- 1Department of Plastic Surgery, The First Affiliated Hospital of China Medical University, Shenyang, China
- 2Department of Pharmaceutical Science, The First Affiliated Hospital of China Medical University, Shenyang, China
- 3Cancer Therapy Research Institute, The First Affiliated Hospital of China Medical University, Shenyang, China
As a classical immune checkpoint molecule, PD-L1 on the surface of tumor cells plays a pivotal role in tumor immunosuppression, primarily by inhibiting the antitumor activities of T cells by binding to its receptor PD-1. PD-1/PD-L1 inhibitors have demonstrated unprecedented promise in treating various human cancers with impressive efficacy. However, a significant portion of cancer patients remains less responsive. Therefore, a better understanding of PD-L1-mediated immune escape is imperative. PD-L1 can be expressed on the surface of tumor cells, but it is also found to exist in extracellular forms, such as on exosomes. Recent studies have revealed the importance of exosomal PD-L1 (ExoPD-L1). As an alternative to membrane-bound PD-L1, ExoPD-L1 produced by tumor cells also plays an important regulatory role in the antitumor immune response. We review the recent remarkable findings on the biological functions of ExoPD-L1, including the inhibition of lymphocyte activities, migration to PD-L1-negative tumor cells and immune cells, induction of both local and systemic immunosuppression, and promotion of tumor growth. We also discuss the potential implications of ExoPD-L1 as a predictor for disease progression and treatment response, sensitive methods for detection of circulating ExoPD-L1, and the novel therapeutic strategies combining the inhibition of exosome biogenesis with PD-L1 blockade in the clinic.
Introduction
Programmed cell death protein-ligand 1 (PD-L1) is an immune checkpoint molecule that interacts with programmed cell death protein-1 (PD-1) to mediate immunosuppression (Ribas and Hu-Lieskovan, 2016; Alsaab et al., 2017; Sun et al., 2018; Han et al., 2020). Binding of PD-L1 to PD-1 conveys a regulatory signal to T cells and an antiapoptotic signal to tumor cells, resulting in T cells exhaustion and tumor cell survival (Dong et al., 2002; Jiang X. et al., 2019; Jiang Y. et al., 2019). It is known that, as a membrane-bound molecule, PD-L1 is expressed on the cell surface of many tumor types and the PD-1/PD-L1 pathway is considered to be a critical mechanism for immune escape and tumor progression (Quail and Joyce, 2013; Yu et al., 2016; Kakavand et al., 2017; Kythreotou et al., 2018; Cha et al., 2019). Therefore, anti-PD-1/PD-L1 inhibitors are able to induce durable tumor regression and represent a unique therapeutic strategy for patients with advanced cancers (Couzin-Frankel, 2013; Mahoney et al., 2015; Ribas et al., 2016; Yaghoubi et al., 2019). However, the effective rate of anti-PD-1/PD-L1 immunotherapy remains low (Xu-Monette et al., 2017; Chamoto et al., 2020; Hatae et al., 2020). Furthermore, patients exhibiting negative PD-L1 expression can also benefit from anti-PD-1/PD-L1 blockade (Patel and Kurzrock, 2015; Shukuya and Carbone, 2016; Shen and Zhao, 2018). Thus, neither the expression pattern of PD-L1 on the tumor cell surface alone is sufficient to account for the mechanism of tumor immune escape nor accurate for predicting the response to anti-PD-1/PD-L1 treatment, although tumor tissue PD-L1 is the only indicator authorized by the FDA (Festino et al., 2016; Wang Q. et al., 2017; Li et al., 2019c; Martinez-Morilla et al., 2020). Therefore, as an alternative to membrane-bound PD-L1, exosomal PD-L1 (ExoPD-L1) that is associated with exosomes secreted by tumor cells has been investigated recently.
Extracellular vesicles (EVs) are membrane-enveloped particles produced by almost all cell types and are classified into three subgroups: microvesicles, apoptotic bodies, and exosomes, according to their biogenesis, cellular source and biological properties (Gould and Raposo, 2013; Colombo et al., 2014; Yanez-Mo et al., 2015; Xu et al., 2016; Hessvik and Llorente, 2018; van Niel et al., 2018; Margolis and Sadovsky, 2019). Microvesicles and apoptotic bodies are large EVs (normally 100−1000 μm) and are shed directly from the plasma membrane (Gould and Raposo, 2013; van Niel et al., 2018; Margolis and Sadovsky, 2019). Exosomes are small EVs (typically 30−100 μm) generated by the endocytic pathway (Thery et al., 2006; Kowal et al., 2014; Lobb et al., 2015; Kalluri, 2016; Tkach and Thery, 2016; Tkach et al., 2018). After the fusion of endosomal multivesicular bodies (MVBs) with the plasma membrane, exosomes are secreted extracellularly (Carlton, 2010; Stoorvogel, 2015; Ha et al., 2016). Neutral sphingomyelinase type 2 (nSMase2) and Rab27a are two key enzymes in the biogenesis of exosomes (Hessvik and Llorente, 2018). They are involved in the inward budding of MVBs to form intraluminal vesicles (ILVs), the intracellular precursors of exosomes, and the transportation and fusion of the MVBs to the plasma membrane, respectively (Trajkovic et al., 2008; Ostrowski et al., 2010; Lallemand et al., 2018). Genetic and pharmacological manipulation of these enzymes offers an approach to determine the various roles of exosomes in vitro and in vivo. Increasing evidence indicates that exosomes derived from tumor cells can regulate the tumor microenvironment and promote cancer progression via their cargos, which include proteins, lipids, and nucleic acids (Anastasiadou and Slack, 2014; Lazaro-Ibanez et al., 2019; Zhang and Yu, 2019; Raimondo et al., 2020; Sahebi et al., 2020). Recent studies have demonstrated that PD-L1 also exists on the surface of exosomes generated by their parental tumor cells (Chen G. et al., 2018; Lubin et al., 2018; Ricklefs et al., 2018; Theodoraki et al., 2018b; Yang et al., 2018; Fan et al., 2019; Kim et al., 2019; Poggio et al., 2019; Cordonnier et al., 2020; Huang et al., 2020). Moreover, ExoPD-L1 can function as efficiently as PD-L1 on the tumor cell surface through direct ligation to PD-1 on the surface of lymphocytes in tumor foci (Chen G. et al., 2018; Lubin et al., 2018; Ricklefs et al., 2018; Theodoraki et al., 2018b; Yang et al., 2018; Fan et al., 2019; Kim et al., 2019; Poggio et al., 2019; Cordonnier et al., 2020; Huang et al., 2020). Surprisingly, although the cell-surface PD-L1 is low or absent, the ExoPD-L1 may be highly secreted by its parental tumor cells that are resistant to anti-PD-L1 therapy (Poggio et al., 2019). Overall, ExoPD-L1 plays a pivotal role in immunosuppression and tumor progression.
In this review, we summarize the various functions of ExoPD-L1 secreted by tumor cells, focusing on the recent findings regarding their expression heterogeneity, the impact on local and systemic immune response, and tumor growth. Moreover, we also discuss the clinical implications of circulating ExoPD-L1 as a non-invasive biomarker to predict tumor progression and immunotherapeutic response, and as a novel target to develop more effective antitumor strategies.
The Expression Pattern of Tumor ExoPD-L1
It is well known that the PD-L1 protein is abundantly expressed on the cell surface of various cancers (Cimino-Mathews et al., 2016; Nduom et al., 2016; Brody et al., 2017; Sunshine et al., 2017). Recent studies have shown that tumor cells can secrete PD-L1 in EVs, particularly in exosomes, which are generally present in the pellet obtained by ultracentrifugation (Chen G. et al., 2018; Yang et al., 2018; Kim et al., 2019; Poggio et al., 2019). Colocalization of PD-L1 and exosomal marker CD63 in MVBs is observed in human breast cancer tissues by immunohistochemical staining (Pols and Klumperman, 2009; Khushman et al., 2017; Farooqi et al., 2018; Yang et al., 2018). Furthermore, human ExoPD-L1 was found in the circulation of nude mice bearing human melanoma xenografts (Chen G. et al., 2018). Thereby, both human and murine tumor cells can secrete ExoPD-L1 both in vitro and in vivo.
The expression of ExoPD-L1 is highly heterogeneous in tumor cells. The variability in the levels of ExoPD-L1 is quite significant between different tumor types and even between different cell lines of the same type (Table 1). In addition, it appears that ExoPD-L1 levels are consistent with the levels of PD-L1 expressed in their parental tumor cells (Chen G. et al., 2018; Ricklefs et al., 2018; Fan et al., 2019; Kim et al., 2019). However, an exception is observed in prostate cancer. These tumor cells produce high levels of PD-L1-containing exosomes, but are devoid of PD-L1 on the tumor cell surface, despite expressing constitutively high levels of PD-L1 mRNA (Poggio et al., 2019). Considering the discordance between exosomal and cell-surface PD-L1 expression, the expression pattern of ExoPD-L1 from tumor cells, especially that of low or undetectable cell-surface PD-L1, should not be neglected. In addition, interferon-γ, a typical inflammatory cytokine, upregulates ExoPD-L1 production by melanoma, breast cancer, prostate cancer, glioblastoma, and non-small cell lung carcinoma (NSCLC) (Chen G. et al., 2018; Monypenny et al., 2018; Ricklefs et al., 2018; Poggio et al., 2019). However, the mechanism regulating ExoPD-1 release is not fully understood. Thus, endeavors to further explore the molecular mechanisms regulating ExoPD-L1 expression are warranted.
The Immunosuppressive Effects of ExoPD-L1
The modulatory effect of tumor cell PD-L1 occurs through binding to PD-1. PD-L1 is a typical transmembrane protein (Dong et al., 1999, 2002). Recent studies reveal that ExoPD-L1 displays the same extracellular domain topology as its cell-surface counterpart (Chen G. et al., 2018). Therefore, ExoPD-L1 may exert a similar function as tumor cell-surface PD-L1 by engaging with PD-1.
The Interaction Between ExoPD-L1 and PD-1 on T Cells
ExoPD-L1 Directly Binds to PD-1 on T Cells
In vitro binding assays showed that PD-L1 on melanoma-derived exosomes is able to ligate to soluble PD-1 molecules in a concentration-dependent manner (Chen G. et al., 2018; Ricklefs et al., 2018; Yang et al., 2018). Consistently, both PD-L1 and PD-1 blocking antibodies can disrupt the ligation in a dose-dependent manner (Chen G. et al., 2018; Ricklefs et al., 2018). The physical combination of melanoma exosomes and T cells was confirmed by using confocal microscopy, flow cytometry and enzyme linked immunosorbent assay (ELISA) (Chen G. et al., 2018; Ricklefs et al., 2018). The binding of melanoma-derived exosomes to CD8+ T cells is increased when the levels of either PD-1 on CD8+ T cells or ExoPD-L1 are upregulated (Chen G. et al., 2018). Studies on glioblastoma-derived exosomes also show that ExoPD-L1 binds to CD4+ and CD8+ T cells (Ricklefs et al., 2018). Furthermore, the in vivo colocalization of ExoPD-L1 to tumor-infiltrating lymphocytes (TILs) in mouse glioblastoma tissues was visualized (Ricklefs et al., 2018). Thus, ExoPD-L1 can ligate to PD-1 on T cells, which is an alternative pathway to membrane-bound PD-L1 interacting with its receptor PD-1.
ExoPD-L1 Interacts With PD-1 on T Cells After Migration to PD-L1-Negative Tumor Cells
It has been demonstrated that exosomes can transfer specific proteins, nucleic acids, and lipids from donor cells to recipient cells, thereby influencing the phenotype of the recipient cells (Milane et al., 2015; Ruivo et al., 2017; Wan et al., 2018; Lazaro-Ibanez et al., 2019). Recent studies found that tumor-derived exosomes can transport PD-L1 from PD-L1-positive tumor cells to PD-L1-negative tumor cells (Yang et al., 2018). After a 24 h incubation with ExoPD-L1 derived from breast cancer cells with constitutive PD-L1 expression, high levels of PD-L1 were detected in breast cancer cells with PD-L1 knockdown or low PD-L1 expression (Yang et al., 2018). Notably, the ExoPD-L1 migration to PD-L1-negative tumor cells was detectable in tumor masses of mice 5 days after coinjection of ExoPD-L1 (Yang et al., 2018). Furthermore, ExoPD-L1 can be transported to immune cells, including human macrophages and dendritic cells in vitro and murine tumor-infiltrated macrophages in vivo (Yang et al., 2018). More importantly, results obtained from flow cytometric analysis demonstrated that the ExoPD-L1, which settled on the surface of the PD-L1-negative tumor cells, is capable of binding to the PD-1 Fc fragment (Yang et al., 2018). Thus, the ExoPD-L1 that migrates to the surface of recipient cells from PD-L1-positive tumor cells still maintains its ability to bind to PD-1 on T cells (Yang et al., 2018).
Notably, CD80 is also a binding partner of PD-L1 and competes with PD-1 for engaging PD-L1 (Butte et al., 2008; Park et al., 2010; Chen and Flies, 2013). The interaction of PD-L1 on tumor cells and CD80 on T cells suppresses T cell activation and survival, suggesting that dual blocking PD-1 and CD80 interaction with PD-L1 might be more favorable for improving the immunotherapy efficacy compared with single PD-1 blockade (Butte et al., 2007; Rollins and Gibbons Johnson, 2017). In addition, PD-L1 can interact in cis with CD80 on the same cell (Chaudhri et al., 2018). The cis-heterodimer of PD-L1 and CD80 on antigen presenting cells is able to restrict PD-1 function and is the requirement for triggering T cell responses (Sugiura et al., 2019; Zhao Y. et al., 2019; Mayoux et al., 2020). Therefore, it is necessary to dissect the functions contributed by the crosstalk between PD-L1/PD-1 and PD-L1/CD80 pathways in tumor microenvironment to explore new opportunities for tumor treatment.
The PD-1/PD-L1 signaling pathway in activated T cells has been reviewed in recent literatures (Ai et al., 2020; Bastaki et al., 2020; Wu et al., 2020). After PD-1 binds to PD-L1, the cytoplasmic tail of PD-1 is phosphorylated and recruits Src homology phosphatase 1 (SHP-1) and SHP-2 (Chemnitz et al., 2004; Yokosuka et al., 2012; Chinai et al., 2015). SHP-2 dephosphorylates and inhibits T cell receptor (TCR) and downstream signaling, such as zeta-chain-associated protein kinase 70 (ZAP70), phosphoinositide 3-kinase (PI3K), protein kinase B (PKB/AKT), mammalian target of rapamycin (mTOR), rat sarcoma (RAS), mitogen-activated protein kinase (MAPK/MEK), and extracellular regulated protein kinase (ERK) (Sheppard et al., 2004; Parry et al., 2005; Riley, 2009; Patsoukis et al., 2012; Hui et al., 2017). Additionally, recent studies reported that CD28, rather than the TCR, is the primary target of SHP-2 (Hui et al., 2017; Kamphorst et al., 2017), suggesting that PD-1 may target both TCR and CD28 to exert regulatory function. It has been shown that ExoPD-L1 released by breast cancer cells significantly suppresses ERK phosphorylation and nuclear factor kappa-B activation in CD3/CD28-activated T cells (Yang et al., 2018). However, whether other molecules participate in the ExoPD-L1 signaling, especially in the context of tumorigenesis, remains unclear and needs to be further investigated.
The in vitro Immunosuppressive Effects of ExoPD-L1
It has been reported that tumor-derived exosomes contribute to CD8+ T cell dysfunction, although the mechanism is not fully understood (Ludwig et al., 2017; Maybruck et al., 2017; Huang et al., 2018; Wang T. et al., 2019). Recent studies found that ExoPD-L1 secreted by tumor cells can efficiently induce T cell dysfunction via interacting with its surface PD-1 (Table 2).
Protein-1 is mainly expressed on activated T cells and it is a central inhibitory receptor that regulates CD8+ T cell dysfunction in tumors (Ahn et al., 2018; Miller et al., 2019). Recent studies found that the activation-signaling pathway in T cells is inhibited in a dose-dependent manner from exposure to ExoPD-L1 derived from breast cancer cells (Yang et al., 2018). Furthermore, the production of IFN-γ, IL-2, and TNF-α by CD8+ T cells was decreased in the presence of ExoPD-L1 derived from melanoma, breast cancer, NSCLC, and prostate cancer (Chen G. et al., 2018; Yang et al., 2018; Kim et al., 2019; Poggio et al., 2019; Cordonnier et al., 2020). In addition, ExoPD-L1 shuts down the expression of activation markers on CD4+ and CD8+ T cells, including CD69, CD25, and PD-1 (Ricklefs et al., 2018; Fan et al., 2019; Cordonnier et al., 2020). Pretreatment with anti-PD-1 antibodies or PD-L1 knockdown constructs significantly diminished the suppression of T cell activation mediated by ExoPD-L1 (Chen G. et al., 2018; Ricklefs et al., 2018; Theodoraki et al., 2018b; Yang et al., 2018; Fan et al., 2019; Kim et al., 2019). Importantly, exosomes from plasma of patients with NSCLC and headneck squamous cell carcinoma (HNSCC) display modulatory effects on T cells (Theodoraki et al., 2018a; Kim et al., 2019). Remarkably, ExoPD-L1 from tumors is not only as efficient as cellular PD-L1, but also stronger than soluble PD-L1 in suppressing T cell activation because of the high stability of ExoPD-L1 and MHC-I expression (Fan et al., 2019; Cordonnier et al., 2020).
Recent studies showed that exosomes derived from human melanoma and NSCLC significantly reduced the Ki-67 expression of T cells and CD8+ T cells, which is restored in the presence anti-PD-1 blocking antibodies (Chen G. et al., 2018; Cordonnier et al., 2020). Additionally, exosomes from human glioblastoma culture block both CD8+ and CD4+ T cell proliferation (Ricklefs et al., 2018). Notably, ExoPD-L1 from NSCLC and HNSCC cells induced apoptosis in CD8+ T cells and the amount of CD8+ T cells decreased in a dose-dependent manner (Theodoraki et al., 2018a; Kim et al., 2019). Overall, tumor-derived ExoPD-L1 is able to suppress the proliferation and survival of T cells, which contributes to T cell dysfunction (Li et al., 2019b; Xia et al., 2019).
