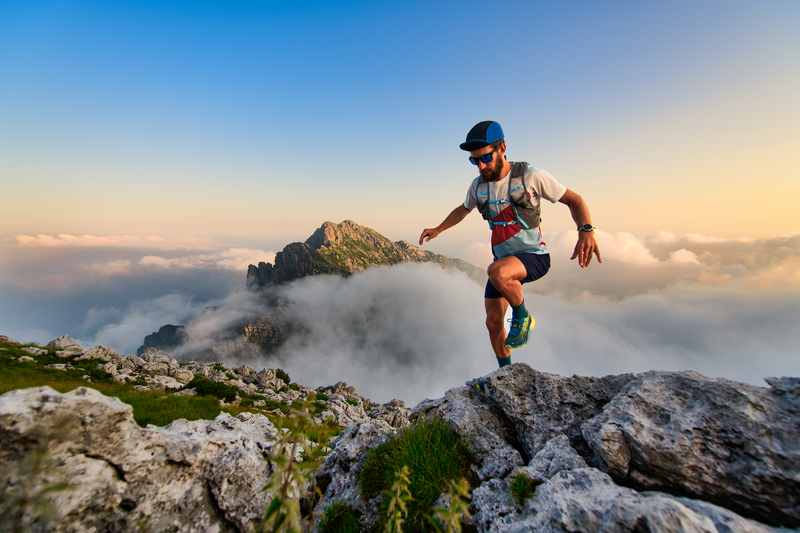
94% of researchers rate our articles as excellent or good
Learn more about the work of our research integrity team to safeguard the quality of each article we publish.
Find out more
MINI REVIEW article
Front. Cell Dev. Biol. , 15 September 2020
Sec. Cell Adhesion and Migration
Volume 8 - 2020 | https://doi.org/10.3389/fcell.2020.588152
This article is part of the Research Topic The Extracellular Environment in Controlling Neuronal Migration During Neocortical Development View all 15 articles
During development of the cerebral cortex, different types of neurons migrate from distinct origins to create the different cortical layers and settle within them. Along their way, migrating neurons use cell adhesion molecules on their surface to interact with other cells that will play critical roles to ensure that migration is successful. Radially migrating projection neurons interact primarily with radial glia and Cajal-Retzius cells, whereas interneurons originating in the subpallium follow a longer, tangential route and encounter additional cellular substrates before reaching the cortex. Cell-cell adhesion is therefore essential for the correct migration of cortical neurons. Several members of the cadherin superfamily of cell adhesion proteins, which mediate cellular interactions through calcium-dependent, mostly homophilic binding, have been shown to play important roles during neuronal migration of both projection neurons and interneurons. Although several classical cadherins and protocadherins are involved in this process, the most prominent is CDH2. This mini review will explore the cellular and molecular mechanisms underpinning cadherin function during cortical migration, including recent advances in our understanding of the control of adhesive strength through regulation of cadherin surface levels.
Whilst cellular movements are an essential developmental feature of most organs, the orchestration of such movements in the nervous system is particularly relevant, as much of the central nervous system is organized in distinct layers that typically share functional properties. In the brain, neurons are generated in proliferative areas close to the ventricles and subsequently migrate to reach their definitive positions in different regions. Neocortical projection neurons migrate radially from the pallial ventricular or subventricular zone (Haubensak et al., 2004; Noctor et al., 2004), whereas cortical interneurons migrate tangentially from the ventral telencephalon (de Carlos et al., 1996; Anderson et al., 1997; reviewed in Marín and Rubenstein, 2001; Ayala et al., 2007; see also Figure 1). During their journey, they all navigate through complex extracellular environments that include other cells, which play critical roles in ensuring a successful migration. Interactions between Cajal-Retzius cells in the marginal zone of the cortex and migrating projection neurons are crucial for somal and terminal translocation (Gil-Sanz et al., 2013). Likewise, locomoting neurons use the basal processes of radial glia progenitors as a scaffold to migrate along (Rakic, 1972). Contact between migrating neurons and their cellular substrates is mediated by different cell-cell adhesion molecules, including the cadherin superfamily, with over 100 members expressed preferentially or exclusively in the nervous system in vertebrates. Cadherins are calcium-dependent cell-cell adhesion molecules, characterized by the presence of a variable number of extracellular cadherin repeats, that can be broadly subdivided into three main subfamilies (Sotomayor et al., 2014): the classical cadherins and the clustered and non-clustered protocadherins. Members of all three subfamilies have been shown to play a role in cortical migration. Alpha clustered protocadherins regulate radial migration through a pathway involving WAVE, Pyk2 kinase and the small GTPase Rac1 (Fan et al., 2018). Interfering with protocadherins DCHS1 and FAT4 also affects neuronal positioning (Cappello et al., 2013) and leads to migration defects in human projection neurons (Klaus et al., 2019). Protocadherin 20 determines the final position of layer 4 neurons in mice (Oishi et al., 2016), while another protocadherin, Celsr3, is required for interneuron migration (Ying et al., 2009). However, the cadherin with the best characterized role in cortical migration is the classical cadherin CDH2 (N-cadherin), which will therefore be the focus of this review.
