- Department of Cell Biology, Faculty of Medicine and Dentistry, University of Alberta, Edmonton, AB, Canada
Research using the fruit fly Drosophila melanogaster has traditionally focused on understanding how mutations affecting gene regulation or function affect processes linked to animal development. Accordingly, flies have become an essential foundation of modern medical research through repeated contributions to our fundamental understanding of how their homologs of human genes function. Peroxisomes are organelles that metabolize lipids and reactive oxygen species like peroxides. However, despite clear linkage of mutations in human genes affecting peroxisomes to developmental defects, for many years fly models were conspicuously absent from the study of peroxisomes. Now, the few early studies linking the Rosy eye color phenotype to peroxisomes in flies have been joined by a growing body of research establishing novel roles for peroxisomes during the development or function of specific tissues or cell types. Similarly, unique properties of cultured fly Schneider 2 cells have advanced our understanding of how peroxisomes move on the cytoskeleton. Here, we profile how those past and more recent Drosophila studies started to link specific effects of peroxisome dysfunction to organ development and highlight the utility of flies as a model for human peroxisomal diseases. We also identify key differences in the function and proliferation of fly peroxisomes compared to yeast or mammals. Finally, we discuss the future of the fly model system for peroxisome research including new techniques that should support identification of additional tissue specific regulation of and roles for peroxisomes.
Overview
Peroxisomes are organelles found in almost all eukaryotic cells. Some functions of peroxisomes, especially in terms of oxygen and lipid metabolism can overlap those of mitochondria. However, the structure of peroxisomes and mitochondria are quite different with peroxisomes being composed of a single bilayer membrane surrounding a dense matrix composed of enzymes. The volume or number of peroxisomes within a single cell changes dynamically depending on surrounding conditions. There are several competing models for the mechanisms mediating peroxisome proliferation in mammalian cells, but all propose templated assembly either by fission and growth of the existing pool and/or de novo assembly from small membrane vesicles and proteins imported from the cytoplasm (reviewed in Mast et al., 2020).
Peroxisome composition and activity varies between organisms and even between cell types in multicellular animals. Generally peroxisomes are responsible for catabolism of branched or VLCFAs, purine catabolism, synthesis of specialized fatty acids like plasmalogens, ether-lipids and bile acids, and regulation of hydrogen peroxide and other ROS (reviewed in Fujiki et al., 2020). Yeast can survive without peroxisomes when grown in most conditions, but are dependent on their activity for β-oxidation (reviewed in Yuan et al., 2016). In mammals, β-oxidation occurs largely in the mitochondria. Peroxisomes are normally required for catabolism of branched and very-long chain fatty acids (≥C22) but can metabolize other fatty acids when mitochondria are compromised (Violante et al., 2019). Peroxisomes are functionally, and in some cases can be physically, linked to other organelles including the endoplasmic reticulum (ER), mitochondria, lipid droplets and lysosomes (reviewed in Schrader et al., 2020). Mutation in human genes encoding peroxisome assembly factors (Peroxins), or in enzymes within peroxisomes, leads to a variety of disorders ranging from mild to very severe forms causing death in infants (reviewed in Schiller et al., 2020).
Use of the fruit fly Drosophila melanogaster as a laboratory animal originated at the turn of the 20th century (reviewed in Tolwinski, 2017). Similar to mammals, Drosophila has a diploid genome with X (female) and Y (male) sex chromosomes. However, relative to mammals, the fly genome is less complex with approximately 15,500 genes on four chromosomes (Morgan and Bridges, 1916; Adams et al., 2000; Roy et al., 2010). The continued success of Drosophila for fundamental genetic and cell physiological research has been supported by the ongoing development of unique tools and collaborative efforts that have generated thousands of unique strains and reagents for genetics, cell and molecular biology studies (reviewed in St Johnston, 2002; Hales et al., 2015). This genetic tractability and a large innovative community of fly researchers remain key elements to its enduring laboratory use. Forward genetic screens driven by traditional mutagenesis techniques like X-rays, chemical mutagens and transposable element insertion or now CRISPR-Cas9, represent a well-used approach to identifying genetic pathways related to cell or developmental functions in flies (reviewed in Gratz et al., 2015). These screens are often part of multi-center, large-scale collaborative projects that generate a wealth of strains with unique mutations, most of which are made widely available from central stock centers (Bellen et al., 2004; Venken et al., 2011). One technique of particular note, used for many of the studies of fly peroxisomes, has been the generation of a large library of transgenic flies with selective time/tissue specific expression of the S. cerevisiae Gal4 gene. These well characterized lines are used to drive transgenes containing an UAS. This binary expression regulation system permits facile alteration of gene expression by double-stranded (dsRNA) knockdown, cell ablation, ectopic gene expression, or expression of reporter genes within specific cell lineages within developing organs, especially the CNS (Brand and Perrimon, 1993; Pfeiffer et al., 2008).