The cytotoxicity of functional effector T cells is responsible for killing cancer cells and eradicating tumors. Exosomes secreted from melanoma and NSCLC cells that express endogenous PD-L1 inhibit the expression of granzyme B (GzmB) from human peripheral and mouse splenic CD8+ T cells activated by TCR stimulation (Chen G. et al., 2018). Pretreatment with exosomes from tumors, such as melanoma and breast cancer, significantly inhibited the cytotoxic T cell-mediated tumor killing, which could be counteracted by anti-PD-L1 antibodies (Chen G. et al., 2018; Yang et al., 2018; Kim et al., 2019). Thus, tumor-derived ExoPD-L1 is capable of inhibiting T cell function by modulating the proliferative capacity and effector function of T cells (Table 2).
Together, ExoPD-L1 secreted from tumor cells is able to mediate immunosuppression in vitro. The expression of inhibitory receptors is also a characteristic of T cell dysfunction, except for low proliferation and loss of effector function (Xia et al., 2019). Therefore, in addition to PD-1, the impact of ExoPD-L1 on other T cell inhibitory receptors should be investigated, including T cell immunoglobulin domain and mucin domain-3 (TIM-3), cytotoxic T lymphocyte antigen 4 (CTLA-4), lymphocyte activation gene 3 (LAG-3), T cell immunoreceptor with Ig and ITIM domains (TIGIT) (Anderson et al., 2016; Andrews et al., 2019; Wolf et al., 2019).
The Immunoinhibitory Effects of ExoPD-L1 in Mouse Tumor Models
Insight into the immunosuppressive effects of ExoPD-L1 in vivo is beneficial to understanding the mechanisms of tumor immune escape. Recent studies have shown that ExoPD-L1 released by tumor cells induces immunosuppressive activities at tumor sites in a paracrine-dependent manner (Chen G. et al., 2018; Yang et al., 2018; Kim et al., 2019). Additionally, exosomes can enter blood and circulate systemically, which may help ExoPD-L1 to function at distant sites in a manner similar to endocrine molecules (Figure 1; Seo et al., 2018; Wortzel et al., 2019).
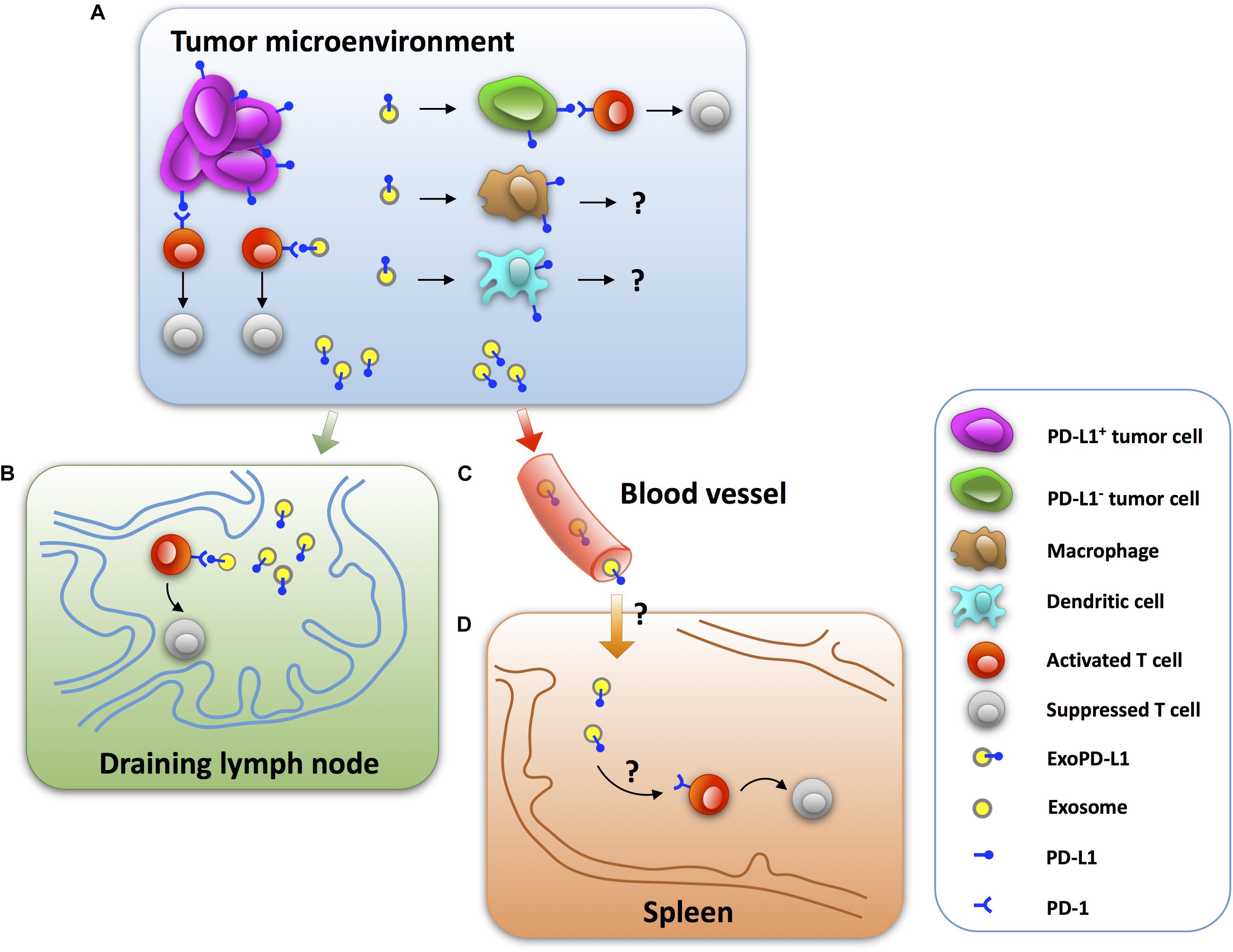
Figure 1. The mechanisms by which tumor ExoPD-L1 induce immunosuppression. (A) ExoPD-L1 originating from tumor cells induces T cell dysfunction in the tumor microenvironment by directly ligating to PD-1 on T cells as well as stationing on PD-L1-negative tumor cells, while its immunoregulatory effect remains intact. ExoPD-L1 also migrates to macrophages and dendritic cells, but the potential effects remain unknown. (B) ExoPD-L1 is able to leave tumor foci and enter the draining lymph node to mediate T cell suppression. (C) ExoPD-L1 can enter and circulate in the blood. (D) ExoPD-L1 inhibits the immune response in spleen and decreases spleen size.
Decreased Frequencies and Activities of TILs
A significant reduction in the number of CD8+ TILs was observed in melanoma tumors of C57BL/6 mice after 24 days of an injection with PD-L1-containing exosomes (Chen G. et al., 2018). Moreover, the frequency of Ki67+PD-1+CD8+ T cells in the tumor microenvironment decreased significantly, which was reversed by anti-PD-L1 antibodies (Chen G. et al., 2018). There was also a significant loss of CD8+ TILs in the tumor area of NSCLC after an intravenous injection of PD-L1-containing exosomes in mice after 14 days (Kim et al., 2019). Furthermore, PD-L1-containing exosomes reduce cytotoxic T cell activity, as assessed by GzmB expression, in the tumor microenvironment (Yang et al., 2018). Collectively, these results suggest that ExoPD-L1 plays a key role in induction of immune escape in tumor microenvironment (Figure 1).
Suppression of T Cell Function in the Draining Lymph Node
It is clear that exosomes are important communicators between tumors and immune cells and they exert modulatory effects on the systemic immune response (Hood et al., 2011; Groot Kormelink et al., 2018; Czystowska-Kuzmicz et al., 2019; Sheehan and D’Souza-Schorey, 2019). Recent studies reported that PD-L1-deletion significantly increases the number, proliferation (Ki67), and effector function (GzmB) of CD8+ T cells, while decreasing the exhaustion (TIM-3) of CD8+ T cells in the draining lymph node of mice injected with TRAMP-C2 prostate cancer cells (Poggio et al., 2019). Meanwhile, similar to PD-L1-deletion, Rab27a-deletion, which inhibits the biogenesis of exosomes, has a promotional effect on the frequency and activity of CD8+ T cells (Poggio et al., 2019). More importantly, the administration of exogenous exosomes derived from wild-type (Newton et al., 2016) prostate cancer cells leads to immunosuppression in the draining lymph node, as evidenced by a reduced number and effector function, and increased exhaustion of CD8+ T cells of mice injected with Rab27a-deleted prostate cancer cells (Poggio et al., 2019). Moreover, the administration of WT melanoma cell-derived exosomes significantly reduced the proportion of Ki67+PD-1+CD8+ T cells in the draining lymph node of mice injected with the PD-L1-deleted melanoma cells, which was counteracted by anti-PD-L1 antibodies (Chen G. et al., 2018). Thus, these findings indicate that tumor-derived ExoPD-L1 is capable of patrolling the draining lymph node and regulating T cell activation (Figure 1).
Reduction of Spleen Size and T Cell Proliferation in Spleen
In addition to inducing immune suppression in the draining lymph node, ExoPD-L1 can inhibit the immune response in the spleen. Recent studies reported that PD-L1-deletion significantly increases the spleen size of mice injected with TRAMP-C2 prostate cancer cells (Poggio et al., 2019). Meanwhile, Rab27a-deletion also increases the spleen size of mice injected with prostate cancer cells. Notably, intravenous injection of exogenous exosomes derived from WT prostate cancer cells resulted in decreased spleen size of mice injected with Rab27a-deleted prostate cancer cells to nearly 50% (Poggio et al., 2019). In addition, administration of WT melanoma B16-F10 cell-derived exosomes significantly reduced the proportion of Ki67+PD-1+CD8+ T cells in murine spleen injected with the PD-L1-deleted melanoma cells. This effect could be counteracted by anti-PD-L1 antibody treatment (Chen G. et al., 2018). Thus, ExoPD-L1 secreted by tumor cells can enter into the circulation to inhibit the antitumor immunity systemically (Figure 1).
Promotion of Tumor Growth Across Different Tumor Types in an Immune-Dependent Manner
It has been shown that ExoPD-L1 derived from tumor cells promotes tumor growth in vivo, including cancers of the breast and prostate, colorectal cancer, melanoma, and NSCLC (Chen G. et al., 2018; Yang et al., 2018; Kim et al., 2019; Poggio et al., 2019). PD-L1 knockout leads to substantial tumor regression or even failure to grow, however, this was reversed by local or intravenous injection of exosomes derived from WT tumor cells (Chen G. et al., 2018; Yang et al., 2018; Poggio et al., 2019). Different from PD-L1 deletion, which downregulates the transcripts of PD-L1 mRNA, genetic deletion of Rab27a or nSMase2 reduces the production of ExoPD-L1 via inhibition of exosome biogenesis. In vitro studies showed that blockade of Rab27a or nSMase2 does not change cell proliferation in prostate cancer, colorectal cancer, or breast cancer, indicating that blockade of exosome biogenesis itself does not cause the suppression of tumor growth (Yang et al., 2018; Poggio et al., 2019). However, deletion of either Rab27a or nSMase2 significantly inhibits tumor growth in mice, including that of breast, prostate, and colorectal cancers (Yang et al., 2018; Poggio et al., 2019). These findings reveal that the inhibition of exosome biogenesis or PD-L1 deletion results in a similar suppressive effect on tumor growth. In addition, prostate and breast cancer cells experiencing a blockade of Rab27a, nSMase2 or PD-L1 failed to grow in WT mice, but grow rapidly in immunodeficient mice (Yang et al., 2018; Poggio et al., 2019). Collectively, ExoPD-L1 promotes tumor growth that is dependent on the inhibition of the antitumor immune response.
ExoPD-L1 Contributes to the Immune Suppression in Tumor Patients
It has been observed that circulating ExoPD-L1 level is positively associated with its ability to suppress the activation of CD8+ T cells in HNSCC patients (Theodoraki et al., 2018b). Additionally, in metastatic gastric tumor patients, the levels of plasma ExoPD-L1 are negatively associated with CD4+ and CD8+ T cell counts as well as the cytotoxicity of T cells (Fan et al., 2019). These findings indicate that ExoPD-L1 contributes to immunosuppression by inducing T-cell dysfunction, suggesting that ExoPD-L1 might promote the disease progression of tumor patients (Theodoraki et al., 2018b; Fan et al., 2019).
Collectively, tumor ExoPD-L1 plays a pivotal part in mediating local and systemic immunosuppression in mouse models and tumor patients.
Potential Clinical Implication of Circulating ExoPD-L1 as a Biomarker for Tumor Diagnosis, Disease Progression, and Immunotherapy Response
Tumor immunotherapy requires biomarkers for predicting disease progression, prognosis, clinical response, and the selection of suitable patients (Buder-Bakhaya and Hassel, 2018; Zhang et al., 2019). Tumor cell PD-L1 has been considered a predictor for response to immunotherapy in the clinic (Patel and Kurzrock, 2015; Aguiar et al., 2017; Lin et al., 2018; Li et al., 2019c). However, there are pitfalls of using cellular PD-L1 such as traumatic biopsy, missing small tumors, heterogeneity of PD-L1 expression within tumors, unavailability of dynamic observation, and limited sensitivity (Kaunitz et al., 2017; Bassanelli et al., 2018; Teixido et al., 2018; Davis and Patel, 2019; Stovgaard et al., 2019; Xu G. et al., 2019). Recent studies indicate that circulating ExoPD-L1 is emerging as a non-invasive and readily available biomarker, and is more easily detectable and reliable than both tissue and soluble PD-L1 in plasma (Liu et al., 2018b; Cordonnier et al., 2020; Huang et al., 2020; Pang et al., 2020).
ExoPD-L1 as a Biomarker for Diagnosis and Disease Progression
Tumor-derived exosomes can be enriched from small volumes of patient plasma and are considered to be a potential biomarker based on liquid biopsy (Crow et al., 2019; Johnsen et al., 2019; Xie C. et al., 2019; Yekula et al., 2019, 2020; Brennan et al., 2020). The number and the protein content of exosomes in the blood of breast cancer patients are higher compared with those of healthy subjects and the increased exosome numbers positively correlates with tumor growth (Hesari et al., 2018). However, in melanoma, the level of ExoPD-L1 in the blood, rather than the number and total protein content of exosomes, is elevated in metastatic melanoma patients compared with healthy subjects (Chen G. et al., 2018). Furthermore, patients with NSCLC and adenocarcinoma also exhibit higher levels of circulating ExoPD-L1 compared with healthy controls (Liu et al., 2018b; Li et al., 2019a; Huang et al., 2020; Pang et al., 2020). Therefore, circulating ExoPD-L1 may be a potential diagnostic marker. In addition, high levels of circulating ExoPD-L1 were associated with metastatic melanoma, advanced HNSCC, and poor prognosis in pancreatic cancer, further indicating that circulating ExoPD-L1 may be a useful biomarker for tumor progression (Theodoraki et al., 2018a; Lux et al., 2019; Huang et al., 2020).
Soluble PD-L1 in plasma is also considered as a potential diagnostic and predictive biomarker for tumor recurrence and prognosis (Chatterjee et al., 2017; Okuma et al., 2017; Zhou et al., 2017; Chang et al., 2019; Shigemori et al., 2019; Liu S. et al., 2020). However, the levels of soluble PD-L1 were not different between NSCLC patients and healthy donors (Li et al., 2019a). Moreover, soluble PD-L1 did not correlate with disease progression in patients with metastatic gastric cancer, HNSCC, or NSCLC (Liu et al., 2018b; Fan et al., 2019; Li et al., 2019a; Pang et al., 2020). On the contrary, circulating ExoPD-L1 in plasma is an independent biomarker to predict poor prognosis in patients with metastatic gastric cancer (Fan et al., 2019). Furthermore, the levels of ExoPD-L1 correlated with the disease progression of patients with HNSCC and NSCLC, including tumor size, lymph node status, metastasis, and clinical stage (Theodoraki et al., 2018a, b; Li et al., 2019a).
Exosomal PD-L1 DNA is present in exosomes isolated from the plasma of glioblastoma patients (Ricklefs et al., 2018). The amount of ExoPD-L1 DNA from glioblastoma patients is associated with tumor volume, although the function of PD-L1 DNA remains unknown (Ricklefs et al., 2018; Lazaro-Ibanez et al., 2019). Together, liquid biopsy analysis of ExoPD-L1 protein and DNA in blood may provide biomarkers for tumor diagnosis and disease progression.
ExoPD-L1 as a Biomarker for Efficacy of Anti-PD-1/PD-L1 Therapy
It is important to provide individualized precise treatment and to predict tumor response to immunotherapy (Madore et al., 2015; Kaunitz et al., 2017; Sui et al., 2018; Liu and Wu, 2019). To fulfill the need for real-time monitoring, the analysis of liquid biopsy-based circulating biomarkers is preferred (Nishino et al., 2013; Ando et al., 2019; Gregg et al., 2019; Kloten et al., 2019; Tang et al., 2020). Recent studies demonstrated that melanoma patients who were less responsive to anti-PD-1 blockade had a significantly higher level of circulating ExoPD-L1 prior to treatment as compared with responders (Chen G. et al., 2018). In addition, the increasing magnitude of circulating ExoPD-L1 in melanoma patients during early treatment periods can distinguish clinical responders from non-responders (Chen G. et al., 2018). Moreover, a prospective study on melanoma indicated that monitoring the levels of circulating ExoPD-L1 may be helpful to predict therapeutic efficacy and clinical outcome (Cordonnier et al., 2020). Additionally, compared with patients exhibiting recurrence, patients who did not relapse had higher levels of tumor-enriched CD3- ExoPD-L1 prior to therapy, which significantly decreased after five weeks of therapy (Theodoraki et al., 2018a, 2019). In contrast, tumor-enriched ExoPD-L1 levels increased at week five of therapy, whereas the CD3+ ExoPD-L1 levels decreased in patients with recurrence (Theodoraki et al., 2019). Thus, studying on the role of ExoPD-L1 derived from immune cells and tumor cells as biomarkers for tumor patients will be necessary. Interestingly, ExoPD-L1 mRNA can also be sequestered in exosomes of patient plasma in melanoma and NSCLC, and associated with response to anti-PD-1 inhibitors (Del Re et al., 2018; Zhao Z. et al., 2019).