Figure 1. Molecular mechanisms of CDH2 during the different steps of radial neuronal migration. Projection neurons are born from Radial Glia progenitors (gray cell, RGC) in the ventricular zone (VZ) or from intermediate progenitors in the subventricular zone (SVZ). They undergo multipolar migration while in the SVZ and intermediate zone (IZ) (step 1, brown cell), before developing a leading process and an axon, and acquiring bipolar morphology. They enter the cortical plate (CP) as bipolar cells and use the processes of the Radial Glia progenitors as scaffold to migrate along (step 2, green cell). Once they are close enough to the marginal zone (MZ) for their leading process to make contact with RGC endfeet and Cajal-Retzius cells (CR cell), neurons switch to terminal translocation for the last phase of their migration (step 3, blue cell). Early born neurons do not need a locomoting step because the cortical wall is still very thin at the time of their migration, so they ascend directly through somal translocation after becoming bipolar. Box 1a shows the molecular mechanisms regulating CDH2 total or surface expression during multipolar migration and multipolar to bipolar transition (step 1), while box 1b shows downstream effects of CDH2 at this stage. Similarly, boxes 2a and 2b show regulation of CDH2 and downstream effects during glia-guided locomotion (step 2), and box 3 shows regulation of CDH2 surface levels during somal translocation (step 3). For details, see text.
After initial bipolar migration to the subventricular zone and lower intermediate zone, newborn neurons adopt a multipolar morphology before developing leading and trailing processes and becoming bipolar in the upper intermediate zone to progress into the cortical plate (Nadarajah et al., 2001; Tabata and Nakajima, 2003; Noctor et al., 2004). The multipolar to bipolar (MBP) transition is a crucial step that involves many different proteins (Cooper, 2014). Failure to form a leading process impedes migration into the cortical plate, which proceeds first through somal translocation for early born neurons and then by glia-guided locomotion (Kawauchi, 2015) followed by terminal translocation as the cortical wall grows in thickness (Nadarajah et al., 2001; Figure 1). Interneurons first migrate tangentially from the ganglionic eminences into the cortex and subsequently switch to radial migration to invade the cortical plate (Marín and Rubenstein, 2001).
CDH2 has been involved in most of these steps. As one of the main components of adherens junctions keeping radial glia endfeet connected (Kadowaki et al., 2007), CDH2 first needs to be downregulated to allow detachment of newborn neurons from the adherens junction belt and their subsequent delamination (Rousso et al., 2012). Once neurons reach the subventricular zone/intermediate zone, CDH2 is needed for correct polarization in the radial direction and for the specification of the leading and trailing processes (Jossin and Cooper, 2011; Gärtner et al., 2012; Xu et al., 2015). Further to its role in MBP transition, CDH2-mediated adhesion between migrating neurons and radial glia fibers is needed for the locomoting phase (Shikanai et al., 2011), with adhesion sites providing traction for the forward movement of the nucleus (Martinez-Garay et al., 2016). Similarly, attachment of the leading process to radial glia endfeet and Cajal-Retzius cells in the marginal zone for somal or terminal translocation is also dependent on CDH2 (Franco et al., 2011; Gil-Sanz et al., 2013). The following sections will focus on the molecular mechanisms known to operate around CDH2 during those different roles, with a significant part of the review devoted to regulation of CDH2 surface levels, as this topic has been more extensively studied.
The strength of cadherin-mediated adhesions depends mainly on the levels of cadherins at the cell surface. These levels are, in turn, regulated by the balance between the rate of biosynthesis and of delivery to the plasma membrane, and turnover of cadherin molecules by endocytic processes. Recent reviews provide a comprehensive account of cadherin trafficking (Cadwell et al., 2016; West and Harris, 2016), so this section will mainly focus on the specifics of CDH2 regulation in migrating neurons (Table 1).
Newly synthesized CDH2 in the endoplasmic reticulum binds to β-catenin and p120-catenin (Wahl et al., 2003), which regulates subsequent transport to the Golgi and the plasma membrane through interaction with kinesin motors (Mary et al., 2002; Chen et al., 2003; Teng et al., 2005; Wehrendt et al., 2016). Internalization of surface CDH2 is mediated by clathrin-dependent and independent endocytic mechanisms (Sharma and Henderson, 2007; Tai et al., 2007; Chen and Tai, 2017) and binding of β-catenin and p120-catenin to CDH2 regulates this process, partly by masking key residues needed for endocytosis (Chen and Tai, 2017). Internalized receptors can be either recycled back to the cell membrane or routed to the lysosome for degradation in an endosomal sorting pathway mainly controlled by Rab GTPases and their effectors (Wandinger-Ness and Zerial, 2014).
An intriguing possibility to further modulate cadherin adhesion is allosteric regulation. This form of inside-out signaling, well characterized in integrin-mediated adhesion (Hynes, 2002), has so far been demonstrated for CDH1 (Shashikanth et al., 2015), but whether it could provide a way to quickly control CDH2 adhesive strength is not yet known.
In migrating neurons, one way to adjust CDH2 levels is through regulation of Cdh2 mRNA. FMRP binds Cdh2 mRNA and in Fmr1–/– animals, which display increased numbers of multipolar neurons in the intermediate zone, Cdh2 mRNA levels are reduced and the migration defect is rescued by overexpression of CDH2 (La Fata et al., 2014).