Finally, although Drosophila is traditionally thought of as a laboratory animal used for genetic studies, there are also cultured cell lines available to complement whole animal studies. Most Drosophila cell lines are isolated by protease digestion or mechanical separation of tissues and immortalized spontaneously from primary cultures (Schneider, 1972; Ui et al., 1987). The most commonly used are Schneider 2 (S2) cells. These are transformable with standard methods, and are used to produce recombinant proteins and for in vivo imaging. S2 cells are particularly amenable to transient dsRNA-based RNA interference (RNAi; reviewed in Baum and Cherbas, 2008) and have been used extensively to identify genes involved in fundamental cell biological events including intracellular peroxisome movement (Kural et al., 2005).
Drosophila Development
Early fly embryo development is largely dependent on mRNA and proteins supplied maternally, so the early stages of development can often proceed for some time even if the embryos are homozygous for mutations in essential genes (reviewed in Lasko, 2020). Under optimal conditions, embryogenesis completes 20–24 h after egg laying and a larva emerges. Drosophila larvae pass through three juvenile developmental stages, or “instars.” The first and second instars last 24 h each and the third instar lasts 48 h (Demerec, 1950). At the end of the third instar, the larva forms a pupa. During this stage much of the larval body is broken down and much of the adult (imago) develops de novo (Hales et al., 2015). Metamorphosis into the imago usually takes 84 h (Demerec, 1950; Hartenstein, 1993). Many adult tissues are pre-figured in the larval stage as collections of cells called imaginal disks. During metamorphosis, the imaginal disks form adult structures including antennae, limbs, eyes, genitals, and wings (reviewed in Beira and Paro, 2016). Most embryonic and larval organs are lost during metamorphosis but some, notably the CNS, are instead remodeled and are largely preserved into adulthood (Hartenstein, 1993). Adult flies have a lifespan of 45–60 days depending on genetic background and culture conditions (Demerec, 1950).
Drosophila as a Model for Human Genetic Diseases
Drosophila has a simple body plan and organs analogous to most of those affected in human patients with peroxisomal disorders making them well-suited to studying the developmental effects of gene mutations that alter peroxisome function. Embryonic studies in particular are facilitated by the external development of the fly embryo, making it amenable to advanced imaging analyses (reviewed in Pantazis and Supatto, 2014). Many of the pathways that regulate development of analogous tissues in humans were characterized initially in Drosophila (reviewed in Wangler et al., 2015). Conservation of the pathways regulating early organ development between Drosophila and humans is sufficient in the CNS and PNS, musculature, kidney and liver analogs and lipid storage (fat body) for easy comparison of effects (reviewed in Yamamoto et al., 2014).
Studying CNS/PNS Development and Behavior in Flies
Peroxisome diseases have a particularly strong effect on CNS development (reviewed in Faust et al., 2005). Drosophila has historically been valuable to model human diseases affecting the CNS. Some good examples include models of Alzheimer’s, Parkinson’s, Fragile X-syndrome, autism spectrum disorder and ALS (Phillips et al., 1995; Torroja et al., 1999; Feany and Bender, 2000; Wan et al., 2000; Whitworth et al., 2005; Park et al., 2006; Feiguin et al., 2009; Stessman et al., 2016; Ordonez et al., 2018). Flies have simple brain morphology and can exhibit learning and memory behaviors. As such, they can be used to study cognitive disorders (reviewed in Androschuk et al., 2015). The relative simplicity of fly behaviors, for example coordinated movement, larval food foraging and adult climbing or “negative geotaxis” or “bang assay” permit quantification because defects are easy to see (Le Bourg and Lints, 1992; Günther et al., 2016). Morphological and molecular characterization of CNS/PNS patterning, locomotor activity in embryos and larvae as well as behavioral assays like the climbing assay have been used to examine the effects of aberrant peroxisome function on CNS/PNS development in Drosophila (Faust et al., 2014; Bulow et al., 2018; Di Cara et al., 2018, 2019).