Collectively, ExoPD-L1, including PD-L1 protein, DNA and mRNA, has the potential to become reliable biomarkers for immunotherapy. This is an effective complement to tumor PD-L1 and soluble PD-L1 to identify patients who may benefit from immunotherapy and to dynamically monitor therapeutic response (Chen G. et al., 2018; Theodoraki et al., 2019; Cordonnier et al., 2020; Daassi et al., 2020).
The regulation of PD-L1 expression is highly intricate and has been extensively addressed at transcriptional, posttranscriptional, translational, and posttranslational levels (Sun et al., 2018; Zerdes et al., 2018; Cha et al., 2019; Xu Y. et al., 2019; Fu et al., 2020; Han et al., 2020; Ju et al., 2020). Notably, soluble PD-L1 may be generated from ectodomain shedding mediated by either matrix metalloproteinases (MMPs) or a disintegrin and metalloproteases (ADAMs). Additionally, soluble PD-L1 may be produced by alternative splice variants omitting transmembrane domain (Dezutter-Dambuyant et al., 2016; Hira-Miyazawa et al., 2018; Aguirre et al., 2020; Orme et al., 2020; Romero et al., 2020). Although soluble PD-L1 is found in human serum and is regarded as a liquid biopsy predictor, whether soluble PD-L1 can deliver a regulatory signal through PD-1 remains elusive (Gu et al., 2018; Takeuchi et al., 2018; Abu Hejleh et al., 2019; Asanuma et al., 2020). Some studies reported that soluble PD-L1 inhibits T cell activation, while others suggested that soluble PD-L1 is likely to increase immune response by proteolytic reducing the amount of membrane-bound PD-L1 on both cell surface and exosome or by competing with membrane-bound PD-L1 for PD-1 binding (Dezutter-Dambuyant et al., 2016; Hira-Miyazawa et al., 2018; Aguirre et al., 2020; Romero et al., 2020). Furthermore, it is demonstrated that soluble PD-L1 produced by CD274-L2A splice variant lacks suppressive activity and functions as a PD-1 antagonist, suggesting the possibility that soluble PD-L1 might limit the immunoinhibitory effects of ExoPD-L1 (Wan et al., 2006; Steidl et al., 2011; Ng et al., 2019).
Additionally, PD-L2 is also expressed on tumor cells and involved in antitumor immune suppression (Latchman et al., 2001; Taube et al., 2014; Yearley et al., 2017; Larsen et al., 2019; Liao et al., 2019; Tanegashima et al., 2019; Nakayama et al., 2020). Moreover, PD-L2 not only possesses higher affinity for PD-1 than PD-L1 does but also may be highly coexpressed with PD-L1 in tumor cells and tissues (Youngnak et al., 2003; Cheng et al., 2013; Morales-Betanzos et al., 2017; Tang and Kim, 2019; Furuse et al., 2020; Wolkow et al., 2020). It is worth mentioning that proteolytic degradation or alternative splice variants also produce soluble PD-L2, which may be a complementary biomarker (He et al., 2004; Dai et al., 2014; Fukasawa et al., 2017; Costantini et al., 2018; Buderath et al., 2019; Wang Q. et al., 2019). Recently, a pilot study found PD-L2-expressing EVs in a murine sepsis model (Kawamoto et al., 2019). Moreover, sepsis patients displayed higher PD-L2 expression on EVs compared with healthy subjects (Kawamoto et al., 2019). Additionally, reduced exosomal PD-L2 was observed in IL-10-treated murine dendritic cells (Ruffner et al., 2009). However, it is unclear whether tumor cells are able to release exosomal PD-L2, and the function and regulation of exosomal PD-L2 in antitumor immunity are unexplored and worthy of further investigations (Solinas et al., 2020).
Potential Methods for the Detection of Circulating ExoPD-L1 in Clinical Samples
A quick, simple, and sensitive assay is a prerequisite for a point-of-care test for ExoPD-L1 as a clinical biomarker. However, due to the small size and high heterogeneity of exosomes, the methods used most widely to detect ExoPD-L1 from tumor patients required ultracentrifugation and ELISA (Table 3). Therefore, low efficiency and sensitivity are two bottlenecks to the classic detection of ExoPD-L1 in the clinic (Liu et al., 2017; Yang et al., 2017). Efforts have been made to improve the sensitivity of the ELISA-based methods for detecting low levels of ExoPD-L1 (Liu et al., 2018a, b; Huang et al., 2020).
Ultracentrifugation-Based Methods
Recently, a homogeneous, low-volume, efficient, and sensitive ExoPD-L1 (HOLMES-ExoPD-L1) quantitation method has been developed (Huang et al., 2020). The HOLMES-ExoPD-L1 method combining PD-L1 aptamer with separation-free thermophoresis exhibits higher sensitivity and is more rapid than the classic ELISA-based methods (Lee et al., 2019; Huang et al., 2020). To completely surmount the disadvantages of ELISA, a nanoplasmonic exosome (nPLEX) assay has been established, which involves modified surface plasmon resonance (Enderle et al., 2015) with a compact SPR biosensor (Liu et al., 2018b). Notably, the nPLEX assay is able to detect ExoPD-L1 in 50 μl serum samples in real-time, which is undetectable by ELISA (Table 3; Liu et al., 2018b).
Ultracentrifugation-Free Method
The methods described above are ultracentrifugation-based, time-consuming and yield low recovery (Momen-Heravi et al., 2013; Lobb et al., 2015). More recently, a quick and precise method for detecting ExoPD-L1 directly from clinical samples has been set up by coupling Fe3O4@TiO2 isolation with a surface-enhanced Raman scattering (SERS) immunoassay (Pang et al., 2020). Although it takes less than 40 min to complete the entire procedure, the separation efficiency for exosomes is 96.5% and the detection limit is one PD-L1+ exosome per microliter (Pang et al., 2020). Moreover, the number of ExoPD-L1 molecules in four μl of a patient’s serum is precisely quantified using this method (Pang et al., 2020). Overall, along with the advancement of novel technologies, there should have more methods in development. It should be noted that it is necessary to validate these methods in large cohorts before routinely using ExoPD-L1 as a clinical biomarker (Table 3).
Potential Strategies Targeting ExoPD-L1 for Antitumor Therapy
Antibody blockade of PD-L1 is able to trigger an antitumor immune response, bringing about a persistent remission in a fraction of tumor patients. Recent studies have shown that the removal of ExoPD-L1 blocks tumor growth, even in mouse models which are resistant to anti-PD-L1 antibody (Chen G. et al., 2018; Yang et al., 2018; Kim et al., 2019; Poggio et al., 2019; Xie F. et al., 2019). This indicates that targeting exosome biogenesis inhibition and PD-L1 deletion represents an unexplored strategy for antitumor therapy.
Blockade of Exosome Biogenesis Provides an Efficient Way to Overcome Resistance to Anti-PD-L1 Antibody
Immune checkpoint inhibitors are effective against various cancers, including melanoma, NSCLC, and renal cancer. However, the overall response rate in patients treated with anti-PD-1/PD-L1 antibodies is low (Page et al., 2014). In some tumor types, such as prostate cancer, the number of responders is very limited (Goswami et al., 2016; Sharma et al., 2017). However, genetic deletion of PD-L1 in TRAMP-C2 prostate cancer cells, which causes a reduction of both cell-surface PD-L1 and ExoPD-L1, strikingly prevents anti-PD-L1 antibody resistant tumor to grow in mice (Foster et al., 1997; Poggio et al., 2019). More importantly, the prostate cancer cells were also unable to grow in mice when Rab27a or aSNase2 was deleted by the CRISPR/Cas9 technique, leading to a blockade of exosome biogenesis (Poggio et al., 2019). Thus, targeting the process of exosome biogenesis may yield new approaches to overcoming tumor resistance to anti-PD-L1 antibodies.
The biogenesis of ExoPD-L1 is a complicated process involving multiple molecules that impact the production of ExoPD-L1 in different ways (Chen G. et al., 2018; Monypenny et al., 2018; Yang et al., 2018; Poggio et al., 2019). The endosomal sorting complex required for transport complex (ESCRT) is a key mediator of MVB biogenesis (Henne et al., 2011; Matusek et al., 2014; Olmos and Carlton, 2016; Schoneberg et al., 2017; Furthauer, 2018). Hepatocyte growth factor-regulated tyrosine kinase substrate (HRS) is a subunit of ESCRT that mediates the recognition and sorting of exosomal cargos (Schmidt and Teis, 2012). Genetic deletion of HRS leads to a decrease in ExoPD-L1 levels but an increase in cellular PD-L1 levels. The effect of HRS blockade on tumor growth remains unknown (Chen G. et al., 2018). Apoptosis-linked gene 2-interacting protein X (ALIX), an ESCRT accessory protein, is a critical regulator potentially involved in the redistribution of PD-L1 between exosomes and cell-surface membranes (Baietti et al., 2012; Hurley and Odorizzi, 2012; Bissig and Gruenberg, 2014; Christ et al., 2017; Monypenny et al., 2018; Skowyra et al., 2018; Szymanska et al., 2018). Similar to nSMase2 or Rab27a deletion, ALIX knockdown also leads to a significant decrease in ExoPD-L1 production in breast cancer (Monypenny et al., 2018). However, in contrast to Rab27a or nSMase2 deletion, ALIX knockdown promotes, but does not suppress the tumor growth (Monypenny et al., 2018). The differential effects of these genetic deletions on cell-surface PD-L1 expression contribute to their different effects on tumor growth. Deletion of nSMase2 leads to a reduction in the levels of both cellular PD-L1 and ExoPD-L1 protein by downregulating the transcription of the PD-L1 gene (Poggio et al., 2019). Meanwhile, Rab27a deletion does not change cell-surface PD-L1 levels but causes a greater inhibition in exosome production compared with nSMase2 deletion (Poggio et al., 2019). Therefore, deletion of either nSMase2 or Rab27a completely inhibits tumor growth (Yang et al., 2018; Poggio et al., 2019). In contrast, ALIX knockdown leads to a significant increase in cell-surface PD-L1 on breast cancer cells, and thereby increases the aggressiveness of tumors (Monypenny et al., 2018). Collectively, the distribution of PD-L1 between exosomes and cell surfaces is pivotal for the efficacy of immunotherapy. Both ExoPD-L1 and cell-surface PD-L1 should be the focus of future therapeutic strategies (Figure 2).
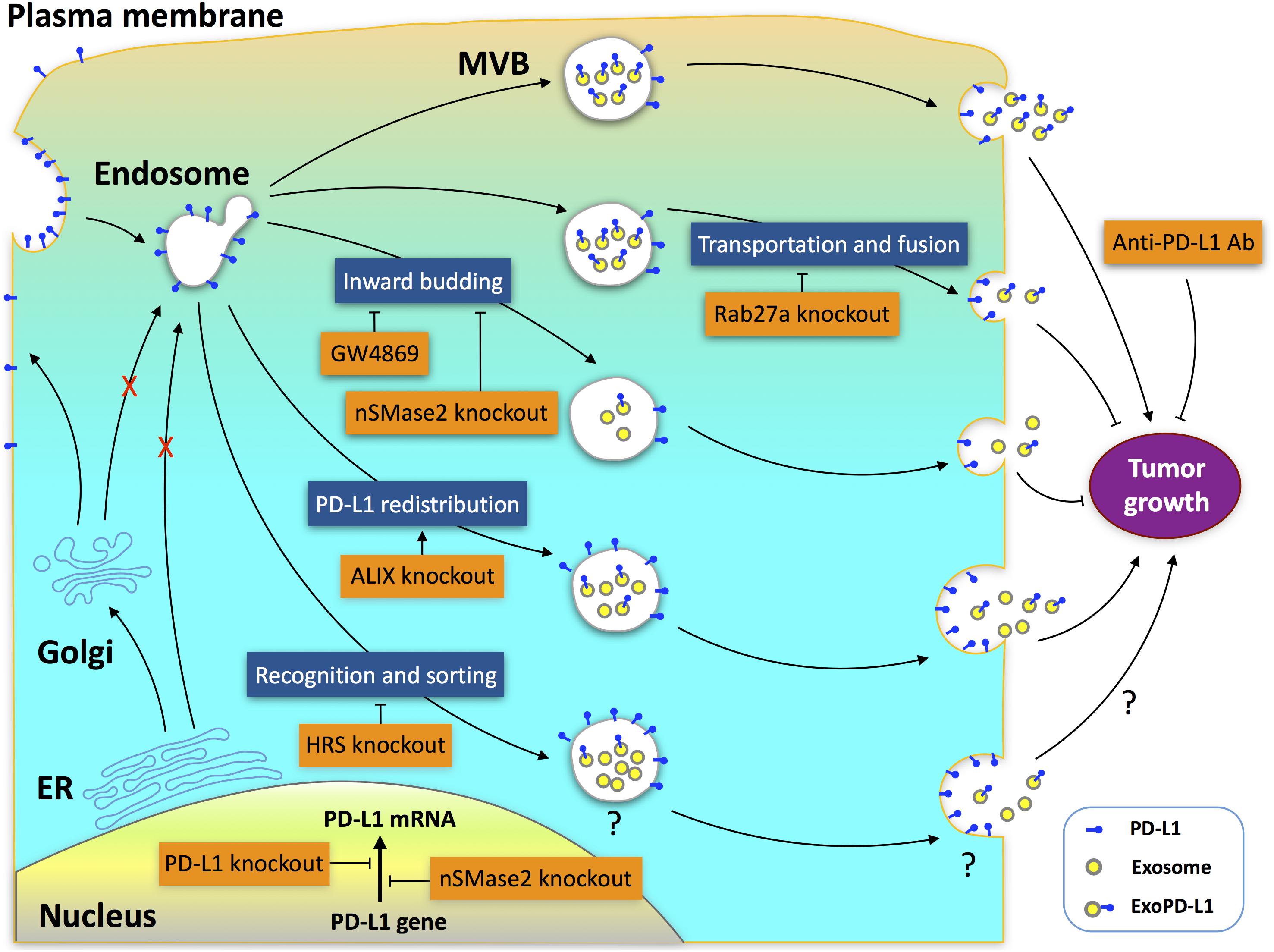
Figure 2. Potential targets for antitumor therapy in ExoPD-L1 biogenesis pathways. Multiple molecules, including Rab27a, nSMase2, ALIX, and HRS, participate in the complex processes of ExoPD-L1 biogenesis, which originates from the cell surface rather than from the ER or Golgi apparatus. Deletion of Rab27a decreases ExoPD-L1, but does not alter cell-surface PD-L1 levels. Deletion of nSMase2 reduces the levels of both cellular PD-L1 and ExoPD-L1 protein. Rab27a deletion causes a greater inhibition in exosome production compared with nSMase2 deletion, while nSMase2 deletion leads to a greater inhibition of ExoPD-L1 production compared with Rab27a deletion. GW4869, an inhibitor of nSMase2, inhibits ExoPD-L1 generation, but does not increase cellular PD-L1 levels. Knockdown of ALIX, which redistributes PD-L1 between the cell-surface and exosomes, results in a reduction of ExoPD-L1 production but an increase in cell-surface PD-L1. Blockade of Rab27a or nSMase2 results in suppression, whereas ALIX knockdown promotes tumor growth. Knockdown of HRS, an ESCRT-0 subunit, confers a decrease in ExoPD-L1 levels but an increase in cellular PD-L1 levels. The effects of HRS knockdown on the cell-surface PD-L1 levels and tumor growth remain unknown.
Combination of Exosome Biogenesis Inhibition With Anti-PD-L1 Antibody Enhances Immunotherapy Efficacy
It is a concern that the antitumor effect of removing ExoPD-L1 is not limited to the anti-PD-L1 resistant model of prostate cancer. Both Rab27a depletion and an nSMase2 inhibitor (GW4869) significantly inhibit the growth of breast cancer derived from 4T1 cells in mice, which is a drug-resistant model for breast cancer (Pulaski and Ostrand-Rosenberg, 2001; Lasso et al., 2019). These findings indicate that the blockade of exosome secretion is an effective tool to disrupt the growth of various tumors (Grasselly et al., 2018; Yang et al., 2018). Importantly, Rab27a knockdown and nSMase2 inhibition are more potent suppressors of breast cancer growth compared with anti-PD-L1 antibody treatment (Yang et al., 2018). More importantly, blocking either Rab27a or sMSase2 markedly enhances the therapeutic effectiveness of anti-PD-L1 antibody for the inhibition of breast cancer growth (Yang et al., 2018). Thus, combining exosome biogenesis inhibition with anti-PD-L1 antibody may be more potent for tumor suppression.
Rab27a knockout suppressed colorectal cancer growth and extended survival in MC38 mice, which is a colorectal cancer model exhibiting a partial response to anti-PD-L1 therapy (Deng et al., 2014; Poggio et al., 2019). In contrast to the resistant TRAMP-C2 prostate cancer model, either Rab27a knockout or anti-PD-L1 antibody blockade exhibited less of an effect on the MC38 colorectal cancer growth compared with PD-L1 genetic deletion (Poggio et al., 2019). However, the combination of exosome deletion with anti-PD-L1 antibody lengthened the lifespan of mice burdened with colorectal cancer to an extent similar to that of PD-L1 deletion (Poggio et al., 2019). Hence, ExoPD-L1 appears to impose an additional, but not redundant impact compared with anti-PD-L1 antibody on the suppression of tumor growth (Chen G. et al., 2018; Poggio et al., 2019). In addition, combined genetic deletion of Rab27a and PD-L1 showed a similar inhibition of tumor growth as compared with PD-L1 deletion, demonstrating that the inhibitory effect of exosomes on colorectal cancer growth occurs mainly through the deletion of ExoPD-L1 (Poggio et al., 2019). Thus, the combination of inhibitors targeting exosome secretion with anti-PD-L1 blockade targeting cell-surface PD-L1 may be a promising strategy to effectively suppress tumor growth in the clinic (Yang et al., 2018; Poggio et al., 2019).