Similarly, downregulation of the small GTPase Rab23 also leads to a decrease of CDH2 levels in newborn neurons (Hor and Goh, 2018). This reduction, which is apparent at contact surfaces between interacting neurons, affects both the full-length protein and its processed cytoplasmic fragment, and is accompanied by a decrease in mRNA levels. Phenotypically, it leads to an increase in the number of multipolar neurons in the intermediate zone that fail to progress into the cortical plate. At the molecular level, diminished Rab23 levels seem to impair activation of PDGFRα and its subsequent phosphorylation of ERK1/2; indeed, pharmacological inhibition of ERK1/2 also led to a reduction in CDH2 levels (Hor and Goh, 2018).
Another Rab GTPase involved in the control of total CDH2 levels is Rab18. Knockdown of this GTPase or interference with its function leads to a migration defect in the intermediate zone that can also be partially rescued by overexpression of CDH2. In this case, reduction of surface CDH2 levels as revealed by TIRF microscopy are correlated with a global decrease in CDH2 through lysosomal degradation rather than protein expression (Wu et al., 2016).
In addition to adjusting global CDH2 levels, migrating neurons also dynamically regulate CDH2 surface levels through several pathways, allowing for more flexible responses to varying extracellular environments (Solecki, 2012; Figures 1,1a,2a,3).
As main regulators of endosomal trafficking, Rab GTPases are ideal candidates to control CDH2 surface levels. Kawauchi et al showed that Rab11 and Rab5 play opposing but essential roles in the trafficking of CDH2 to and from the plasma membrane (Kawauchi et al., 2010; Kawauchi, 2011; Figures 1,1a,2a). Knockdown of Rab5 reduces Rab5-mediated endocytosis, leading to an accumulation of cells in the intermediate zone with abnormal morphology and increased adhesion between neurons and radial glia processes. This migration defect is partially rescued through slight downregulation of CDH2 levels, implicating Rab5 in the internalization of CDH2 (Kawauchi et al., 2010). This same study showed that Rab11 is needed for correct recycling of CDH2 back to the cell membrane after endocytosis. Electroporation of dominant negative (DN)-Rab11 results in redistribution of CDH2 from the cell surface to perinuclear regions and its accumulation in transferrin positive vesicular compartments, concomitant with a delay in radial migration.
Two other proteins have been shown to regulate CDH2 surface levels through an interplay with Rab11. Lack of HTT, which is expressed in the upper intermediate zone and the cortical plate, results in migration defects with neurons failing to acquire a bipolar morphology and showing abnormal interaction with radial glia fibers. HTT and CDH2 normally co-localize in the leading process of migrating neurons, but in the absence of HTT, CDH2 is relocated to transferrin positive vesicles in the perinuclear region and CDH2 surface levels are significantly reduced. Interestingly, co-expression of Rab11 or its constitutively active form rescues the migration defect of HTT depleted cells, as does overexpression of CDH2 (Barnat et al., 2016; Figures 1,1a).
The small GTPase Arf6 localizes to a subpopulation of early and recycling endosomes and interfering with its function disrupts migration and increases intracellular CDH2. This increase is not due to changes in the internalization of CDH2, but rather to its defective recycling back to the cell surface. Only Arf6 capable of interacting with its effector FIP3 and with Rab11 can rescue the migration defect of Arf6 knockdown (Hara et al., 2016; Figures 1,1a).
The small GTPase Rap1 is one of the major regulators of CDH2 surface levels, both during multipolar migration and the MBP transition, and during somal and terminal translocation (Figures 1,1a,3). Jossin and Cooper showed that interfering with normal Rap1 function delays the progression of neurons to a bipolar state (Jossin and Cooper, 2011). This cell-autonomous defect is due to reduced surface levels of CDH2 and can be rescued by CDH2 overexpression. The molecular mechanism linking Rap1 to CDH2 involves Vav2, an activator for Rac1, and other small GTPases (RalA/B and Rac1/Cdc42). Interestingly, reelin signaling acts upstream of Rap1 to regulate CDH2 membrane levels in the intermediate zone, as it does in the upper cortical plate during somal or terminal translocation (Franco et al., 2011; Jossin and Cooper, 2011). In translocating neurons, reelin activates Rap1 through RapGEF1 (C3G) (Franco et al., 2011), but CDH2 recruitment to the cell surface is dependent on nectin-mediated adhesion between migrating neurons and Cajal-Retzius cells (Gil-Sanz et al., 2013). CDH2 overexpression rescues migration defects caused by downregulation of nectin 3 or its effector Afadin, which interacts with Rap1 and p120 catenin, providing a link between Rap1 activation and CDH2 (Gil-Sanz et al., 2013; Figures 1,3). Recently, the reelin-induced increase in CDH2 surface levels in translocating neurons has been shown to be transient rather than sustained, but the mechanism behind it remains unknown (Matsunaga et al., 2017).
Another molecule acting upstream of Rap1 to regulate CDH2 surface levels is CDK5. Despite initial reports of CDK5 negatively regulating cadherin-mediated adhesion and the interaction between β-catenin and CDH2 (Kwon et al., 2000), this negative effect of CDK5 on CDH2 adhesion has not been reproduced in vivo. CDK5 phosphorylates RapGEF2 in the developing cortex, enhancing its GEF activity toward Rap1 (Ye et al., 2014). RapGEF2 is strongest expressed in the upper intermediate zone and is required for MBP transition, since its knockdown leads to multipolar neurons that accumulate in the lower intermediate zone. Membrane CDH2 levels are reduced in the Cdk5–/– cortex and in neurons electroporated with either shRNA against RapGEF2, or its non-phosphorylatable form S1124A, and migration defects caused by RapGEF2 inhibition can be rescued by moderate overexpression of CDH2 (Ye et al., 2014). Similar results were reported in a recent study that looked at the link between CDK5 and CDH2 in the developing cortex, which showed that migration defects caused by in utero electroporation of DNCDK5 at E14.5 could be partially rescued by co-electroporation with CDH2 (Lee et al., 2019; Figures 1,1a).
