). Improved supercapacitor performance of MnO2-electrospun carbon nanofibers electrodes by mT magnetic field. J. Power Sources 358, 22–28. doi: 10.1016/j.jpowsour.2017.05.008
Zhang, Q., Zhao, B., Wang, J., Qu, C., Sun, H., Zhang, K., et al. (2012). High-performance hybrid supercapacitors based on self-supported 3D ultrathin porous quaternary Zn-Ni-Al-Co oxide nanosheets. Nano Energy 28, 475–485. doi: 10.1016/j.nanoen.2016.08.049
Zhang, Y., and Park, S. J. (2017). Incorporation of RuO2 into charcoal-derived carbon with controllable microporosity by CO2 activation for high-performance supercapacitor. Carbon 122, 287–297. doi: 10.1016/j.carbon.2017.06.085
Zhang, Y., Yao, Q. Q., Gao, H. L., Zhang, L. S., Wang, L. Z., Zhang, A. Q., et al. (2015). Synthesis and electrochemical performance of MnO2 /BC composite as active materials for supercapacitors. J. Anal. Appl. Pyrolysis 111, 233–237. doi: 10.1016/j.jaap.2014.11.002
Zhang, Z., Wang, X., Wang, Y., Xia, S., Chen, L., Zhang, Y., et al. (2013). Pb (II) removal from water using Fe-coated bamboo charcoal with the assistance of microwaves. J. Environ. Sci. 25, 1044–1053. doi: 10.1016/S1001-0742(12)60144-2
Zhao, B., Zhang, L., Zhang, Q., Chen, D., Chen, Y., Deng, X., et al. (2017). Rational design of nickel hydroxide-based nanocrystals on graphene for ultrafast energy storage. Adv. Energy Mater. 2017:1702247. doi: 10.1002/aenm.201702247
Zhao, Y., Hu, L., Zhao, S., and Wu, L. (2016). Preparation of MnCo2O4@Ni(OH)2 core–shell flowers for asymmetric supercapacitor materials with ultrahigh specific capacitance. Adv. Funct. Mater. 26, 4085–4093. doi: 10.1002/adfm.201600494
Zhao, Y., Xu, L., Yan, J., Yan, W., Wu, C., Lian, J., et al. (2017). Facile preparation of NiFe2O4/MoS2 composite material with synergistic effect for high performance supercapacitor. J. Alloy. Compds. 726, 608–617. doi: 10.1016/j.jallcom.2017.07.327
Zhao, Y., Yuan, M., Chen, Y., Yan, J., Xu, L., Huang, Y., et al. (2018). Construction of molybdenum dioxide nanosheets coated on the surface of nickel ferrite nanocrystals with ultrahigh specific capacity for hybrid supercapacitor. Electrochimica Acta 260, 439–448. doi: 10.1016/j.electacta.2017.12.053
Zhong, M., Fu, Z., Huang, W., Yang, X., and Liu, M. (2015). Fabrication of porous Pd/C microspheres in carbon aerogels by radiation method. Mater. Lett. 139, 314–317. doi: 10.1016/j.matlet.2014.10.098
Zhong, M., Fu, Z., Huang, W., Yang, X., and Liu, M. (2016). A solution-phase synthesis method to prepare Pd doped carbon aerogels for hydrogen storage. RSC Adv. 5, 20966–20971. doi: 10.1039/c4ra16505e
Keywords: γ-irradiation method, WO3·2H2O/BC hybrids, higher specific capacitance, cyclic stability, supercapacitors
Citation: Yang F, Jia J, Mi R, Liu X, Fu Z, Wang C, Liu X and Tang Y (2018) Fabrication of WO3·2H2O/BC Hybrids by the Radiation Method for Enhanced Performance Supercapacitors. Front. Chem. 6:290. doi: 10.3389/fchem.2018.00290
Received: 06 May 2018; Accepted: 25 June 2018;
Published: 13 August 2018.
Edited by:
Qiaobao Zhang, Xiamen University, ChinaCopyright © 2018 Yang, Jia, Mi, Liu, Fu, Wang, Liu and Tang. This is an open-access article distributed under the terms of the Creative Commons Attribution License (CC BY). The use, distribution or reproduction in other forums is permitted, provided the original author(s) and the copyright owner(s) are credited and that the original publication in this journal is cited, in accordance with accepted academic practice. No use, distribution or reproduction is permitted which does not comply with these terms.
*Correspondence: Xudong Liu, OHNsaXV4dWRvbmdAY2FlcC5jbg==
Yongjian Tang, dGFuZ3lvbmdqaWFuMjAwMEBzaW5hLmNvbQ==
†These authors have contributed equally to this work