ExoPD-L1-Deficient Tumor Cells Induce Abscopal Effect and Antitumor Immune Memory
The abscopal effect refers to that treatment of a local tumor leads to the regression of distant tumors (Postow et al., 2012; Demaria and Formenti, 2020; Fionda et al., 2020; Mondini et al., 2020). This represents a promising therapeutic strategy for tumors and has drawn increased attention (Liu et al., 2018; Ngwa et al., 2018; Rodriguez-Ruiz et al., 2018; Choi et al., 2020). Interestingly, blocking ExoPD-L1 suppresses the growth of not only the local tumor, but also tumors at a distant site (Poggio et al., 2019). In the TRAMP-C2 mouse prostate cancer model, mutant cancer cells devoid of Rab27a, nSMase2, or PD-L1 expression completely failed to grow (Chen G. et al., 2018; Yang et al., 2018; Poggio et al., 2019). Surprisingly, the growth of WT tumor cells was reduced dramatically when the above mutant cells were injected simultaneously in the opposite sides of mice (Poggio et al., 2019). This indicates that ExoPD-L1-deficient tumor cells induce an abscopal effect on tumor growth (Poggio et al., 2019). On the contrary, WT cells had little or no effect on the growth of the mutant cells (Poggio et al., 2019). Furthermore, the numbers and activities of TILs in WT tumors in mice coinjected with ExoPD-L1-deleted mutant cells were significantly increased in comparison with the mice injected with WT cells alone (Poggio et al., 2019). Thereby, local anti-ExoPD-L1 treatment is able to induce a durable immune response to suppress the growth of tumors at distant sites (Figure 3).
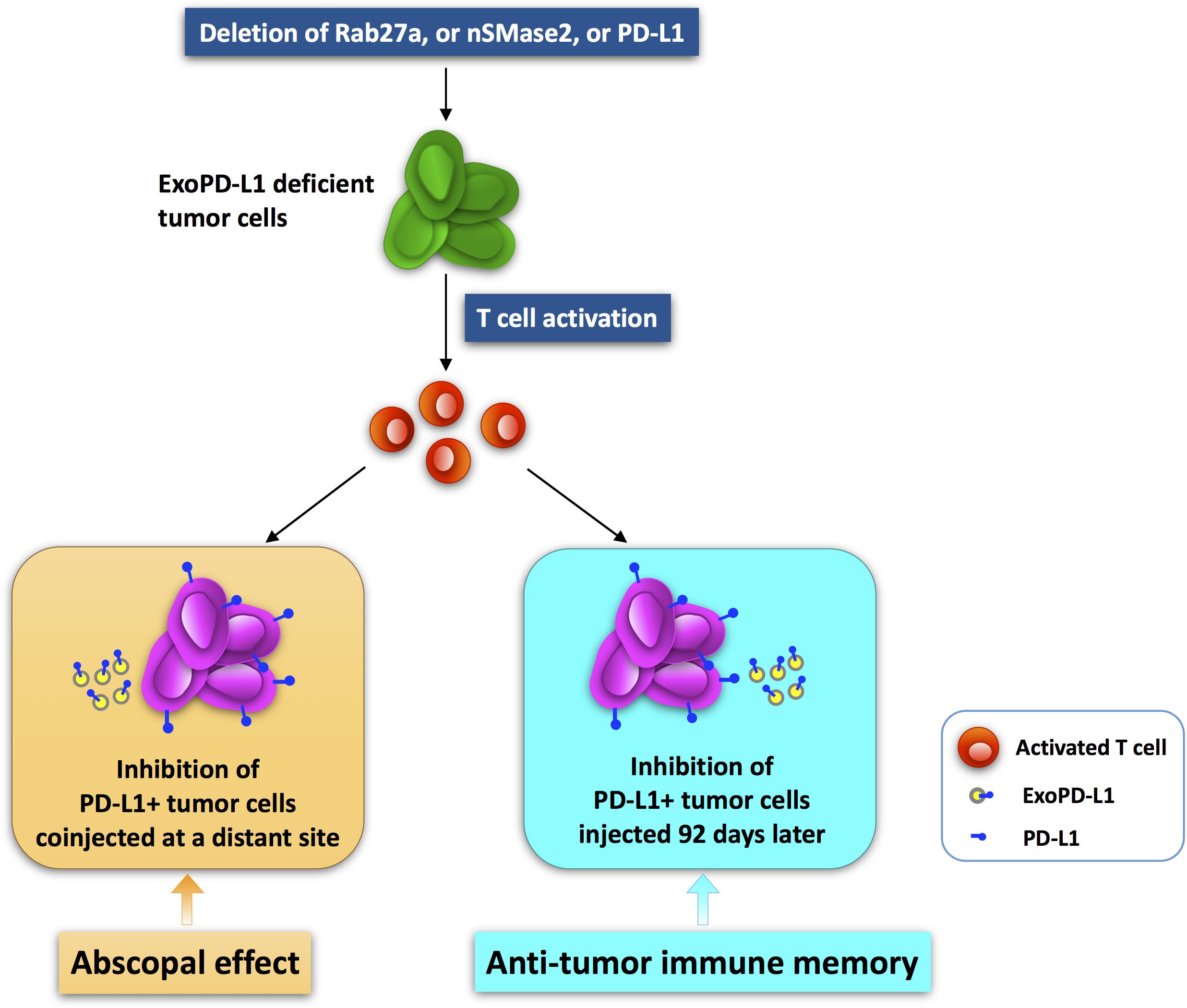
Figure 3. The abscopal effect and antitumor immune memory induced by ExoPD-L1-deficient tumor cells. Tumor cells with ExoPD-L1 deletion are generated by genetic mutation of Rab27a, nSMase2, or PD-L1. The growth of PD-L1-positive tumors at a distant site is inhibited when ExoPD-L1-deleted tumor cells are coinjected simultaneously. In addition, the growth of PD-L1-positive tumors injected secondarily 92 days later is also suppressed.
Additionally, in the prostate cancer model, the mice injected with mutant cells deleted of PD-L1, Rab27a, or nSMase2 survived more than 90 days, whereas the mice injected with WT cells died soon or had to be euthanized because of tumors greater than 2 cm in diameter (Poggio et al., 2019). At 92 days after primary injection with mutant cells, surviving mice were reinjected with WT cells on the opposite flank. Interestingly, the WT prostate cancer cells were unable to grow in the mice that were preinjected with mutant cells (Poggio et al., 2019). In contrast, they grew normally in mice that were not preinjected with mutant cells (Poggio et al., 2019). Therefore, local import of tumor cells lacking of ExoPD-L1 induced a strong antitumor memory response against secondarily challenged tumor cells that secrete ExoPD-L1 (Poggio et al., 2019). In summary, the anti-ExoPD-L1 therapy that targets the tumor at one site may be of clinical significance due to the triggering of a systemic and durable immune response against tumors at multiple sites or challenged secondarily (Figure 3).
Currently, a dozen of small molecules have been recognized as exosome inhibitors (Luberto et al., 2002; Johnson et al., 2016; Catalano and O’Driscoll, 2020). GW4869 and Nexinhib-20 are inhibitors of nSMase2 and Rab27a, respectively (Luberto et al., 2002; Johnson et al., 2016). Moreover, Yang et al. reported that GW4869 inhibited exosome secretion of MDA-MB-231 human breast tumor cells in vitro and 4T1 mouse mammary tumor cells in vitro and in vivo (Yang et al., 2018). However, neither GW4869 nor Nexinhib-20 are able to inhibit exosome release in other tumor cell lines (Phuyal et al., 2014), although genetic deletion of either nSMase2 or Rab27a leads to tremendous loss of exosome secretion (Poggio et al., 2019). It has been established that exosomes participate in a variety of physiological processes and can be released from a variety of cell types (Colombo et al., 2014; Lo Cicero et al., 2015; van Niel et al., 2018; Catalano and O’Driscoll, 2020). It is likely that exosome inhibitors might interfere with normal cell functions by affecting the exosome release from healthy cells (Kalluri, 2016; Hessvik and Llorente, 2018; van Niel et al., 2018; Catalano and O’Driscoll, 2020; Hassanpour et al., 2020). Thus, it should be noted that exploitation of exosome inhibitors for tumor immunotherapy should be conducted with caution due to the potential adverse effects on healthy tissues (Dinkins et al., 2014). In addition, similar to immune checkpoint inhibitors, the restoration of T cell activation mediated by ExoPD-L1 blockade is non-specific and may result in immune-related adverse events (Cuzzubbo et al., 2017; Wanchoo et al., 2017; Barroso-Sousa et al., 2018; Myers, 2018; Sandigursky and Mor, 2018; Sibaud, 2018; Varricchi et al., 2018; Fan et al., 2020; Gauci et al., 2020; Liu T. et al., 2020; Ueki et al., 2020; Williams et al., 2020). Collectively, exosome inhibitors that selectively target cancer cells need to be developed to maximize the antitumor immune responses and minimize the possible side effects of blocking ExoPD-L1 release (Nagai and Muto, 2018; Weinmann and Pisetsky, 2019).
Concluding Remarks
Exosomal PD-L1 derived from tumors is able to suppress antitumor immunity locally and systemically through ligation of PD-1 on T cells, which facilitates immune escape and tumor progression. Additionally, circulating ExoPD-L1 is emerging as a liquid biopsy biomarker for diagnosis, prognosis, stratifying eligible patients, and real-time monitoring of clinical response. Furthermore, the therapeutic strategies targeting ExoPD-L1 are potentially promising by inhibiting the biogenesis of PD-L1-expressing exosomes, and inducing the abscopal effect and antitumor memory response.
However, many issues remain to be resolved. First, understanding the mechanism by which cytokines regulate the biogenesis of ExoPD-L1 is needed. It has been reported that IFN-γ enhances ExoPD-L1 secretion by multiple tumors (Chen G. et al., 2018; Mimura et al., 2018; Monypenny et al., 2018; Poggio et al., 2019). Moreover, there is crosstalk between IFN-γ and epidermal growth factor in the regulation of the distribution of ExoPD-L1 and cellular PD-L1 in breast cancer cells (Monypenny et al., 2018). Recent studies have revealed that both cell-surface PD-L1 and ExoPD-L1 play crucial roles in immunosuppression, tumor progression, and response to cancer immunotherapy (Zou et al., 2016; Chen G. et al., 2018; Theodoraki et al., 2018b; Fan et al., 2019; Kim et al., 2019; Xie F. et al., 2019; Cordonnier et al., 2020; Huang et al., 2020; Tang et al., 2020). Nevertheless, the manner in which inflammatory cytokines affect PD-L1 expression on tumor cells and exosomes is still elusive (Akbay et al., 2013; Chen et al., 2015, 2019; Wang X. et al., 2017; Li et al., 2018; Lin et al., 2020). Second, studies regarding the origin of the PD-L1 nucleic acids in exosomes and their function in antitumor immunity are required. PD-L1 mRNA and DNA are found in exosomes derived from tumor cells in addition to PD-L1 protein (Del Re et al., 2018; Lubin et al., 2018; Ricklefs et al., 2018). It has been reported that RNA in cancer-derived exosomes is also relevant to the local and systemic interaction of exosomes with target cells (Skog et al., 2008; Matei et al., 2017; Yang et al., 2020). However, little is known about the role that ExoPD-L1 nucleic acids play, which should be addressed in the future. Third, immune cells and other cells in the tumor microenvironment or outside of the tumor also express PD-L1 and release exosomes. Moreover, the PD-L1 expression on immune cells is differently regulated and has an important impact on anticancer immunity (Kowanetz et al., 2018). However, the dynamics of host cell-derived ExoPD-L1 production and its potential function in immunosuppression remain unclear (Chen S. et al., 2018; Choo et al., 2018; Zhou et al., 2018; Carreras-Planella et al., 2019; Hong et al., 2019; Lan et al., 2019; Wang X. et al., 2019). Recent studies of murine tumor models indicate that PD-L1 expressed on host cells rather than on tumor cells is the primary target for immunotherapy, and determines the efficacy of the PD-1/PD-L1 blockade (Lin et al., 2018; Tang et al., 2018). Thereby, the immunoregulatory impact of ExoPD-L1 produced by host cells, such as T and B cells, macrophages, dendritic cells, epithelial cells, and mesenchymal stem cells, should be addressed. Finally, determining the regulatory role of ExoPD-L1 on various PD-1-expressing immune cells is of significant interest. In addition to T cells, PD-1 is also expressed on natural killer cells, macrophages, and dendritic cells, which are enriched in the tumor microenvironment (Karyampudi et al., 2016; Gordon et al., 2017; Hsu et al., 2018; Mariotti et al., 2019). Hence, the effect mediated by ExoPD-L1 on a group of PD-1-positive immune cells is a highly relevant issue in tumor immunology. We believe that a better understanding of the regulatory roles of ExoPD-L1 in host resistance to immunotherapies will offer novel therapeutic strategies for cancer patients in the future (Shergold et al., 2019).
Author Contributions
KZ and FL were major contributors in searching the literature and writing the manuscript. SG and GL reviewed the manuscript and provided significant revisions. QS gave guidance for figures. All authors read and approved the final manuscript.
Funding
This work was supported by grants from the National Natural Science Foundation of China (No. 51872332) and the National Natural Science Foundation of Liaoning Province (No. 20170541040).
Conflict of Interest
The authors declare that the research was conducted in the absence of any commercial or financial relationships that could be construed as a potential conflict of interest.