In addition to small GTPases, other proteins linked to endocytosis and the actin cytoskeleton are involved in the control of CDH2 surface levels in migrating neurons. The actin motor MYO10 interacts with CDH2 through its FERM domain and seems to mediate its transport from the Golgi to the plasma membrane. Downregulation of this unconventional myosin reduces surface CDH2, but not its total levels. This leads to accumulation of cells in the intermediate zone that display disrupted interaction with radial glia fibers and decreased locomoting speed in those neurons that make it to the cortical plate. MYO10 also colocalizes with markers for early, late and recycling endosomes, suggesting that it might play a role in the trafficking of CDH2-containing endosomes (Lai et al., 2015). Drebrin-like (DBNL) is an adaptor protein that binds F-actin and Dynamin 1 and is thus involved in receptor-mediated endocytosis and remodeling of the actin cytoskeleton. As in the case of MYO10, knockdown of DBNL reduces CDH2 levels at the cell surface. How this reduction is brought about at the molecular or cellular level is not known, but it seems to involve phosphorylation of two Tyr residues in Dbnl by Fyn. Dbnl-deficient neurons complete the MBP transition despite defects in neurite extension and polarization, but do not enter the cortical plate (Inoue et al., 2019). In both cases, overexpression of CDH2 partially rescues the migration defects (Figures 1,1a).
Despite neurons not displaying caveolae, caveolin 1 is expressed in the developing cortex, particularly in the neurites of multipolar cells in the intermediate zone, where it is involved in clathrin-independent endocytosis. Downregulation of caveolin 1 increases the ratio of surface to total levels of two adhesion proteins: CDH2 and L1CAM, while decreasing their levels in early endosomes, suggesting that caveolin 1 is needed for their internalization (Shikanai et al., 2018). Neurons deficient in caveolin 1 acquire bipolar morphology, but their leading processes are shorter and more branched than in control neurons, with increased immature neurites that are retained even after leading process formation (Figures 1,1a).
Compared to the wealth of information about the regulation of CDH2 surface levels in migrating neurons, much less is known about the mechanisms operating downstream of CDH2. With regards to the initial specification of neuronal processes, in vitro experiments suggest that opposing gradients of active RhoA at the leading process and Rac1 in the axon are established as a consequence of CDH2-mediated contact (Xu et al., 2015). Although the exact mechanism by which this is accomplished remains unclear, work in C2C12 fibroblasts has shown that CDH2 engagement decreases Rac1 and Cdc42 activity and increases RhoA activity (Charrasse et al., 2002). This could explain the formation of the gradients, as sites of CDH2 adhesion between neurons and radial glia fibers provide a positional cue for the development of the leading process while directing axonal formation to the opposite pole of the cell (Gärtner et al., 2012; Xu et al., 2015; Figures 1,1b).
A second mechanism at play during CDH2-mediated neuronal polarization involves its interaction with FGFRs to prevent their ubiquitination and subsequent lysosomal degradation. This interaction happens in cis and, surprisingly, does not require CDH2-mediated adhesion. As a result of higher levels of FGFR at the cell membrane, the ERK1/2 signaling pathway is activated. Since similar results in ERK1/2 activation are obtained by long treatment with reelin in vitro, FGFR and ERK1/2 can be considered downstream components of the reelin – Rap1 – CDH2 axis (Kon et al., 2019; Figures 1,1b).
Glia-guided locomotion requires CDH2-mediated adhesion and its connection to the actin cytoskeleton through alpha-N-catenin. When CDH2 (and CDH4) adhesion is weakened by electroporation of different dominant negative forms, neurons can still polarize and extend leading processes into the cortical plate. However, nucleokinesis fails and the leading processes become twice as long as in control neurons. The collapse of the processes upon induced actomyosin contraction indicates that CDH2-mediated contacts between neurons and radial glia fibers probably act as sites for traction generation to allow nuclear movement. In addition, neurons electroporated with DNCDH also show an abnormal accumulation of LIS1 in the leading process, hinting to a potential mechanism involving microtubules downstream of CDH2 in migrating neurons (Martinez-Garay et al., 2016; Figures 1,2b).
CDH2 is also involved in the generation and migration of cortical interneurons. As well as maintaining the organization of the neuroepithelium through adherens junctions, CDH2 engagement stimulates interneuron motility (Luccardini et al., 2013). Interneurons expressing DNCDH in vitro show disrupted centrosome dynamics and non-muscle myosin IIB localization, while complete elimination of CDH2 reduces their migration speed and impairs polarity. In vivo, interneurons lacking CDH2 are less efficient in leaving the medial ganglionic eminence and reaching the cortex, as well as in invading the cortical plate (Luccardini et al., 2013, 2015). Interestingly, the effect of CDH2 on the ability of interneurons to migrate to the cortex and colonize it seems to be cell type specific, as knockout of CDH2 in Dlx5/6 expressing cells selectively reduces the numbers of calretinin and somatostatin positive interneurons, but does not alter other interneuronal types (László et al., 2020). However, the molecular mechanisms underpinning the role of CDH2 in interneuron migration remain to be elucidated.
The involvement of CDH2 in every step of radial migration and its function in interneurons underscore the importance of this adhesion molecule in mediating cell-cell interactions during cortical development. However, we still have a fragmented view with different observations that need to be integrated to provide a full picture of CDH2 function during neuronal migration. It is still not known if and how the different players regulating CDH2 levels are coordinated. For example, it remains to be determined whether Rap1 and Rab GTPases act in parallel pathways of if they cooperate to regulate CDH2 surface levels. The molecular signals activated upon CDH2 adhesion are also poorly understood, and the fact that mechanisms downstream of cadherins seem to be context specific means that caution should be exerted when extrapolating from different cellular systems and assumptions should be experimentally verified. It is important to keep in mind that the timepoint of intervention, dependent on age at electroporation but also on the use of different promoters, will influence results. The timing until analysis is also important because delays in polarization might mask roles in later migration phases, and the use of different dominant negative cadherin forms, sometimes at different concentrations, will also impact the phenotypes observed. These factors might explain why locomotion was considered relatively independent of CDH2 in one study (Jossin and Cooper, 2011), while being shown to be needed for this process in others (Shikanai et al., 2011; Martinez-Garay et al., 2016). Similarly, the requirement for CDH2 adhesion during MBP transition is questioned by a recent study (Kon et al., 2019), but this might reflect separate functions of CDH2 at slightly different timepoints during this complex process. Another controversy that might be explained, at least in part, by different experimental conditions is the fact that although reelin-mediated Rap1 activation seems to be required for MBP transition (Jossin and Cooper, 2011), Dab1 deficient neurons polarize correctly, enter the cortical plate and only show defects in somal translocation (Franco et al., 2011).
A final open question is the extent to which CDH2 cooperates with other adhesion molecules. Beyond its cooperation with nectins during somal translocation, no equivalent mechanism has been described for other migration phases. Connexins 43 and 26 also provide adhesion between cortical migrating neurons and radial glia fibers (Elias et al., 2007) and, interestingly, Cx43 directly downregulates CDH2 transcription during neural crest cell migration (Kotini et al., 2018). In addition, CDH2 binds astrotactin in cerebellar migration (Horn et al., 2018), raising the possibility of a similar function in the cortex. These examples highlight the potential for functional interactions between adhesion proteins and the need to expand our studies beyond individual molecules.
IM-G wrote the manuscript and created the figure and table.
IM-G was supported by funding from the BBSRC (BB/S0023 59/1).
The author declares that the research was conducted in the absence of any commercial or financial relationships that could be construed as a potential conflict of interest.
I would like to thank the members of the Martinez-Garay lab and Prof. Yves A. Barde for proofreading the manuscript and providing useful comments.
Anderson, S. A., Eisenstat, D. D., Shi, L., and Rubenstein, J. L. (1997). Interneuron migration from basal forebrain to neocortex: dependence on Dlx genes. Science 278, 474–476. doi: 10.1126/science.278.5337.474
Ayala, R., Shu, T., and Tsai, L.-H. (2007). Trekking across the brain: the journey of neuronal migration. Cell 128, 29–43. doi: 10.1016/j.cell.2006.12.021
Barnat, M., Le Friec, J., Benstaali, C., and Humbert, S. (2016). Huntingtin-mediated multipolar-bipolar transition of newborn cortical neurons is critical for their postnatal neuronal morphology. Neuron 93, 99–114. doi: 10.1016/j.neuron.2016.11.035
Cadwell, C. M., Su, W., and Kowalczyk, A. P. (2016). Cadherin tales: regulation of cadherin function by endocytic membrane trafficking. Traffic 17, 1262–1271. doi: 10.1111/tra.12448
Cappello, S., Gray, M. J., Badouel, C., Lange, S., Einsiedler, M., Srour, M., et al. (2013). Mutations in genes encoding the cadherin receptor-ligand pair DCHS1 and FAT4 disrupt cerebral cortical development. Nat. Genet. 45, 1300–1308. doi: 10.1038/ng.2765
Charrasse, S., Meriane, M., Comunale, F., Blangy, A., and Gauthier-Rouvière, C. (2002). N-cadherin-dependent cell-cell contact regulates Rho GTPases and beta-catenin localization in mouse C2C12 myoblasts. J. Cell Biol. 158, 953–965. doi: 10.1083/jcb.200202034
Chen, X., Kojima, S.-I., Borisy, G. G., and Green, K. J. (2003). p120 catenin associates with kinesin and facilitates the transport of cadherin-catenin complexes to intercellular junctions. J. Cell Biol. 163, 547–557. doi: 10.1083/jcb.200305137
Chen, Y. T., and Tai, C.-Y. (2017). μ2-dependent endocytosis of N-cadherin is regulated by β-catenin to facilitate neurite outgrowth. Traffic 18, 287–303. doi: 10.1111/tra.12473
Cooper, J. A. (2014). Molecules and mechanisms that regulate multipolar migration in the intermediate zone. Front. Cell. Neurosci. 8:386. doi: 10.3389/fncel.2014.00386
de Carlos, J. A., López-Mascaraque, L., and Valverde, F. (1996). Dynamics of cell migration from the lateral ganglionic eminence in the rat. J. Neurosci. 16, 6146–6156. doi: 10.1523/JNEUROSCI.16-19-06146.1996
Elias, L. A. B., Wang, D. D., and Kriegstein, A. R. (2007). Gap junction adhesion is necessary for radial migration in the neocortex. Nature 448, 901–907. doi: 10.1038/nature06063
Fan, L., Lu, Y., Shen, X., Shao, H., Suo, L., and Wu, Q. (2018). Alpha protocadherins and Pyk2 kinase regulate cortical neuron migration and cytoskeletal dynamics via Rac1 GTPase and WAVE complex in mice. eLife 7:e35242.