References
Abu Hejleh, T., Furqan, M., Ballas, Z., and Clamon, G. (2019). The clinical significance of soluble PD-1 and PD-L1 in lung cancer. Crit. Rev. Oncol. Hematol. 143, 148–152. doi: 10.1016/j.critrevonc.2019.08.009
Aguiar, P. N. Jr., De Mello, R. A., Hall, P., Tadokoro, H., and Lima Lopes, G. (2017). PD-L1 expression as a predictive biomarker in advanced non-small-cell lung cancer: updated survival data. Immunotherapy 9, 499–506. doi: 10.2217/imt-2016-0150
Aguirre, J. E., Beswick, E. J., Grim, C., Uribe, G., Tafoya, M., Chacon Palma, G., et al. (2020). Matrix metalloproteinases cleave membrane-bound PD-L1 on CD90+ (myo-)fibroblasts in Crohn’s disease and regulate Th1/Th17 cell responses. Int. Immunol. 32, 57–68. doi: 10.1093/intimm/dxz060
Ahn, E., Araki, K., Hashimoto, M., Li, W., Riley, J. L., Cheung, J., et al. (2018). Role of PD-1 during effector CD8 T cell differentiation. Proc. Natl. Acad. Sci. U.S.A. 115, 4749–4754. doi: 10.1073/pnas.1718217115
Ai, L., Xu, A., and Xu, J. (2020). Roles of PD-1/PD-L1 Pathway: signaling. Cancer, and Beyond. Adv. Exp. Med. Biol. 1248, 33–59. doi: 10.1007/978-981-15-3266-5_3
Akbay, E. A., Koyama, S., Carretero, J., Altabef, A., Tchaicha, J. H., Christensen, C. L., et al. (2013). Activation of the PD-1 pathway contributes to immune escape in EGFR-driven lung tumors. Cancer Discov. 3, 1355–1363. doi: 10.1158/2159-8290.CD-13-0310
Alsaab, H. O., Sau, S., Alzhrani, R., Tatiparti, K., Bhise, K., Kashaw, S. K., et al. (2017). PD-1 and PD-L1 checkpoint signaling inhibition for cancer immunotherapy: mechanism, combinations, and clinical outcome. Front. Pharmacol. 8:561. doi: 10.3389/fphar.2017.00561
Anastasiadou, E., and Slack, F. J. (2014). Cancer. Malicious exosomes. Science 346, 1459–1460. doi: 10.1126/science.aaa4024
Anderson, A. C., Joller, N., and Kuchroo, V. K. (2016). Lag-3, Tim-3, and TIGIT: co-inhibitory receptors with specialized functions in immune regulation. Immunity 44, 989–1004. doi: 10.1016/j.immuni.2016.05.001
Ando, K., Hamada, K., Watanabe, M., Ohkuma, R., Shida, M., Onoue, R., et al. (2019). Plasma Levels of Soluble PD-L1 correlate with tumor regression in patients with lung and gastric cancer treated with immune checkpoint inhibitors. Anticancer Res. 39, 5195–5201. doi: 10.21873/anticanres.13716
Andrews, L. P., Yano, H., and Vignali, D. A. A. (2019). Inhibitory receptors and ligands beyond PD-1, PD-L1 and CTLA-4: breakthroughs or backups. Nat. Immunol. 20, 1425–1434. doi: 10.1038/s41590-019-0512-0
Asanuma, K., Nakamura, T., Hayashi, A., Okamoto, T., Iino, T., Asanuma, Y., et al. (2020). Soluble programmed death-ligand 1 rather than PD-L1 on tumor cells effectively predicts metastasis and prognosis in soft tissue sarcomas. Sci. Rep. 10:9077. doi: 10.1038/s41598-020-65895-0
Baietti, M. F., Zhang, Z., Mortier, E., Melchior, A., Degeest, G., Geeraerts, A., et al. (2012). Syndecan-syntenin-ALIX regulates the biogenesis of exosomes. Nat. Cell Biol. 14, 677–685. doi: 10.1038/ncb2502
Barroso-Sousa, R., Barry, W. T., Garrido-Castro, A. C., Hodi, F. S., Min, L., Krop, I. E., et al. (2018). Incidence of endocrine dysfunction following the use of different immune checkpoint inhibitor regimens: a systematic review and meta-analysis. JAMA Oncol. 4, 173–182. doi: 10.1001/jamaoncol.2017.3064
Bassanelli, M., Sioletic, S., Martini, M., Giacinti, S., Viterbo, A., Staddon, A., et al. (2018). Heterogeneity of PD-L1 Expression and Relationship with Biology of NSCLC. Anticancer Res. 38, 3789–3796. doi: 10.21873/anticanres.12662
Bastaki, S., Irandoust, M., Ahmadi, A., Hojjat-Farsangi, M., Ambrose, P., Hallaj, S., et al. (2020). PD-L1/PD-1 axis as a potent therapeutic target in breast cancer. Life Sci. 247:117437. doi: 10.1016/j.lfs.2020.117437
Bissig, C., and Gruenberg, J. (2014). ALIX and the multivesicular endosome: ALIX in Wonderland. Trends Cell Biol. 24, 19–25. doi: 10.1016/j.tcb.2013.10.009
Brennan, K., Martin, K., FitzGerald, S. P., O’Sullivan, J., Wu, Y., Blanco, A., et al. (2020). A comparison of methods for the isolation and separation of extracellular vesicles from protein and lipid particles in human serum. Sci. Rep. 10:1039. doi: 10.1038/s41598-020-57497-7
Brody, R., Zhang, Y., Ballas, M., Siddiqui, M. K., Gupta, P., Barker, C., et al. (2017). PD-L1 expression in advanced NSCLC: Insights into risk stratification and treatment selection from a systematic literature review. Lung Cancer 112, 200–215. doi: 10.1016/j.lungcan.2017.08.005
Buderath, P., Schwich, E., Jensen, C., Horn, P. A., Kimmig, R., Kasimir-Bauer, S., et al. (2019). Soluble programmed death receptor ligands sPD-L1 and sPD-L2 as liquid biopsy markers for prognosis and platinum response in epithelial ovarian cancer. Front. Oncol. 9:1015. doi: 10.3389/fonc.2019.01015
Buder-Bakhaya, K., and Hassel, J. C. (2018). Biomarkers for clinical benefit of immune checkpoint inhibitor treatment-A review from the melanoma perspective and beyond. Front. Immunol. 9:1474. doi: 10.3389/fimmu.2018.01474
Butte, M. J., Keir, M. E., Phamduy, T. B., Sharpe, A. H., and Freeman, G. J. (2007). Programmed death-1 ligand 1 interacts specifically with the B7-1 costimulatory molecule to inhibit T cell responses. Immunity 27, 111–122. doi: 10.1016/j.immuni.2007.05.016
Butte, M. J., Pena-Cruz, V., Kim, M. J., Freeman, G. J., and Sharpe, A. H. (2008). Interaction of human PD-L1 and B7-1. Mol. Immunol. 45, 3567–3572. doi: 10.1016/j.molimm.2008.05.014
Carlton, J. (2010). The ESCRT machinery: a cellular apparatus for sorting and scission. Biochem. Soc. Trans. 38, 1397–1412. doi: 10.1042/BST0381397
Carreras-Planella, L., Monguio-Tortajada, M., Borras, F. E., and Franquesa, M. (2019). Immunomodulatory effect of MSC on B cells is independent of secreted extracellular vesicles. Front. Immunol. 10:1288. doi: 10.3389/fimmu.2019.01288
Catalano, M., and O’Driscoll, L. (2020). Inhibiting extracellular vesicles formation and release: a review of EV inhibitors. J. Extracell. Vesicles 9:1703244. doi: 10.1080/20013078.2019.1703244
Cha, J. H., Chan, L. C., Li, C. W., Hsu, J. L., and Hung, M. C. (2019). Mechanisms Controlling PD-L1 expression in cancer. Mol. Cell 76, 359–370. doi: 10.1016/j.molcel.2019.09.030
Chamoto, K., Hatae, R., and Honjo, T. (2020). Current issues and perspectives in PD-1 blockade cancer immunotherapy. Int. J. Clin. Oncol. 25, 790–800. doi: 10.1007/s10147-019-01588-7
Chang, B., Huang, T., Wei, H., Shen, L., Zhu, D., He, W., et al. (2019). The correlation and prognostic value of serum levels of soluble programmed death protein 1 (sPD-1) and soluble programmed death-ligand 1 (sPD-L1) in patients with hepatocellular carcinoma. Cancer Immunol. Immunother. 68, 353–363. doi: 10.1007/s00262-018-2271-4
Chatterjee, J., Dai, W., Aziz, N. H. A., Teo, P. Y., Wahba, J., Phelps, D. L., et al. (2017). Clinical use of programmed cell death-1 and its ligand expression as discriminatory and predictive markers in ovarian cancer. Clin. Cancer Res. 23, 3453–3460. doi: 10.1158/1078-0432.CCR-16-2366
Chaudhri, A., Xiao, Y., Klee, A. N., Wang, X., Zhu, B., and Freeman, G. J. (2018). PD-L1 Binds to B7-1 only in Cis on the same cell surface. Cancer Immunol. Res. 6, 921–929. doi: 10.1158/2326-6066.CIR-17-0316
Chemnitz, J. M., Parry, R. V., Nichols, K. E., June, C. H., and Riley, J. L. (2004). SHP-1 and SHP-2 associate with immunoreceptor tyrosine-based switch motif of programmed death 1 upon primary human T cell stimulation, but only receptor ligation prevents T cell activation. J. Immunol. 173, 945–954. doi: 10.4049/jimmunol.173.2.945
Chen, G., Huang, A. C., Zhang, W., Zhang, G., Wu, M., Xu, W., et al. (2018). Exosomal PD-L1 contributes to immunosuppression and is associated with anti-PD-1 response. Nature 560, 382–386. doi: 10.1038/s41586-018-0392-8
Chen, L., and Flies, D. B. (2013). Molecular mechanisms of T cell co-stimulation and co-inhibition. Nat. Rev. Immunol. 13, 227–242. doi: 10.1038/nri3405
Chen, M., Sharma, A., Lin, Y., Wu, Y., He, Q., Gu, Y., et al. (2019). Insluin and epithelial growth factor (EGF) promote programmed death ligand 1(PD-L1) production and transport in colon cancer stem cells. BMC Cancer 19:153. doi: 10.1186/s12885-019-5364-3
Chen, N., Fang, W., Zhan, J., Hong, S., Tang, Y., Kang, S., et al. (2015). Upregulation of PD-L1 by EGFR activation mediates the immune escape in EGFR-Driven NSCLC: implication for optional immune targeted therapy for NSCLC patients with EGFR mutation. J. Thorac. Oncol. 10, 910–923. doi: 10.1097/JTO.0000000000000500
Chen, S., Lv, M., Fang, S., Ye, W., Gao, Y., and Xu, Y. (2018). Poly(I:C) enhanced anti-cervical cancer immunities induced by dendritic cells-derived exosomes. Int. J. Biol. Macromol. 113, 1182–1187. doi: 10.1016/j.ijbiomac.2018.02.034
Cheng, X., Veverka, V., Radhakrishnan, A., Waters, L. C., Muskett, F. W., Morgan, S. H., et al. (2013). Structure and interactions of the human programmed cell death 1 receptor. J. Biol. Chem. 288, 11771–11785. doi: 10.1074/jbc.M112.448126
Chinai, J. M., Janakiram, M., Chen, F., Chen, W., Kaplan, M., and Zang, X. (2015). New immunotherapies targeting the PD-1 pathway. Trends Pharmacol. Sci. 36, 587–595. doi: 10.1016/j.tips.2015.06.005
Choi, J. S., Sansoni, E. R., Lovin, B. D., Lindquist, N. R., Phan, J., Mayo, L. L., et al. (2020). Abscopal effect following immunotherapy and combined stereotactic body radiation therapy in recurrent metastatic head and neck squamous cell carcinoma: a report of two cases and literature review. Ann. Otol. Rhinol. Laryngol. 129, 517–522. doi: 10.1177/0003489419896602
Choo, Y. W., Kang, M., Kim, H. Y., Han, J., Kang, S., Lee, J. R., et al. (2018). M1 macrophage-derived nanovesicles potentiate the anticancer efficacy of immune checkpoint inhibitors. ACS Nano 12, 8977–8993. doi: 10.1021/acsnano.8b02446
Christ, L., Raiborg, C., Wenzel, E. M., Campsteijn, C., and Stenmark, H. (2017). Cellular functions and molecular mechanisms of the ESCRT membrane-scission machinery. Trends Biochem. Sci. 42, 42–56. doi: 10.1016/j.tibs.2016.08.016
Cimino-Mathews, A., Thompson, E., Taube, J. M., Ye, X., Lu, Y., Meeker, A., et al. (2016). PD-L1 (B7-H1) expression and the immune tumor microenvironment in primary and metastatic breast carcinomas. Hum. Pathol. 47, 52–63. doi: 10.1016/j.humpath.2015.09.003
Colombo, M., Raposo, G., and Thery, C. (2014). Biogenesis, secretion, and intercellular interactions of exosomes and other extracellular vesicles. Annu. Rev. Cell Dev. Biol. 30, 255–289. doi: 10.1146/annurev-cellbio-101512-122326
Cordonnier, M., Nardin, C., Chanteloup, G., Derangere, V., Algros, M. P., Arnould, L., et al. (2020). Tracking the evolution of circulating exosomal-PD-L1 to monitor melanoma patients. J. Extracell. Vesicles 9:1710899. doi: 10.1080/20013078.2019.1710899
Costantini, A., Julie, C., Dumenil, C., Helias-Rodzewicz, Z., Tisserand, J., Dumoulin, J., et al. (2018). Predictive role of plasmatic biomarkers in advanced non-small cell lung cancer treated by nivolumab. Oncoimmunology 7:e1452581. doi: 10.1080/2162402X.2018.1452581
Couzin-Frankel, J. (2013). Breakthrough of the year 2013. Cancer immunotherapy. Science 342, 1432–1433. doi: 10.1126/science.342.6165.1432
Crow, J., Samuel, G., and Godwin, A. K. (2019). Beyond tumor mutational burden: potential and limitations in using exosomes to predict response to immunotherapy. Expert Rev. Mol. Diagn. 19, 1079–1088. doi: 10.1080/14737159.2020.1688144
Cuzzubbo, S., Javeri, F., Tissier, M., Roumi, A., Barlog, C., Doridam, J., et al. (2017). Neurological adverse events associated with immune checkpoint inhibitors: review of the literature. Eur. J. Cancer 73, 1–8. doi: 10.1016/j.ejca.2016.12.001
Czystowska-Kuzmicz, M., Sosnowska, A., Nowis, D., Ramji, K., Szajnik, M., Chlebowska-Tuz, J., et al. (2019). Small extracellular vesicles containing arginase-1 suppress T-cell responses and promote tumor growth in ovarian carcinoma. Nat. Commun. 10:3000. doi: 10.1038/s41467-019-10979-3
Daassi, D., Mahoney, K. M., and Freeman, G. J. (2020). The importance of exosomal PDL1 in tumour immune evasion. Nat. Rev. Immunol. 20, 209–215. doi: 10.1038/s41577-019-0264-y
Dai, S., Jia, R., Zhang, X., Fang, Q., and Huang, L. (2014). The PD-1/PD-Ls pathway and autoimmune diseases. Cell. Immunol. 290, 72–79. doi: 10.1016/j.cellimm.2014.05.006
Davis, A. A., and Patel, V. G. (2019). The role of PD-L1 expression as a predictive biomarker: an analysis of all US Food and Drug Administration (FDA) approvals of immune checkpoint inhibitors. J. Immunother. Cancer 7:278. doi: 10.1186/s40425-019-0768-9
Del Re, M., Marconcini, R., Pasquini, G., Rofi, E., Vivaldi, C., Bloise, F., et al. (2018). PD-L1 mRNA expression in plasma-derived exosomes is associated with response to anti-PD-1 antibodies in melanoma and NSCLC. Br. J. Cancer 118, 820–824. doi: 10.1038/bjc.2018.9
Demaria, S., and Formenti, S. C. (2020). The abscopal effect 67 years later: from a side story to center stage. Br. J. Radiol. 93:20200042. doi: 10.1259/bjr.20200042
Deng, L., Liang, H., Burnette, B., Beckett, M., Darga, T., Weichselbaum, R. R., et al. (2014). Irradiation and anti-PD-L1 treatment synergistically promote antitumor immunity in mice. J. Clin. Invest. 124, 687–695. doi: 10.1172/JCI67313
Dezutter-Dambuyant, C., Durand, I., Alberti, L., Bendriss-Vermare, N., Valladeau-Guilemond, J., Duc, A., et al. (2016). A novel regulation of PD-1 ligands on mesenchymal stromal cells through MMP-mediated proteolytic cleavage. Oncoimmunology 5:e1091146. doi: 10.1080/2162402X.2015.1091146
Dinkins, M. B., Dasgupta, S., Wang, G., Zhu, G., and Bieberich, E. (2014). Exosome reduction in vivo is associated with lower amyloid plaque load in the 5XFAD mouse model of Alzheimer’s disease. Neurobiol. Aging 35, 1792–1800. doi: 10.1016/j.neurobiolaging.2014.02.012
Dong, H., Strome, S. E., Salomao, D. R., Tamura, H., Hirano, F., Flies, D. B., et al. (2002). Tumor-associated B7-H1 promotes T-cell apoptosis: a potential mechanism of immune evasion. Nat. Med. 8, 793–800. doi: 10.1038/nm730
Dong, H., Zhu, G., Tamada, K., and Chen, L. (1999). B7-H1, a third member of the B7 family, co-stimulates T-cell proliferation and interleukin-10 secretion. Nat. Med. 5, 1365–1369. doi: 10.1038/70932
Enderle, D., Spiel, A., Coticchia, C. M., Berghoff, E., Mueller, R., Schlumpberger, M., et al. (2015). Characterization of RNA from exosomes and other extracellular vesicles isolated by a novel spin column-based method. PLoS One 10:e0136133. doi: 10.1371/journal.pone.0136133
Fan, Y., Che, X., Qu, J., Hou, K., Wen, T., Li, Z., et al. (2019). Exosomal PD-L1 retains immunosuppressive activity and is associated with gastric cancer prognosis. Ann. Surg. Oncol. 26, 3745–3755. doi: 10.1245/s10434-019-07431-7
Fan, Y., Geng, Y., Shen, L., and Zhang, Z. (2020). Advances on immune-related adverse events associated with immune checkpoint inhibitors. Front. Med. doi: 10.1007/s11684-019-0735-3 [Epub ahead of print].
Farooqi, A. A., Desai, N. N., Qureshi, M. Z., Librelotto, D. R. N., Gasparri, M. L., Bishayee, A., et al. (2018). Exosome biogenesis, bioactivities and functions as new delivery systems of natural compounds. Biotechnol. Adv. 36, 328–334. doi: 10.1016/j.biotechadv.2017.12.010
Festino, L., Botti, G., Lorigan, P., Masucci, G. V., Hipp, J. D., Horak, C. E., et al. (2016). Cancer treatment with Anti-PD-1/PD-L1 agents: Is PD-L1 expression a biomarker for patient selection? Drugs 76, 925–945. doi: 10.1007/s40265-016-0588-x
Fionda, B., Massaccesi, M., Tagliaferri, L., Dinapoli, N., Iezzi, R., and Boldrini, L. (2020). Abscopal effect and interventional oncology: state of art and future perspectives. Eur. Rev. Med. Pharmacol. Sci. 24, 773–776. doi: 10.26355/eurrev_202001_20058
Foster, B. A., Gingrich, J. R., Kwon, E. D., Madias, C., and Greenberg, N. M. (1997). Characterization of prostatic epithelial cell lines derived from transgenic adenocarcinoma of the mouse prostate (TRAMP) model. Cancer Res. 57, 3325–3330.
Fu, Y., Liu, C. J., Kobayashi, D. K., Johanns, T. M., Bowman-Kirigin, J. A., Schaettler, M. O., et al. (2020). GATA2 Regulates Constitutive PD-L1 and PD-L2 Expression in Brain Tumors. Sci. Rep. 10:9027. doi: 10.1038/s41598-020-65915-z
Fukasawa, T., Yoshizaki, A., Ebata, S., Nakamura, K., Saigusa, R., Miura, S., et al. (2017). Contribution of soluble forms of programmed death 1 and programmed death ligand 2 to disease severity and progression in systemic sclerosis. Arthritis Rheumatol. 69, 1879–1890. doi: 10.1002/art.40164
Furthauer, M. (2018). The ESCRT machinery: When function follows form. Semin. Cell Dev. Biol. 74, 1–3. doi: 10.1016/j.semcdb.2017.11.003
Furuse, M., Kuwabara, H., Ikeda, N., Hattori, Y., Ichikawa, T., Kagawa, N., et al. (2020). PD-L1 and PD-L2 expression in the tumor microenvironment including peritumoral tissue in primary central nervous system lymphoma. BMC Cancer 20:277. doi: 10.1186/s12885-020-06755-y
Gauci, M. L., Baroudjian, B., Bederede, U., Zeboulon, C., Delyon, J., Allayous, C., et al. (2020). Severe immune-related hepatitis induced by immune checkpoint inhibitors: clinical features and management proposal. Clin. Res. Hepatol. Gastroenterol. doi: 10.1016/j.clinre.2020.06.016 [Epub ahead of print].
Gordon, S. R., Maute, R. L., Dulken, B. W., Hutter, G., George, B. M., McCracken, M. N., et al. (2017). PD-1 expression by tumour-associated macrophages inhibits phagocytosis and tumour immunity. Nature 545, 495–499. doi: 10.1038/nature22396
Goswami, S., Aparicio, A., and Subudhi, S. K. (2016). Immune checkpoint therapies in prostate cancer. Cancer J. 22, 117–120. doi: 10.1097/PPO.0000000000000176
Gould, S. J., and Raposo, G. (2013). As we wait: coping with an imperfect nomenclature for extracellular vesicles. J. Extracell. Vesicles 2:20389. doi: 10.3402/jev.v2i0.20389
Grasselly, C., Denis, M., Bourguignon, A., Talhi, N., Mathe, D., Tourette, A., et al. (2018). The antitumor activity of combinations of cytotoxic chemotherapy and immune checkpoint inhibitors is model-dependent. Front. Immunol. 9:2100. doi: 10.3389/fimmu.2018.02100
Gregg, J. P., Li, T., and Yoneda, K. Y. (2019). Molecular testing strategies in non-small cell lung cancer: optimizing the diagnostic journey. Transl. Lung Cancer Res. 8, 286–301. doi: 10.21037/tlcr.2019.04.14
Groot Kormelink, T., Mol, S., de Jong, E. C., and Wauben, M. H. M. (2018). The role of extracellular vesicles when innate meets adaptive. Semin. Immunopathol. 40, 439–452. doi: 10.1007/s00281-018-0681-1
Gu, D., Ao, X., Yang, Y., Chen, Z., and Xu, X. (2018). Soluble immune checkpoints in cancer: production, function and biological significance. J. Immunother. Cancer 6:132. doi: 10.1186/s40425-018-0449-0
Ha, D., Yang, N., and Nadithe, V. (2016). Exosomes as therapeutic drug carriers and delivery vehicles across biological membranes: current perspectives and future challenges. Acta Pharm. Sin. B 6, 287–296. doi: 10.1016/j.apsb.2016.02.001
Han, Y., Liu, D., and Li, L. (2020). PD-1/PD-L1 pathway: current researches in cancer. Am. J. Cancer Res. 10, 727–742.