Franco, S. J., Martinez-Garay, I., Gil-Sanz, C., Harkins-Perry, S. R., and Müller, U. (2011). Reelin regulates cadherin function via Dab1/Rap1 to control neuronal migration and lamination in the neocortex. Neuron 69, 482–497. doi: 10.1016/j.neuron.2011.01.003
Gärtner, A., Fornasiero, E. F., Munck, S., Vennekens, K., Seuntjens, E., Huttner, W. B., et al. (2012). N-cadherin specifies first asymmetry in developing neurons. EMBO J. 31, 1893–1903. doi: 10.1038/emboj.2012.41
Gil-Sanz, C., Franco, S. J., Martinez-Garay, I., Espinosa, A., Harkins-Perry, S., and Müller, U. (2013). Cajal-retzius cells instruct neuronal migration by coincidence signaling between secreted and contact-dependent guidance cues. Neuron 79, 461–477. doi: 10.1016/j.neuron.2013.06.040
Hara, Y., Fukaya, M., Hayashi, K., Kawauchi, T., Nakajima, K., and Sakagami, H. (2016). ADP ribosylation factor 6 regulates neuronal migration in the developing cerebral cortex through FIP3/Arfophilin-1-dependent endosomal trafficking of N-cadherin. eNeuro 3:ENEURO.0148-16. doi: 10.1523/ENEURO.0148-16.2016
Haubensak, W., Attardo, A., Denk, W., and Huttner, W. B. (2004). Neurons arise in the basal neuroepithelium of the early mammalian telencephalon: a major site of neurogenesis. Proc. Natl. Acad. Sci. U.S.A. 101, 3196–3201. doi: 10.1073/pnas.0308600100
Hor, C. H. H., and Goh, E. L. K. (2018). Rab23 regulates radial migration of projection neurons via N-cadherin. Cereb. Cortex 28, 1516–1531. doi: 10.1093/cercor/bhy018
Horn, Z., Behesti, H., and Hatten, M. E. (2018). N-cadherin provides a cis and trans ligand for astrotactin that functions in glial-guided neuronal migration. Proc. Natl. Acad. Sci. U.S.A. 115, 10556–10563. doi: 10.1073/pnas.1811100115
Inoue, S., Hayashi, K., Fujita, K., Tagawa, K., Okazawa, H., Kubo, K.-I., et al. (2019). Drebrin-like (Dbnl) controls neuronal migration via regulating N-cadherin expression in the developing cerebral cortex. J. Neurosci. 39, 678–691. doi: 10.1523/JNEUROSCI.1634-18.2018
Jossin, Y., and Cooper, J. A. (2011). Reelin, Rap1 and N-cadherin orient the migration of multipolar neurons in the developing neocortex. Nat. Neurosci. 14, 697–703. doi: 10.1038/nn.2816
Kadowaki, M., Nakamura, S., Machon, O., Krauss, S., Radice, G. L., and Takeichi, M. (2007). N-cadherin mediates cortical organization in the mouse brain. Dev. Biol. 304, 22–33. doi: 10.1016/j.ydbio.2006.12.014
Kawauchi, T. (2011). Regulation of cell adhesion and migration in cortical neurons: not only Rho but also Rab family small GTPases. Small GTPases 2, 36–40. doi: 10.4161/sgtp.2.1.15001
Kawauchi, T. (2015). Cellullar insights into cerebral cortical development: focusing on the locomotion mode of neuronal migration. Front. Cell. Neurosci. 9:394. doi: 10.3389/fncel.2015.00394
Kawauchi, T., Sekine, K., Shikanai, M., Chihama, K., Tomita, K., Kubo, K.-I., et al. (2010). Rab GTPases-dependent endocytic pathways regulate neuronal migration and maturation through N-cadherin trafficking. Neuron 67, 588–602. doi: 10.1016/j.neuron.2010.07.007
Klaus, J., Kanton, S., Kyrousi, C., Ayo-Martin, A. C., Di Giaimo, R., Riesenberg, S., et al. (2019). Altered neuronal migratory trajectories in human cerebral organoids derived from individuals with neuronal heterotopia. Nat. Med. 25, 561–568. doi: 10.1038/s41591-019-0371-0
Kon, E., Calvo-Jiménez, E., Cossard, A., Na, Y., Cooper, J. A., and Jossin, Y. (2019). N-cadherin-regulated FGFR ubiquitination and degradation control mammalian neocortical projection neuron migration. eLife 8:e47673. doi: 10.7554/eLife.47673
Kotini, M., Barriga, E. H., Leslie, J., Gentzel, M., Rauschenberger, V., Schambony, A., et al. (2018). Gap junction protein Connexin-43 is a direct transcriptional regulator of N-cadherin in vivo. Nat. Commun. 9:3846. doi: 10.1038/s41467-018-06368-x
Kwon, Y. T., Gupta, A., Zhou, Y., Nikolic, M., and Tsai, L. H. (2000). Regulation of N-cadherin-mediated adhesion by the p35-Cdk5 kinase. Curr. Biol. 10, 363–372. doi: 10.1016/s0960-9822(00)00411-5
La Fata, G., Gärtner, A., Domínguez-Iturza, N., Dresselaers, T., Dawitz, J., Poorthuis, R. B., et al. (2014). FMRP regulates multipolar to bipolar transition affecting neuronal migration and cortical circuitry. Nat. Neurosci. 17, 1693–1700. doi: 10.1038/nn.3870
Lai, M., Guo, Y., Ma, J., Yu, H., Zhao, D., Fan, W., et al. (2015). Myosin X regulates neuronal radial migration through interacting with N-cadherin. Front. Cell. Neurosci. 9:326. doi: 10.3389/fncel.2015.00326
László, Z. I., Bercsenyi, K., Mayer, M., Lefkovics, K., Szabó, G., Katona, I., et al. (2020). N-cadherin (Cdh2) maintains migration and postmitotic survival of cortical interneuron precursors in a cell-type-specific manner. Cereb. Cortex 30, 1318–1329. doi: 10.1093/cercor/bhz168
Lee, D.-K., Lee, H., Yoon, J., Hong, S., Lee, Y., Kim, K.-T., et al. (2019). Cdk5 regulates N-cadherin-dependent neuronal migration during cortical development. Biochem. Biophys. Res. Commun. 514, 645–652. doi: 10.1016/j.bbrc.2019.04.166
Luccardini, C., Hennekinne, L., Viou, L., Yanagida, M., Murakami, F., Kessaris, N., et al. (2013). N-cadherin sustains motility and polarity of future cortical interneurons during tangential migration. J. Neurosci. 33, 18149–18160. doi: 10.1523/JNEUROSCI.0593-13.2013
Luccardini, C., Leclech, C., Viou, L., Rio, J.-P., and Métin, C. (2015). Cortical interneurons migrating on a pure substrate of N-cadherin exhibit fast synchronous centrosomal and nuclear movements and reduced ciliogenesis. Front. Cell. Neurosci. 9:286. doi: 10.3389/fncel.2015.00286
Marín, O., and Rubenstein, J. L. (2001). A long, remarkable journey: tangential migration in the telencephalon. Nat. Rev. Neurosci. 2, 780–790. doi: 10.1038/35097509
Martinez-Garay, I., Gil-Sanz, C., Franco, S. J., Espinosa, A., Molnár, Z., and Mueller, U. (2016). Cadherin2/4-signaling via PTP1B and catenins is critical for nucleokinesis during radial neuronal migration in the neocortex. Development 143, 2121–2134. doi: 10.1242/dev.132456
Mary, S., Charrasse, S., Meriane, M., Comunale, F., Travo, P., Blangy, A., et al. (2002). Biogenesis of N-cadherin-dependent cell-cell contacts in living fibroblasts is a microtubule-dependent kinesin-driven mechanism. Mol. Biol. Cell 13, 285–301. doi: 10.1091/mbc.01-07-0337
Matsunaga, Y., Noda, M., Murakawa, H., Hayashi, K., Nagasaka, A., Inoue, S., et al. (2017). Reelin transiently promotes N-cadherin-dependent neuronal adhesion during mouse cortical development. Proc. Natl. Acad. Sci. U.S.A. 114, 2048–2053. doi: 10.1073/pnas.1615215114
Nadarajah, B., Brunstrom, J. E., Grutzendler, J., Wong, R. O., and Pearlman, A. L. (2001). Two modes of radial migration in early development of the cerebral cortex. Nat. Neurosci. 4, 143–150. doi: 10.1038/83967
Noctor, S. C., Martínez-Cerdeño, V., Ivic, L., and Kriegstein, A. R. (2004). Cortical neurons arise in symmetric and asymmetric division zones and migrate through specific phases. Nat. Neurosci. 7, 136–144. doi: 10.1038/nn1172
Oishi, K., Nakagawa, N., Tachikawa, K., Sasaki, S., Aramaki, M., Hirano, S., et al. (2016). Identity of neocortical layer 4 neurons is specified through correct positioning into the cortex. eLife 5:e10907. doi: 10.7554/eLife.10907
Rakic, P. (1972). Mode of cell migration to the superficial layers of fetal monkey neocortex. J. Comp. Neurol. 145, 61–83. doi: 10.1002/cne.901450105
Rousso, D. L., Pearson, C. A., Gaber, Z. B., Miquelajauregui, A., Li, S., Portera-Cailliau, C., et al. (2012). Foxp-mediated suppression of N-cadherin regulates neuroepithelial character and progenitor maintenance in the CNS. Neuron 74, 314–330. doi: 10.1016/j.neuron.2012.02.024
Shah, B., Lutter, D., Tsytsyura, Y., Glyvuk, N., Sakakibara, A., Klingauf, J., et al. (2016). Rap1 GTPases are master regulators of neural cell polarity in the developing neocortex. Cereb. Cortex 27, 1253–1269. doi: 10.1093/cercor/bhv341
Sharma, M., and Henderson, B. R. (2007). IQ-domain GTPase-activating protein 1 regulates beta-catenin at membrane ruffles and its role in macropinocytosis of N-cadherin and adenomatous polyposis coli. J. Biol. Chem. 282, 8545–8556. doi: 10.1074/jbc.M610272200
Shashikanth, N., Petrova, Y. I., Park, S., Chekan, J., Maiden, S., Spano, M., et al. (2015). Allosteric regulation of E-cadherin adhesion. J. Biol. Chem. 290, 21749–21761. doi: 10.1074/jbc.M115.657098
Shikanai, M., Nakajima, K., and Kawauchi, T. (2011). N-cadherin regulates radial glial fiber-dependent migration of cortical locomoting neurons. Commun. Integr. Biol. 4, 326–330. doi: 10.4161/cib.4.3.14886