Hassanpour, M., Rezabakhsh, A., Rezaie, J., Nouri, M., and Rahbarghazi, R. (2020). Exosomal cargos modulate autophagy in recipient cells via different signaling pathways. Cell Biosci. 10:92. doi: 10.1186/s13578-020-00455-7
Hatae, R., Chamoto, K., Kim, Y. H., Sonomura, K., Taneishi, K., Kawaguchi, S., et al. (2020). Combination of host immune metabolic biomarkers for the PD-1 blockade cancer immunotherapy. JCI Insight 5:e133501. doi: 10.1172/jci.insight.133501
He, X. H., Liu, Y., Xu, L. H., and Zeng, Y. Y. (2004). Cloning and identification of two novel splice variants of human PD-L2. Acta Biochim. Biophys. Sin. 36, 284–289. doi: 10.1093/abbs/36.4.284
Henne, W. M., Buchkovich, N. J., and Emr, S. D. (2011). The ESCRT pathway. Dev. Cell 21, 77–91. doi: 10.1016/j.devcel.2011.05.015
Hesari, A., Golrokh Moghadam, S. A., Siasi, A., Rahmani, M., Behboodi, N., Rastgar-Moghadam, A., et al. (2018). Tumor-derived exosomes: potential biomarker or therapeutic target in breast cancer? J. Cell. Biochem. 119, 4236–4240. doi: 10.1002/jcb.26364
Hessvik, N. P., and Llorente, A. (2018). Current knowledge on exosome biogenesis and release. Cell. Mol. Life Sci. 75, 193–208. doi: 10.1007/s00018-017-2595-9
Hira-Miyazawa, M., Nakamura, H., Hirai, M., Kobayashi, Y., Kitahara, H., Bou-Gharios, G., et al. (2018). Regulation of programmed-death ligand in the human head and neck squamous cell carcinoma microenvironment is mediated through matrix metalloproteinase-mediated proteolytic cleavage. Int. J. Oncol. 52, 379–388. doi: 10.3892/ijo.2017.4221
Hong, P., Yang, H., Wu, Y., Li, K., and Tang, Z. (2019). The functions and clinical application potential of exosomes derived from adipose mesenchymal stem cells: a comprehensive review. Stem Cell Res. Ther. 10:242. doi: 10.1186/s13287-019-1358-y
Hood, J. L., San, R. S., and Wickline, S. A. (2011). Exosomes released by melanoma cells prepare sentinel lymph nodes for tumor metastasis. Cancer Res. 71, 3792–3801. doi: 10.1158/0008-5472.CAN-10-4455
Hsu, J., Hodgins, J. J., Marathe, M., Nicolai, C. J., Bourgeois-Daigneault, M. C., Trevino, T. N., et al. (2018). Contribution of NK cells to immunotherapy mediated by PD-1/PD-L1 blockade. J. Clin. Invest. 128, 4654–4668. doi: 10.1172/JCI99317
Huang, M., Yang, J., Wang, T., Song, J., Xia, J., Wu, L., et al. (2020). Homogeneous, low-volume, efficient, and sensitive quantitation of circulating exosomal PD-L1 for cancer diagnosis and immunotherapy response prediction. Angew. Chem. Int. Ed. Engl. 59, 4800–4805. doi: 10.1002/anie.201916039
Huang, Y., Liu, K., Li, Q., Yao, Y., and Wang, Y. (2018). Exosomes function in tumor immune microenvironment. Adv. Exp. Med. Biol. 1056, 109–122. doi: 10.1007/978-3-319-74470-4_7
Hui, E., Cheung, J., Zhu, J., Su, X., Taylor, M. J., Wallweber, H. A., et al. (2017). T cell costimulatory receptor CD28 is a primary target for PD-1-mediated inhibition. Science 355, 1428–1433. doi: 10.1126/science.aaf1292
Hurley, J. H., and Odorizzi, G. (2012). Get on the exosome bus with ALIX. Nat. Cell Biol. 14, 654–655. doi: 10.1038/ncb2530
Jiang, X., Wang, J., Deng, X., Xiong, F., Ge, J., Xiang, B., et al. (2019). Role of the tumor microenvironment in PD-L1/PD-1-mediated tumor immune escape. Mol. Cancer 18:10. doi: 10.1186/s12943-018-0928-4
Jiang, Y., Chen, M., Nie, H., and Yuan, Y. (2019). PD-1 and PD-L1 in cancer immunotherapy: clinical implications and future considerations. Hum. Vaccin Immunother. 15, 1111–1122. doi: 10.1080/21645515.2019.1571892
Johnsen, K. B., Gudbergsson, J. M., Andresen, T. L., and Simonsen, J. B. (2019). What is the blood concentration of extracellular vesicles? Implications for the use of extracellular vesicles as blood-borne biomarkers of cancer. Biochim. Biophys. Acta Rev. Cancer 1871, 109–116. doi: 10.1016/j.bbcan.2018.11.006
Johnson, J. L., Ramadass, M., He, J., Brown, S. J., Zhang, J., Abgaryan, L., et al. (2016). Identification of Neutrophil Exocytosis Inhibitors (Nexinhibs), Small Molecule Inhibitors of Neutrophil Exocytosis and Inflammation: druggability of the small GTPase Rab27a. J. Biol. Chem. 291, 25965–25982. doi: 10.1074/jbc.M116.741884
Ju, X., Zhang, H., Zhou, Z., and Wang, Q. (2020). Regulation of PD-L1 expression in cancer and clinical implications in immunotherapy. Am. J. Cancer Res. 10, 1–11.
Kakavand, H., Rawson, R. V., Pupo, G. M., Yang, J. Y. H., Menzies, A. M., Carlino, M. S., et al. (2017). PD-L1 expression and immune escape in melanoma resistance to MAPK inhibitors. Clin. Cancer Res. 23, 6054–6061. doi: 10.1158/1078-0432.CCR-16-1688
Kalluri, R. (2016). The biology and function of exosomes in cancer. J. Clin. Invest. 126, 1208–1215. doi: 10.1172/JCI81135
Kamphorst, A. O., Pillai, R. N., Yang, S., Nasti, T. H., Akondy, R. S., Wieland, A., et al. (2017). Proliferation of PD-1+ CD8 T cells in peripheral blood after PD-1-targeted therapy in lung cancer patients. Proc. Natl. Acad. Sci. U.S.A. 114, 4993–4998. doi: 10.1073/pnas.1705327114
Karyampudi, L., Lamichhane, P., Krempski, J., Kalli, K. R., Behrens, M. D., Vargas, D. M., et al. (2016). PD-1 blunts the function of ovarian tumor-infiltrating dendritic cells by inactivating NF-kappaB. Cancer Res. 76, 239–250. doi: 10.1158/0008-5472.CAN-15-0748
Kaunitz, G. J., Cottrell, T. R., Lilo, M., Muthappan, V., Esandrio, J., Berry, S., et al. (2017). Melanoma subtypes demonstrate distinct PD-L1 expression profiles. Lab. Invest. 97, 1063–1071. doi: 10.1038/labinvest.2017.64
Kawamoto, E., Masui-Ito, A., Eguchi, A., Soe, Z. Y., Prajuabjinda, O., Darkwah, S., et al. (2019). Integrin and PD-1 ligand expression on circulating extracellular vesicles in systemic inflammatory response syndrome and sepsis. Shock 52, 13–22. doi: 10.1097/SHK.0000000000001228
Khushman, M., Bhardwaj, A., Patel, G. K., Laurini, J. A., Roveda, K., Tan, M. C., et al. (2017). Exosomal Markers (CD63 and CD9) expression pattern using immunohistochemistry in resected malignant and nonmalignant pancreatic specimens. Pancreas 46, 782–788. doi: 10.1097/MPA.0000000000000847
Kim, D. H., Kim, H., Choi, Y. J., Kim, S. Y., Lee, J. E., Sung, K. J., et al. (2019). Exosomal PD-L1 promotes tumor growth through immune escape in non-small cell lung cancer. Exp. Mol. Med. 51, 1–13. doi: 10.1038/s12276-019-0295-2
Kloten, V., Lampignano, R., Krahn, T., and Schlange, T. (2019). Circulating Tumor Cell PD-L1 Expression as Biomarker for Therapeutic Efficacy of Immune Checkpoint Inhibition in NSCLC. Cells 8:809. doi: 10.3390/cells8080809
Kowal, J., Tkach, M., and Thery, C. (2014). Biogenesis and secretion of exosomes. Curr. Opin. Cell Biol. 29, 116–125. doi: 10.1016/j.ceb.2014.05.004
Kowanetz, M., Zou, W., Gettinger, S. N., Koeppen, H., Kockx, M., Schmid, P., et al. (2018). Differential regulation of PD-L1 expression by immune and tumor cells in NSCLC and the response to treatment with atezolizumab (anti-PD-L1). Proc. Natl. Acad. Sci. U.S.A. 115, E10119–E10126. doi: 10.1073/pnas.1802166115
Kythreotou, A., Siddique, A., Mauri, F. A., Bower, M., and Pinato, D. J. (2018). Pd-L1. J. Clin. Pathol. 71, 189–194. doi: 10.1136/jclinpath-2017-204853
Lallemand, T., Rouahi, M., Swiader, A., Grazide, M. H., Geoffre, N., Alayrac, P., et al. (2018). nSMase2 (Type 2-Neutral Sphingomyelinase) Deficiency or Inhibition by GW4869 Reduces Inflammation and Atherosclerosis in Apoe(-/-) Mice. Arterioscler. Thromb. Vasc. Biol. 38, 1479–1492. doi: 10.1161/ATVBAHA.118.311208
Lan, J., Sun, L., Xu, F., Liu, L., Hu, F., Song, D., et al. (2019). M2 macrophage-derived exosomes promote cell migration and invasion in colon cancer. Cancer Res. 79, 146–158. doi: 10.1158/0008-5472.CAN-18-0014
Larsen, T. V., Hussmann, D., and Nielsen, A. L. (2019). PD-L1 and PD-L2 expression correlated genes in non-small-cell lung cancer. Cancer Commun. 39:30. doi: 10.1186/s40880-019-0376-6
Lasso, P., Llano Murcia, M., Sandoval, T. A., Uruena, C., Barreto, A., and Fiorentino, S. (2019). Breast tumor cells highly resistant to drugs are controlled only by the immune response induced in an immunocompetent mouse model. Integr. Cancer Ther. 18:1534735419848047. doi: 10.1177/1534735419848047
Latchman, Y., Wood, C. R., Chernova, T., Chaudhary, D., Borde, M., Chernova, I., et al. (2001). PD-L2 is a second ligand for PD-1 and inhibits T cell activation. Nat. Immunol. 2, 261–268. doi: 10.1038/85330
Lazaro-Ibanez, E., Lasser, C., Shelke, G. V., Crescitelli, R., Jang, S. C., Cvjetkovic, A., et al. (2019). DNA analysis of low- and high-density fractions defines heterogeneous subpopulations of small extracellular vesicles based on their DNA cargo and topology. J. Extracell. Vesicles 8:1656993. doi: 10.1080/20013078.2019.1656993
Lee, H. H., Wang, Y. N., Xia, W., Chen, C. H., Rau, K. M., Ye, L., et al. (2019). Removal of N-Linked Glycosylation Enhances PD-L1 Detection and Predicts Anti-PD-1/PD-L1 Therapeutic Efficacy. Cancer Cell 36, 168–178.e4. doi: 10.1016/j.ccell.2019.06.008
Li, C., Li, C., Zhi, C., Liang, W., Wang, X., Chen, X., et al. (2019a). Clinical significance of PD-L1 expression in serum-derived exosomes in NSCLC patients. J. Transl. Med. 17:355. doi: 10.1186/s12967-019-2101-2
Li, H., van der Leun, A. M., Yofe, I., Lubling, Y., Gelbard-Solodkin, D., van Akkooi, A. C. J., et al. (2019b). Dysfunctional CD8 T cells form a proliferative, dynamically regulated compartment within human melanoma. Cell 176, 775–789.e18. doi: 10.1016/j.cell.2018.11.043
Li, H., Xu, Y., Wan, B., Song, Y., Zhan, P., Hu, Y., et al. (2019c). The clinicopathological and prognostic significance of PD-L1 expression assessed by immunohistochemistry in lung cancer: a meta-analysis of 50 studies with 11,383 patients. Transl. Lung Cancer Res. 8, 429–449. doi: 10.21037/tlcr.2019.08.04
Li, N., Wang, J., Zhang, N., Zhuang, M., Zong, Z., Zou, J., et al. (2018). Cross-talk between TNF-alpha and IFN-gamma signaling in induction of B7-H1 expression in hepatocellular carcinoma cells. Cancer Immunol. Immunother. 67, 271–283. doi: 10.1007/s00262-017-2086-8
Liao, H., Chen, W., Dai, Y., Richardson, J. J., Guo, J., Yuan, K., et al. (2019). Expression of programmed cell death-ligands in hepatocellular carcinoma: correlation with immune microenvironment and survival outcomes. Front. Oncol. 9:883. doi: 10.3389/fonc.2019.00883
Lin, H., Wei, S., Hurt, E. M., Green, M. D., Zhao, L., Vatan, L., et al. (2018). Host expression of PD-L1 determines efficacy of PD-L1 pathway blockade-mediated tumor regression. J. Clin. Invest. 128:1708. doi: 10.1172/JCI120803
Lin, X., Zeng, T., Lin, J., Zhang, Q., Cheng, H., Fang, S., et al. (2020). Establishment of humanized tumor microenvironment mouse models based on the injection of peripheral blood mononuclear cells and IFN-gamma to evaluate the efficacy of PD-L1/PD-1-targeted immunotherapy. Cancer Biol. Ther. 21, 130–138. doi: 10.1080/15384047.2019.1670520
Liu, C., Xu, X., Li, B., Situ, B., Pan, W., Hu, Y., et al. (2018a). Single-exosome-counting immunoassays for cancer diagnostics. Nano Lett. 18, 4226–4232. doi: 10.1021/acs.nanolett.8b01184
Liu, C., Zeng, X., An, Z., Yang, Y., Eisenbaum, M., Gu, X., et al. (2018b). Sensitive detection of exosomal proteins via a compact surface plasmon resonance biosensor for cancer diagnosis. ACS Sens. 3, 1471–1479. doi: 10.1021/acssensors.8b00230
Liu, F., Vermesh, O., Mani, V., Ge, T. J., Madsen, S. J., Sabour, A., et al. (2017). The exosome total isolation chip. ACS Nano 11, 10712–10723. doi: 10.1021/acsnano.7b04878
Liu, S., Zhu, Y., Zhang, C., Meng, X., Sun, B., Zhang, G., et al. (2020). The clinical significance of soluble programmed cell Death-Ligand 1 (sPD-L1) in patients with gliomas. Front. Oncol. 10:9. doi: 10.3389/fonc.2020.00009
Liu, S. Y., and Wu, Y. L. (2019). Biomarker for personalized immunotherapy. Transl. Lung Cancer Res. 8, S308–S317. doi: 10.21037/tlcr.2019.08.02
Liu, T., Jin, B., Chen, J., Wang, H., Lin, S., Dang, J., et al. (2020). Comparative risk of serious and fatal treatment-related adverse events caused by 19 immune checkpoint inhibitors used in cancer treatment: a network meta-analysis. Ther. Adv. Med. Oncol. 12:1758835920940927. doi: 10.1177/1758835920940927
Liu, Y., Dong, Y., Kong, L., Shi, F., Zhu, H., and Yu, J. (2018). Abscopal effect of radiotherapy combined with immune checkpoint inhibitors. J. Hematol. Oncol. 11:104. doi: 10.1186/s13045-018-0647-8
Lo Cicero, A., Stahl, P. D., and Raposo, G. (2015). Extracellular vesicles shuffling intercellular messages: for good or for bad. Curr. Opin. Cell Biol. 35, 69–77. doi: 10.1016/j.ceb.2015.04.013
Lobb, R. J., Becker, M., Wen, S. W., Wong, C. S., Wiegmans, A. P., Leimgruber, A., et al. (2015). Optimized exosome isolation protocol for cell culture supernatant and human plasma. J. Extracell. Vesicles 4:27031. doi: 10.3402/jev.v4.27031
Luberto, C., Hassler, D. F., Signorelli, P., Okamoto, Y., Sawai, H., Boros, E., et al. (2002). Inhibition of tumor necrosis factor-induced cell death in MCF7 by a novel inhibitor of neutral sphingomyelinase. J. Biol. Chem. 277, 41128–41139. doi: 10.1074/jbc.M206747200
Lubin, J. A., Zhang, R. R., and Kuo, J. S. (2018). Extracellular vesicles containing PD-L1 contribute to immune evasion in glioblastoma. Neurosurgery 83, E98–E100. doi: 10.1093/neuros/nyy295
Ludwig, S., Floros, T., Theodoraki, M. N., Hong, C. S., Jackson, E. K., Lang, S., et al. (2017). Suppression of lymphocyte functions by plasma exosomes correlates with disease activity in patients with head and neck cancer. Clin. Cancer Res. 23, 4843–4854. doi: 10.1158/1078-0432.CCR-16-2819
Lux, A., Kahlert, C., Grutzmann, R., and Pilarsky, C. (2019). c-Met and PD-L1 on circulating exosomes as diagnostic and prognostic markers for pancreatic cancer. Int. J. Mol. Sci. 20:567. doi: 10.3390/ijms20133305
Madore, J., Vilain, R. E., Menzies, A. M., Kakavand, H., Wilmott, J. S., Hyman, J., et al. (2015). PD-L1 expression in melanoma shows marked heterogeneity within and between patients: implications for anti-PD-1/PD-L1 clinical trials. Pigment Cell Melanoma Res. 28, 245–253. doi: 10.1111/pcmr.12340
Mahoney, K. M., Freeman, G. J., and McDermott, D. F. (2015). The next immune-checkpoint inhibitors: PD-1/PD-L1 blockade in melanoma. Clin. Ther. 37, 764–782. doi: 10.1016/j.clinthera.2015.02.018
Margolis, L., and Sadovsky, Y. (2019). The biology of extracellular vesicles: the known unknowns. PLoS Biol. 17:e3000363. doi: 10.1371/journal.pbio.3000363
Mariotti, F. R., Petrini, S., Ingegnere, T., Tumino, N., Besi, F., Scordamaglia, F., et al. (2019). PD-1 in human NK cells: evidence of cytoplasmic mRNA and protein expression. Oncoimmunology 8:1557030. doi: 10.1080/2162402X.2018.1557030
Martinez-Morilla, S., McGuire, J., Gaule, P., Moore, L., Acs, B., Cougot, D., et al. (2020). Quantitative assessment of PD-L1 as an analyte in immunohistochemistry diagnostic assays using a standardized cell line tissue microarray. Lab. Invest. 100, 4–15. doi: 10.1038/s41374-019-0295-9
Matei, I., Kim, H. S., and Lyden, D. (2017). Unshielding exosomal RNA unleashes tumor growth and metastasis. Cell 170, 223–225. doi: 10.1016/j.cell.2017.06.047
Matusek, T., Wendler, F., Poles, S., Pizette, S., D’Angelo, G., Furthauer, M., et al. (2014). The ESCRT machinery regulates the secretion and long-range activity of Hedgehog. Nature 516, 99–103. doi: 10.1038/nature13847
Maybruck, B. T., Pfannenstiel, L. W., Diaz-Montero, M., and Gastman, B. R. (2017). Tumor-derived exosomes induce CD8(+) T cell suppressors. J. Immunother. Cancer 5:65. doi: 10.1186/s40425-017-0269-7
Mayoux, M., Roller, A., Pulko, V., Sammicheli, S., Chen, S., Sum, E., et al. (2020). Dendritic cells dictate responses to PD-L1 blockade cancer immunotherapy. Sci. Transl. Med. 12:eaav7431. doi: 10.1126/scitranslmed.aav7431
Milane, L., Singh, A., Mattheolabakis, G., Suresh, M., and Amiji, M. M. (2015). Exosome mediated communication within the tumor microenvironment. J. Control. Release 219, 278–294. doi: 10.1016/j.jconrel.2015.06.029
Miller, B. C., Sen, D. R., Al Abosy, R., Bi, K., Virkud, Y. V., LaFleur, M. W., et al. (2019). Subsets of exhausted CD8(+) T cells differentially mediate tumor control and respond to checkpoint blockade. Nat. Immunol. 20, 326–336. doi: 10.1038/s41590-019-0312-6
Mimura, K., Teh, J. L., Okayama, H., Shiraishi, K., Kua, L. F., Koh, V., et al. (2018). PD-L1 expression is mainly regulated by interferon gamma associated with JAK-STAT pathway in gastric cancer. Cancer Sci. 109, 43–53. doi: 10.1111/cas.13424
Momen-Heravi, F., Balaj, L., Alian, S., Mantel, P. Y., Halleck, A. E., Trachtenberg, A. J., et al. (2013). Current methods for the isolation of extracellular vesicles. Biol. Chem. 394, 1253–1262. doi: 10.1515/hsz-2013-0141
Mondini, M., Levy, A., Meziani, L., Milliat, F., and Deutsch, E. (2020). Radiotherapy-immunotherapy combinations - perspectives and challenges. Mol. Oncol. 14, 1529–1537. doi: 10.1002/1878-0261.12658
Monypenny, J., Milewicz, H., Flores-Borja, F., Weitsman, G., Cheung, A., Chowdhury, R., et al. (2018). ALIX regulates tumor-mediated immunosuppression by controlling EGFR activity and PD-L1 presentation. Cell Rep. 24, 630–641. doi: 10.1016/j.celrep.2018.06.066
Morales-Betanzos, C. A., Lee, H., Gonzalez Ericsson, P. I., Balko, J. M., Johnson, D. B., Zimmerman, L. J., et al. (2017). Quantitative Mass Spectrometry Analysis of PD-L1 Protein Expression, N-glycosylation and Expression Stoichiometry with PD-1 and PD-L2 in Human Melanoma. Mol. Cell Proteomics 16, 1705–1717. doi: 10.1074/mcp.RA117.000037
Myers, G. (2018). Immune-related adverse events of immune checkpoint inhibitors: a brief review. Curr. Oncol. 25, 342–347. doi: 10.3747/co.25.4235
Nagai, H., and Muto, M. (2018). Optimal management of immune-related adverse events resulting from treatment with immune checkpoint inhibitors: a review and update. Int. J. Clin. Oncol. 23, 410–420. doi: 10.1007/s10147-018-1259-6
Nakayama, Y., Mimura, K., Kua, L. F., Okayama, H., Min, A. K. T., Saito, K., et al. (2020). Immune suppression caused by PD-L2 expression on tumor cells in gastric cancer. Gastric Cancer. doi: 10.1007/s10120-020-01079-z [Epub ahed of print].