Shikanai, M., Nishimura, Y. V., Sakurai, M., Nabeshima, Y.-I., Yuzaki, M., and Kawauchi, T. (2018). Caveolin-1 promotes early neuronal maturation via caveolae-independent trafficking of N-cadherin and L1. iScience 7, 53–67. doi: 10.1016/j.isci.2018.08.014
Solecki, D. J. (2012). Sticky situations: recent advances in control of cell adhesion during neuronal migration. Curr. Opin. Neurobiol. 22, 791–798. doi: 10.1016/j.conb.2012.04.010
Sotomayor, M., Gaudet, R., and Corey, D. P. (2014). Sorting out a promiscuous superfamily: towards cadherin connectomics. Trends Cell Biol. 24, 524–536. doi: 10.1016/j.tcb.2014.03.007
Tabata, H., and Nakajima, K. (2003). Multipolar migration: the third mode of radial neuronal migration in the developing cerebral cortex. J. Neurosci. 23, 9996–10001. doi: 10.1523/jneurosci.23-31-09996.2003
Tai, C.-Y., Mysore, S. P., Chiu, C., and Schuman, E. M. (2007). Activity-regulated N-cadherin endocytosis. Neuron 54, 771–785. doi: 10.1016/j.neuron.2007.05.013
Teng, J., Rai, T., Tanaka, Y., Takei, Y., Nakata, T., Hirasawa, M., et al. (2005). The KIF3 motor transports N-cadherin and organizes the developing neuroepithelium. Nat. Cell Biol. 7, 474–482. doi: 10.1038/ncb1249
Wahl, J. K., Kim, Y. J., Cullen, J. M., Johnson, K. R., and Wheelock, M. J. (2003). N-cadherin-catenin complexes form prior to cleavage of the proregion and transport to the plasma membrane. J. Biol. Chem. 278, 17269–17276. doi: 10.1074/jbc.M211452200
Wandinger-Ness, A., and Zerial, M. (2014). Rab proteins and the compartmentalization of the endosomal system. Cold Spring Harb. Perspect. Biol. 6:a022616. doi: 10.1101/cshperspect.a022616
Wehrendt, D. P., Carmona, F., Wusener, A. E. G., González, Á., Martínez, J. M. L., and Arregui, C. O. (2016). P120-catenin regulates early trafficking stages of the N-cadherin precursor complex. PLoS One 11:e0156758. doi: 10.1371/journal.pone.0156758
West, J. J., and Harris, T. J. C. (2016). Cadherin trafficking for tissue morphogenesis: control and consequences. Traffic 17, 1233–1243. doi: 10.1111/tra.12407
Wu, Q., Sun, X., Yue, W., Lu, T., Ruan, Y., Chen, T., et al. (2016). RAB18, a protein associated with Warburg Micro syndrome, controls neuronal migration in the developing cerebral cortex. Mol. Brain 9:19.
Xu, C., Funahashi, Y., Watanabe, T., Takano, T., Nakamuta, S., Namba, T., et al. (2015). Radial glial cell-neuron interaction directs axon formation at the opposite side of the neuron from the contact site. J. Neurosci. 35, 14517–14532. doi: 10.1523/JNEUROSCI.1266-15.2015
Ye, T., Ip, J. P. K., Fu, A. K. Y., and Ip, N. Y. (2014). Cdk5-mediated phosphorylation of RapGEF2 controls neuronal migration in the developing cerebral cortex. Nat Commun. 5:4826. doi: 10.1038/ncomms5826
Keywords: cerebral cortex, neuron, migration, cell surface, adhesion molecules, CDH2, molecular mechanism
Citation: Martinez-Garay I (2020) Molecular Mechanisms of Cadherin Function During Cortical Migration. Front. Cell Dev. Biol. 8:588152. doi: 10.3389/fcell.2020.588152
Received: 28 July 2020; Accepted: 27 August 2020;
Published: 15 September 2020.
Edited by:
Yuki Hirota, Keio University, JapanReviewed by:
Takeshi Kawauchi, Foundation for Biomedical Research and Innovation at Kobe, JapanCopyright © 2020 Martinez-Garay. This is an open-access article distributed under the terms of the Creative Commons Attribution License (CC BY). The use, distribution or reproduction in other forums is permitted, provided the original author(s) and the copyright owner(s) are credited and that the original publication in this journal is cited, in accordance with accepted academic practice. No use, distribution or reproduction is permitted which does not comply with these terms.
*Correspondence: Isabel Martinez-Garay, bWFydGluZXpnYXJheWlAY2FyZGlmZi5hYy51aw==
Disclaimer: All claims expressed in this article are solely those of the authors and do not necessarily represent those of their affiliated organizations, or those of the publisher, the editors and the reviewers. Any product that may be evaluated in this article or claim that may be made by its manufacturer is not guaranteed or endorsed by the publisher.
Research integrity at Frontiers
Learn more about the work of our research integrity team to safeguard the quality of each article we publish.