Nduom, E. K., Wei, J., Yaghi, N. K., Huang, N., Kong, L. Y., Gabrusiewicz, K., et al. (2016). PD-L1 expression and prognostic impact in glioblastoma. Neuro Oncol. 18, 195–205. doi: 10.1093/neuonc/nov172
Newton, R., Priyadharshini, B., and Turka, L. A. (2016). Immunometabolism of regulatory T cells. Nat. Immunol. 17, 618–625. doi: 10.1038/ni.3466
Ng, K. W., Attig, J., Young, G. R., Ottina, E., Papamichos, S. I., Kotsianidis, I., et al. (2019). Soluble PD-L1 generated by endogenous retroelement exaptation is a receptor antagonist. eLife 8:e50256. doi: 10.7554/eLife.50256
Ngwa, W., Irabor, O. C., Schoenfeld, J. D., Hesser, J., Demaria, S., and Formenti, S. C. (2018). Using immunotherapy to boost the abscopal effect. Nat. Rev. Cancer 18, 313–322. doi: 10.1038/nrc.2018.6
Nishino, M., Giobbie-Hurder, A., Gargano, M., Suda, M., Ramaiya, N. H., and Hodi, F. S. (2013). Developing a common language for tumor response to immunotherapy: immune-related response criteria using unidimensional measurements. Clin. Cancer Res. 19, 3936–3943. doi: 10.1158/1078-0432.CCR-13-0895
Okuma, Y., Hosomi, Y., Nakahara, Y., Watanabe, K., Sagawa, Y., and Homma, S. (2017). High plasma levels of soluble programmed cell death ligand 1 are prognostic for reduced survival in advanced lung cancer. Lung Cancer 104, 1–6. doi: 10.1016/j.lungcan.2016.11.023
Olmos, Y., and Carlton, J. G. (2016). The ESCRT machinery: new roles at new holes. Curr. Opin. Cell Biol. 38, 1–11. doi: 10.1016/j.ceb.2015.12.001
Orme, J. J., Jazieh, K. A., Xie, T., Harrington, S., Liu, X., Ball, M., et al. (2020). ADAM10 and ADAM17 cleave PD-L1 to mediate PD-(L)1 inhibitor resistance. Oncoimmunology 9:1744980. doi: 10.1080/2162402X.2020.1744980
Ostrowski, M., Carmo, N. B., Krumeich, S., Fanget, I., Raposo, G., Savina, A., et al. (2010). Rab27a and Rab27b control different steps of the exosome secretion pathway. Nat. Cell Biol. 12, 19–30. doi: 10.1038/ncb2000
Page, D. B., Postow, M. A., Callahan, M. K., Allison, J. P., and Wolchok, J. D. (2014). Immune modulation in cancer with antibodies. Annu. Rev. Med. 65, 185–202. doi: 10.1146/annurev-med-092012-112807
Pang, Y., Shi, J., Yang, X., Wang, C., Sun, Z., and Xiao, R. (2020). Personalized detection of circling exosomal PD-L1 based on Fe3O4@TiO2 isolation and SERS immunoassay. Biosens. Bioelectron. 148:111800. doi: 10.1016/j.bios.2019.111800
Park, J. J., Omiya, R., Matsumura, Y., Sakoda, Y., Kuramasu, A., Augustine, M. M., et al. (2010). B7-H1/CD80 interaction is required for the induction and maintenance of peripheral T-cell tolerance. Blood 116, 1291–1298. doi: 10.1182/blood-2010-01-265975
Parry, R. V., Chemnitz, J. M., Frauwirth, K. A., Lanfranco, A. R., Braunstein, I., Kobayashi, S. V., et al. (2005). CTLA-4 and PD-1 receptors inhibit T-cell activation by distinct mechanisms. Mol. Cell. Biol. 25, 9543–9553. doi: 10.1128/MCB.25.21.9543-9553.2005
Patel, S. P., and Kurzrock, R. (2015). PD-L1 expression as a predictive biomarker in cancer immunotherapy. Mol. Cancer Ther. 14, 847–856. doi: 10.1158/1535-7163.MCT-14-0983
Patsoukis, N., Brown, J., Petkova, V., Liu, F., Li, L., and Boussiotis, V. A. (2012). Selective effects of PD-1 on Akt and Ras pathways regulate molecular components of the cell cycle and inhibit T cell proliferation. Sci. Signal. 5:ra46. doi: 10.1126/scisignal.2002796
Phuyal, S., Hessvik, N. P., Skotland, T., Sandvig, K., and Llorente, A. (2014). Regulation of exosome release by glycosphingolipids and flotillins. FEBS J. 281, 2214–2227. doi: 10.1111/febs.12775
Poggio, M., Hu, T., Pai, C. C., Chu, B., Belair, C. D., Chang, A., et al. (2019). Suppression of exosomal PD-L1 induces systemic anti-tumor immunity and memory. Cell 177, 414–427.e13. doi: 10.1016/j.cell.2019.02.016
Pols, M. S., and Klumperman, J. (2009). Trafficking and function of the tetraspanin CD63. Exp. Cell Res. 315, 1584–1592. doi: 10.1016/j.yexcr.2008.09.020
Postow, M. A., Callahan, M. K., Barker, C. A., Yamada, Y., Yuan, J., Kitano, S., et al. (2012). Immunologic correlates of the abscopal effect in a patient with melanoma. N. Engl. J. Med. 366, 925–931. doi: 10.1056/NEJMoa1112824
Pulaski, B. A., and Ostrand-Rosenberg, S. (2001). Mouse 4T1 breast tumor model. Curr. Protoc. Immunol. Chapter 20:Unit 20.2. doi: 10.1002/0471142735.im2002s39
Quail, D. F., and Joyce, J. A. (2013). Microenvironmental regulation of tumor progression and metastasis. Nat. Med. 19, 1423–1437. doi: 10.1038/nm.3394
Raimondo, S., Pucci, M., Alessandro, R., and Fontana, S. (2020). Extracellular vesicles and tumor-immune escape: biological functions and clinical perspectives. Int. J. Mol. Sci. 21:2286. doi: 10.3390/ijms21072286
Ribas, A., Hamid, O., Daud, A., Hodi, F. S., Wolchok, J. D., Kefford, R., et al. (2016). Association of pembrolizumab with tumor response and survival among patients with advanced melanoma. JAMA 315, 1600–1609. doi: 10.1001/jama.2016.4059
Ribas, A., and Hu-Lieskovan, S. (2016). What does PD-L1 positive or negative mean? J. Exp. Med. 213, 2835–2840. doi: 10.1084/jem.20161462
Ricklefs, F. L., Alayo, Q., Krenzlin, H., Mahmoud, A. B., Speranza, M. C., Nakashima, H., et al. (2018). Immune evasion mediated by PD-L1 on glioblastoma-derived extracellular vesicles. Sci. Adv. 4:eaar2766. doi: 10.1126/sciadv.aar2766
Riley, J. L. (2009). PD-1 signaling in primary T cells. Immunol. Rev. 229, 114–125. doi: 10.1111/j.1600-065X.2009.00767.x
Rodriguez-Ruiz, M. E., Vanpouille-Box, C., Melero, I., Formenti, S. C., and Demaria, S. (2018). Immunological mechanisms responsible for radiation-induced abscopal effect. Trends Immunol. 39, 644–655. doi: 10.1016/j.it.2018.06.001
Rollins, M. R., and Gibbons Johnson, R. M. (2017). CD80 Expressed by CD8(+) T Cells Contributes to PD-L1-Induced Apoptosis of Activated CD8(+) T Cells. J. Immunol. Res. 2017:7659462. doi: 10.1155/2017/7659462
Romero, Y., Wise, R., and Zolkiewska, A. (2020). Proteolytic processing of PD-L1 by ADAM proteases in breast cancer cells. Cancer Immunol. Immunother. 69, 43–55. doi: 10.1007/s00262-019-02437-2
Ruffner, M. A., Kim, S. H., Bianco, N. R., Francisco, L. M., Sharpe, A. H., and Robbins, P. D. (2009). B7-1/2, but not PD-L1/2 molecules, are required on IL-10-treated tolerogenic DC and DC-derived exosomes for in vivo function. Eur. J. Immunol. 39, 3084–3090. doi: 10.1002/eji.200939407
Ruivo, C. F., Adem, B., Silva, M., and Melo, S. A. (2017). The biology of cancer exosomes: insights and new perspectives. Cancer Res. 77, 6480–6488. doi: 10.1158/0008-5472.CAN-17-0994
Sahebi, R., Langari, H., Fathinezhad, Z., Bahari Sani, Z., Avan, A., Ghayour Mobarhan, M., et al. (2020). Exosomes: new insights into cancer mechanisms. J. Cell. Biochem. 121, 7–16. doi: 10.1002/jcb.29120
Sandigursky, S., and Mor, A. (2018). Immune-related adverse events in cancer patients treated with immune checkpoint inhibitors. Curr. Rheumatol. Rep. 20:65. doi: 10.1007/s11926-018-0770-0
Schmidt, O., and Teis, D. (2012). The ESCRT machinery. Curr. Biol. 22, R116–R120. doi: 10.1016/j.cub.2012.01.028
Schoneberg, J., Lee, I. H., Iwasa, J. H., and Hurley, J. H. (2017). Reverse-topology membrane scission by the ESCRT proteins. Nat. Rev. Mol. Cell Biol. 18, 5–17. doi: 10.1038/nrm.2016.121
Seo, N., Akiyoshi, K., and Shiku, H. (2018). Exosome-mediated regulation of tumor immunology. Cancer Sci. 109, 2998–3004. doi: 10.1111/cas.13735
Sharma, P., Hu-Lieskovan, S., Wargo, J. A., and Ribas, A. (2017). Primary, adaptive, and acquired resistance to cancer immunotherapy. Cell 168, 707–723. doi: 10.1016/j.cell.2017.01.017
Sheehan, C., and D’Souza-Schorey, C. (2019). Tumor-derived extracellular vesicles: molecular parcels that enable regulation of the immune response in cancer. J. Cell Sci. 132:jcs235085. doi: 10.1242/jcs.235085
Shen, X., and Zhao, B. (2018). Efficacy of PD-1 or PD-L1 inhibitors and PD-L1 expression status in cancer: meta-analysis. BMJ 362:k3529. doi: 10.1136/bmj.k3529
Sheppard, K. A., Fitz, L. J., Lee, J. M., Benander, C., George, J. A., Wooters, J., et al. (2004). PD-1 inhibits T-cell receptor induced phosphorylation of the ZAP70/CD3zeta signalosome and downstream signaling to PKCtheta. FEBS Lett. 574, 37–41. doi: 10.1016/j.febslet.2004.07.083
Shergold, A. L., Millar, R., and Nibbs, R. J. B. (2019). Understanding and overcoming the resistance of cancer to PD-1/PD-L1 blockade. Pharmacol. Res. 145:104258. doi: 10.1016/j.phrs.2019.104258
Shigemori, T., Toiyama, Y., Okugawa, Y., Yamamoto, A., Yin, C., Narumi, A., et al. (2019). Soluble PD-L1 Expression in Circulation as a Predictive Marker for Recurrence and Prognosis in Gastric Cancer: direct Comparison of the Clinical Burden Between Tissue and Serum PD-L1 Expression. Ann. Surg. Oncol. 26, 876–883. doi: 10.1245/s10434-018-07112-x
Shukuya, T., and Carbone, D. P. (2016). Predictive markers for the efficacy of Anti-PD-1/PD-L1 antibodies in lung cancer. J. Thorac. Oncol. 11, 976–988. doi: 10.1016/j.jtho.2016.02.015
Sibaud, V. (2018). Dermatologic reactions to immune checkpoint inhibitors : skin toxicities and immunotherapy. Am. J. Clin. Dermatol. 19, 345–361. doi: 10.1007/s40257-017-0336-3
Skog, J., Wurdinger, T., van Rijn, S., Meijer, D. H., Gainche, L., Sena-Esteves, M., et al. (2008). Glioblastoma microvesicles transport RNA and proteins that promote tumour growth and provide diagnostic biomarkers. Nat. Cell Biol. 10, 1470–1476. doi: 10.1038/ncb1800
Skowyra, M. L., Schlesinger, P. H., Naismith, T. V., and Hanson, P. I. (2018). Triggered recruitment of ESCRT machinery promotes endolysosomal repair. Science 360:eaar5078. doi: 10.1126/science.aar5078
Solinas, C., Aiello, M., Rozali, E., Lambertini, M., Willard-Gallo, K., and Migliori, E. (2020). Programmed Cell Death-Ligand 2: a neglected but important target in the immune response to cancer? Transl. Oncol. 13:100811. doi: 10.1016/j.tranon.2020.100811
Steidl, C., Shah, S. P., Woolcock, B. W., Rui, L., Kawahara, M., Farinha, P., et al. (2011). MHC class II transactivator CIITA is a recurrent gene fusion partner in lymphoid cancers. Nature 471, 377–381. doi: 10.1038/nature09754
Stoorvogel, W. (2015). Resolving sorting mechanisms into exosomes. Cell Res. 25, 531–532. doi: 10.1038/cr.2015.39
Stovgaard, E. S., Dyhl-Polk, A., Roslind, A., Balslev, E., and Nielsen, D. (2019). PD-L1 expression in breast cancer: expression in subtypes and prognostic significance: a systematic review. Breast Cancer Res. Treat. 174, 571–584. doi: 10.1007/s10549-019-05130-1
Sugiura, D., Maruhashi, T., Okazaki, I. M., Shimizu, K., Maeda, T. K., Takemoto, T., et al. (2019). Restriction of PD-1 function by cis-PD-L1/CD80 interactions is required for optimal T cell responses. Science 364, 558–566. doi: 10.1126/science.aav7062
Sui, H., Ma, N., Wang, Y., Li, H., Liu, X., Su, Y., et al. (2018). Anti-PD-1/PD-L1 Therapy for Non-Small-Cell Lung Cancer: toward Personalized Medicine and Combination Strategies. J. Immunol. Res. 2018, 6984948. doi: 10.1155/2018/6984948
Sun, C., Mezzadra, R., and Schumacher, T. N. (2018). Regulation and Function of the PD-L1 Checkpoint. Immunity 48, 434–452. doi: 10.1016/j.immuni.2018.03.014
Sunshine, J. C., Nguyen, P. L., Kaunitz, G. J., Cottrell, T. R., Berry, S., Esandrio, J., et al. (2017). PD-L1 expression in melanoma: a quantitative immunohistochemical antibody comparison. Clin. Cancer Res. 23, 4938–4944. doi: 10.1158/1078-0432.CCR-16-1821
Szymanska, E., Budick-Harmelin, N., and Miaczynska, M. (2018). Endosomal “sort” of signaling control: the role of ESCRT machinery in regulation of receptor-mediated signaling pathways. Semin. Cell Dev. Biol. 74, 11–20. doi: 10.1016/j.semcdb.2017.08.012
Takeuchi, M., Doi, T., Obayashi, K., Hirai, A., Yoneda, K., Tanaka, F., et al. (2018). Soluble PD-L1 with PD-1-binding capacity exists in the plasma of patients with non-small cell lung cancer. Immunol. Lett. 196, 155–160. doi: 10.1016/j.imlet.2018.01.007
Tanegashima, T., Togashi, Y., Azuma, K., Kawahara, A., Ideguchi, K., Sugiyama, D., et al. (2019). Immune suppression by PD-L2 against spontaneous and treatment-related antitumor immunity. Clin. Cancer Res. 25, 4808–4819. doi: 10.1158/1078-0432.CCR-18-3991
Tang, H., Liang, Y., Anders, R. A., Taube, J. M., Qiu, X., Mulgaonkar, A., et al. (2018). PD-L1 on host cells is essential for PD-L1 blockade-mediated tumor regression. J. Clin. Invest. 128, 580–588. doi: 10.1172/JCI96061
Tang, S., and Kim, P. S. (2019). A high-affinity human PD-1/PD-L2 complex informs avenues for small-molecule immune checkpoint drug discovery. Proc. Natl. Acad. Sci. U.S.A. 116, 24500–24506. doi: 10.1073/pnas.1916916116
Tang, Y., Zhang, P., Wang, Y., Wang, J., Su, M., Wang, Y., et al. (2020). The biogenesis, biology, and clinical significance of exosomal PD-L1 in cancer. Front. Immunol. 11:604. doi: 10.3389/fimmu.2020.00604
Taube, J. M., Klein, A., Brahmer, J. R., Xu, H., Pan, X., Kim, J. H., et al. (2014). Association of PD-1, PD-1 ligands, and other features of the tumor immune microenvironment with response to anti-PD-1 therapy. Clin. Cancer Res. 20, 5064–5074. doi: 10.1158/1078-0432.CCR-13-3271
Teixido, C., Vilarino, N., Reyes, R., and Reguart, N. (2018). PD-L1 expression testing in non-small cell lung cancer. Ther. Adv. Med. Oncol. 10:1758835918763493. doi: 10.1177/1758835918763493
Theodoraki, M. N., Hoffmann, T. K., and Whiteside, T. L. (2018a). Separation of plasma-derived exosomes into CD3((+) and CD3((-) fractions allows for association of immune cell and tumour cell markers with disease activity in HNSCC patients. Clin. Exp. Immunol. 192, 271–283. doi: 10.1111/cei.13113
Theodoraki, M. N., Yerneni, S., Gooding, W. E., Ohr, J., Clump, D. A., Bauman, J. E., et al. (2019). Circulating exosomes measure responses to therapy in head and neck cancer patients treated with cetuximab, ipilimumab, and IMRT. Oncoimmunology 8:1593805. doi: 10.1080/2162402X.2019.1593805
Theodoraki, M. N., Yerneni, S. S., Hoffmann, T. K., Gooding, W. E., and Whiteside, T. L. (2018b). Clinical significance of PD-L1(+) exosomes in plasma of head and neck cancer patients. Clin. Cancer Res. 24, 896–905. doi: 10.1158/1078-0432.CCR-17-2664
Thery, C., Amigorena, S., Raposo, G., and Clayton, A. (2006). Isolation and characterization of exosomes from cell culture supernatants and biological fluids. Curr. Protoc. Cell Biol. Chapter 3:Unit 3.22. doi: 10.1002/0471143030.cb0322s30
Tkach, M., Kowal, J., and Thery, C. (2018). Why the need and how to approach the functional diversity of extracellular vesicles. Philos. Trans. R. Soc. Lond. B Biol. Sci. 373:20160479. doi: 10.1098/rstb.2016.0479
Tkach, M., and Thery, C. (2016). Communication by extracellular vesicles: Where we are and where we need to go. Cell 164, 1226–1232. doi: 10.1016/j.cell.2016.01.043
Trajkovic, K., Hsu, C., Chiantia, S., Rajendran, L., Wenzel, D., Wieland, F., et al. (2008). Ceramide triggers budding of exosome vesicles into multivesicular endosomes. Science 319, 1244–1247. doi: 10.1126/science.1153124
Ueki, Y., Suzuki, M., Horikawa, Y., Watanabe, H., Yamaguchi, Y., Morita, C., et al. (2020). Pembrolizumab-induced pancytopenia in a patient with squamous cell lung cancer. Thorac. Cancer. doi: 10.1111/1759-7714.13582 [Epub ahead of print].
van Niel, G., D’Angelo, G., and Raposo, G. (2018). Shedding light on the cell biology of extracellular vesicles. Nat. Rev. Mol. Cell Biol. 19, 213–228. doi: 10.1038/nrm.2017.125
Varricchi, G., Marone, G., Mercurio, V., Galdiero, M. R., Bonaduce, D., and Tocchetti, C. G. (2018). Immune checkpoint inhibitors and cardiac toxicity: an emerging issue. Curr. Med. Chem. 25, 1327–1339. doi: 10.2174/0929867324666170407125017
Wan, B., Nie, H., Liu, A., Feng, G., He, D., Xu, R., et al. (2006). Aberrant regulation of synovial T cell activation by soluble costimulatory molecules in rheumatoid arthritis. J. Immunol. 177, 8844–8850. doi: 10.4049/jimmunol.177.12.8844
Wan, Z., Gao, X., Dong, Y., Zhao, Y., Chen, X., Yang, G., et al. (2018). Exosome-mediated cell-cell communication in tumor progression. Am. J. Cancer Res. 8, 1661–1673.
Wanchoo, R., Karam, S., Uppal, N. N., Barta, V. S., Deray, G., Devoe, C., et al. (2017). Adverse renal effects of immune checkpoint inhibitors: a narrative review. Am. J. Nephrol. 45, 160–169. doi: 10.1159/000455014
Wang, Q., Liu, F., and Liu, L. (2017). Prognostic significance of PD-L1 in solid tumor: an updated meta-analysis. Medicine 96:e6369. doi: 10.1097/MD.0000000000006369
Wang, Q., Zhang, J., Tu, H., Liang, D., Chang, D. W., Ye, Y., et al. (2019). Soluble immune checkpoint-related proteins as predictors of tumor recurrence, survival, and T cell phenotypes in clear cell renal cell carcinoma patients. J. Immunother. Cancer 7:334. doi: 10.1186/s40425-019-0810-y
Wang, T., Nasser, M. I., Shen, J., Qu, S., He, Q., and Zhao, M. (2019). Functions of exosomes in the triangular relationship between the tumor, inflammation, and immunity in the tumor microenvironment. J. Immunol. Res. 2019:4197829. doi: 10.1155/2019/4197829
Wang, X., Wang, G., Wang, Z., Liu, B., Han, N., Li, J., et al. (2019). PD-1-expressing B cells suppress CD4(+) and CD8(+) T cells via PD-1/PD-L1-dependent pathway. Mol. Immunol. 109, 20–26. doi: 10.1016/j.molimm.2019.02.009
Wang, X., Yang, L., Huang, F., Zhang, Q., Liu, S., Ma, L., et al. (2017). Inflammatory cytokines IL-17 and TNF-alpha up-regulate PD-L1 expression in human prostate and colon cancer cells. Immunol. Lett. 184, 7–14. doi: 10.1016/j.imlet.2017.02.006
Weinmann, S. C., and Pisetsky, D. S. (2019). Mechanisms of immune-related adverse events during the treatment of cancer with immune checkpoint inhibitors. Rheumatology 58, vii59–vii67. doi: 10.1093/rheumatology/kez308
Williams, S. G., Mollaeian, A., Katz, J. D., and Gupta, S. (2020). Immune checkpoint inhibitor-induced inflammatory arthritis: identification and management. Expert Rev. Clin. Immunol. doi: 10.1080/1744666X.2020.1804362 [Epub ahead of print].
Wolf, Y., Anderson, A. C., and Kuchroo, V. K. (2019). TIM3 comes of age as an inhibitory receptor. Nat. Rev. Immunol. 20, 173–185. doi: 10.1038/s41577-019-0224-6
Wolkow, N., Jakobiec, F. A., Afrogheh, A. H., Pai, S. I., and Faquin, W. C. (2020). High expression of PD-L1 and PD-L2 in ophthalmic sebaceous carcinoma: the case for a clinical trial of checkpoint inhibitors. Am. J. Ophthalmol. (in press). doi: 10.1016/j.ajo.2020.07.031
Wortzel, I., Dror, S., Kenific, C. M., and Lyden, D. (2019). Exosome-mediated metastasis: communication from a distance. Dev. Cell 49, 347–360. doi: 10.1016/j.devcel.2019.04.011
Wu, Q., Jiang, L., Li, S. C., He, Q. J., Yang, B., and Cao, J. (2020). Small molecule inhibitors targeting the PD-1/PD-L1 signaling pathway. Acta Pharmacol. Sin. doi: 10.1038/s41401-020-0366-x [Epub ahead of print].
Xia, A., Zhang, Y., Xu, J., Yin, T., and Lu, X. J. (2019). T cell dysfunction in cancer immunity and immunotherapy. Front. Immunol. 10:1719. doi: 10.3389/fimmu.2019.01719
Xie, C., Ji, N., Tang, Z., Li, J., and Chen, Q. (2019). The role of extracellular vesicles from different origin in the microenvironment of head and neck cancers. Mol. Cancer 18:83. doi: 10.1186/s12943-019-0985-3
Xie, F., Xu, M., Lu, J., Mao, L., and Wang, S. (2019). The role of exosomal PD-L1 in tumor progression and immunotherapy. Mol. Cancer 18:146. doi: 10.1186/s12943-019-1074-3
Xu, G., Sun, L., Li, Y., Xie, F., Zhou, X., Yang, H., et al. (2019). The clinicopathological and prognostic value of PD-L1 expression in cholangiocarcinoma: a meta-analysis. Front. Oncol. 9:897. doi: 10.3389/fonc.2019.00897
Xu, R., Greening, D. W., Zhu, H. J., Takahashi, N., and Simpson, R. J. (2016). Extracellular vesicle isolation and characterization: toward clinical application. J. Clin. Invest. 126, 1152–1162. doi: 10.1172/JCI81129
Xu, Y., Wu, Y., Zhang, S., Ma, P., Jin, X., Wang, Z., et al. (2019). A tumor-specific super-enhancer drives immune evasion by guiding synchronous expression of PD-L1 and PD-L2. Cell Rep. 29:3435. doi: 10.1016/j.celrep.2019.10.093
Xu-Monette, Z. Y., Zhang, M., Li, J., and Young, K. H. (2017). PD-1/PD-L1 blockade: Have we found the key to unleash the antitumor immune response? Front. Immunol. 8:1597. doi: 10.3389/fimmu.2017.01597
Yaghoubi, N., Soltani, A., Ghazvini, K., Hassanian, S. M., and Hashemy, S. I. (2019). PD-1/PD-L1 blockade as a novel treatment for colorectal cancer. Biomed. Pharmacother. 110, 312–318. doi: 10.1016/j.biopha.2018.11.105
Yanez-Mo, M., Siljander, P. R., Andreu, Z., Zavec, A. B., Borras, F. E., Buzas, E. I., et al. (2015). Biological properties of extracellular vesicles and their physiological functions. J. Extracell. Vesicles 4:27066. doi: 10.3402/jev.v4.27066
Yang, F., Liao, X., Tian, Y., and Li, G. (2017). Exosome separation using microfluidic systems: size-based, immunoaffinity-based and dynamic methodologies. Biotechnol. J. 12:1600699. doi: 10.1002/biot.201600699
Yang, Y., Li, C. W., Chan, L. C., Wei, Y., Hsu, J. M., Xia, W., et al. (2018). Exosomal PD-L1 harbors active defense function to suppress T cell killing of breast cancer cells and promote tumor growth. Cell Res. 28, 862–864. doi: 10.1038/s41422-018-0060-4
Yang, Z., Shi, J., Xie, J., Wang, Y., Sun, J., Liu, T., et al. (2020). Large-scale generation of functional mRNA-encapsulating exosomes via cellular nanoporation. Nat. Biomed. Eng. 4, 69–83. doi: 10.1038/s41551-019-0485-1
Yearley, J. H., Gibson, C., Yu, N., Moon, C., Murphy, E., Juco, J., et al. (2017). PD-L2 expression in human tumors: relevance to anti-PD-1 therapy in cancer. Clin. Cancer Res. 23, 3158–3167. doi: 10.1158/1078-0432.CCR-16-1761
Yekula, A., Minciacchi, V. R., Morello, M., Shao, H., Park, Y., Zhang, X., et al. (2020). Large and small extracellular vesicles released by glioma cells in vitro and in vivo. J. Extracell. Vesicles 9:1689784. doi: 10.1080/20013078.2019.1689784
Yekula, A., Yekula, A., Muralidharan, K., Kang, K., Carter, B. S., and Balaj, L. (2019). Extracellular vesicles in glioblastoma tumor microenvironment. Front. Immunol. 10:3137. doi: 10.3389/fimmu.2019.03137
Yokosuka, T., Takamatsu, M., Kobayashi-Imanishi, W., Hashimoto-Tane, A., Azuma, M., and Saito, T. (2012). Programmed cell death 1 forms negative costimulatory microclusters that directly inhibit T cell receptor signaling by recruiting phosphatase SHP2. J. Exp. Med. 209, 1201–1217. doi: 10.1084/jem.20112741
Youngnak, P., Kozono, Y., Kozono, H., Iwai, H., Otsuki, N., Jin, H., et al. (2003). Differential binding properties of B7-H1 and B7-DC to programmed death-1. Biochem. Biophys. Res. Commun. 307, 672–677. doi: 10.1016/s0006-291x(03)01257-9
Yu, H., Boyle, T. A., Zhou, C., Rimm, D. L., and Hirsch, F. R. (2016). PD-L1 expression in lung cancer. J. Thorac. Oncol. 11, 964–975. doi: 10.1016/j.jtho.2016.04.014
Zerdes, I., Matikas, A., Bergh, J., Rassidakis, G. Z., and Foukakis, T. (2018). Genetic, transcriptional and post-translational regulation of the programmed death protein ligand 1 in cancer: biology and clinical correlations. Oncogene 37, 4639–4661. doi: 10.1038/s41388-018-0303-3
Zhang, L., and Yu, D. (2019). Exosomes in cancer development, metastasis, and immunity. Biochim. Biophys. Acta Rev. Cancer 1871, 455–468. doi: 10.1016/j.bbcan.2019.04.004
Zhang, Y., Liu, Y., Liu, H., and Tang, W. H. (2019). Exosomes: biogenesis, biologic function and clinical potential. Cell Biosci. 9:19. doi: 10.1186/s13578-019-0282-2
Zhao, Y., Lee, C. K., Lin, C. H., Gassen, R. B., Xu, X., Huang, Z., et al. (2019). PD-L1:CD80 Cis-Heterodimer Triggers the Co-stimulatory Receptor CD28 While Repressing the Inhibitory PD-1 and CTLA-4 Pathways. Immunity 51, 1059–1073.e9. doi: 10.1016/j.immuni.2019.11.003
Zhao, Z., Fan, J., Hsu, Y. S., Lyon, C. J., Ning, B., and Hu, T. Y. (2019). Extracellular vesicles as cancer liquid biopsies: from discovery, validation, to clinical application. Lab Chip 19, 1114–1140. doi: 10.1039/c8lc01123k
Zhou, J., Mahoney, K. M., Giobbie-Hurder, A., Zhao, F., Lee, S., Liao, X., et al. (2017). Soluble PD-L1 as a biomarker in malignant melanoma treated with checkpoint blockade. Cancer Immunol. Res. 5, 480–492. doi: 10.1158/2326-6066.CIR-16-0329
Zhou, K., Guo, S., Tong, S., Sun, Q., Li, F., Zhang, X., et al. (2018). Immunosuppression of human adipose-derived stem cells on t cell subsets via the reduction of NF-kappaB activation mediated by PD-L1/PD-1 and Gal-9/TIM-3 Pathways. Stem Cells Dev. 27, 1191–1202. doi: 10.1089/scd.2018.0033
Keywords: exosomal PD-L1, tumor microenvironment, immune escape, antitumor immune memory, abscopal effect, biomarker, detection method, immunotherapy
Citation: Zhou K, Guo S, Li F, Sun Q and Liang G (2020) Exosomal PD-L1: New Insights Into Tumor Immune Escape Mechanisms and Therapeutic Strategies. Front. Cell Dev. Biol. 8:569219. doi: 10.3389/fcell.2020.569219
Received: 03 June 2020; Accepted: 27 August 2020;
Published: 15 October 2020.
Edited by:
Adriana Franco Paes Leme, Brazilian Biosciences National Laboratory (LNBio), BrazilReviewed by:
Hinrich Peter Hansen, University of Cologne, GermanyMariateresa Giuliano, University of Campania Luigi Vanvitelli, Italy
Diana Noronha Nunes, A C Camargo Cancer Center, Brazil
Copyright © 2020 Zhou, Guo, Li, Sun and Liang. This is an open-access article distributed under the terms of the Creative Commons Attribution License (CC BY). The use, distribution or reproduction in other forums is permitted, provided the original author(s) and the copyright owner(s) are credited and that the original publication in this journal is cited, in accordance with accepted academic practice. No use, distribution or reproduction is permitted which does not comply with these terms.
*Correspondence: Shu Guo, c2d1b0BjbXUuZWR1LmNu