The Fly Analogs of Kidney and Liver and Their Role in Lipid Metabolism
Peroxisome disorders in humans are associated with several deleterious effects on the organs responsible for lipid metabolism such as the kidney or liver (reviewed in Wanders, 2013). Drosophila can be used to model the effect of gene mutations on development and function of the human kidney and liver (reviewed in Bharucha, 2009). The Drosophila Malpighian tubules are functionally analogous to the renal tubules of the vertebrate kidney, clearing toxins, and regulating ion homeostasis in the hemolymph (reviewed in Gautam et al., 2017). Nephrocyte cells, surrounding the heart (pericardial) and esophagus (garland), combined with the Malpighian tubule perform the size-based filtration mechanism of podocyte cells found in the glomerulus of the mammalian kidney (reviewed in Weavers et al., 2009). One good example of modeling kidney disease in flies is xanthinuria type one caused by loss of enzyme xanthine dehydrogenase (XDH). This results in yellowish inclusions indicating xanthine stone, xanthine and hypoxanthine accumulation, and low levels of metabolites like uric acid (Dent and Philpot, 1954; Hadorn and Schwinck, 1956; Hilliker et al., 1992). In humans, XDH has been proposed to localize to peroxisomes and the cytosol, but in Drosophila it is exclusively peroxisomal (Angermüller et al., 1987; Beard and Holtzman, 1987; Ichikawa et al., 1992). Flies have also been used previously to model the effects of dysfunction of a single peroxisome enzyme. Urate oxidase (Uro), an enzyme catalyzing the generation of uric acid following XDH activity, is present in peroxisomes in Drosophila and mammals. However, Uro is inactive in primates (Wu et al., 1989). Knockdown of the Uro gene in Malpighian tubules recapitulates uric acid nephrolithiasis, reduced longevity, and resulted in purine sensitivity (Lang et al., 2019).
Modeling the effects of specific gene mutations on liver function is somewhat more complicated in flies. While Drosophila fat body was long considered functionally equivalent to the liver, oenocytes are now considered by most to be the functional equivalent of hepatocytes based on similarities in lipid metabolism (reviewed in Makki et al., 2014). In insect models of mammalian liver function the contribution of both the fat body and oenocytes must be taken into account. Oenocyte-specific mutations and knockdown, as well as dietary approaches, have been used to successfully model non-alcoholic fatty liver disease (reviewed in Ugur et al., 2016). The lipid mobilization ability of the Drosophila fat body has been used to model human metabolic diseases associated with abnormal lipid storage, such as obesity linked to the lipases and perilipins caused by loss of brummer (bmm) and Lipid storage droplet-1 (Lsd-1), or Lipid storage droplet-2 (Lsd-2) overexpression, respectively; liver steatosis, linked to mutation in bmm overexpression in fat body and non-alcoholic fatty liver disease, in Drosophila Hepatic nuclear factor 4 (Hnf4)-null flies via genetic or dietary methods (Grönke et al., 2003; Gutierrez et al., 2007; Beller et al., 2010; Ugur et al., 2016). Mutations in peroxisome-linked genes have significant effects on the development or function of Malpighian tubules, fat body and oenocytes (Beard and Holtzman, 1987; Faust et al., 2014; Bulow et al., 2018; Huang et al., 2019).
Rosy Eyes and Other Early Studies of Mutations Affecting Drosophila Peroxisomes
The earliest examples of peroxisome studies in Drosophila were electron micrographs observing the localization of the peroxisomal enzymes XDH, encoded by rosy (ry), and D-amino acid oxidase (DAO; Beard and Holtzman, 1987; St Jules et al., 1989, 1990). Beard and Holtzman (1987) were the first to show that a Drosophila peroxisomal enzyme had tissue-specific differences in expression. Using 3,3′-diaminobenzidine-based detection of catalase suitable for TEM imaging they determined that peroxisomes were abundant in wild-type Drosophila Malpighian tubules and gut. EM-based visual examination of the peroxisomes in adult Drosophila heads determined peroxisome abundance varied with tissue type, suggesting the factors that govern their biogenesis and function might be tissue specific (St Jules et al., 1990). Flies homozygous for ry loss-of-function alleles lacked detectable XDH activity and had reduced catalase activity (Beard and Holtzman, 1987). Drosophila XDH activity requires functional peroxisomes as the Rosy eye phenotype also occurs when Peroxin (Pex) gene function is inhibited in the developing eye (Figure 1) (Nakayama et al., 2011). Notably, many of these early papers directly commented on the suitability of Drosophila for the study of heritable peroxisome disorders, and proposed a link between peroxisomes and other organelles based on observations of increased peroxisomal abundance in cells of the fat body (St Jules et al., 1990). Despite this endorsement, flies were not widely utilized as a model system for studying peroxisomes for another 20 years.
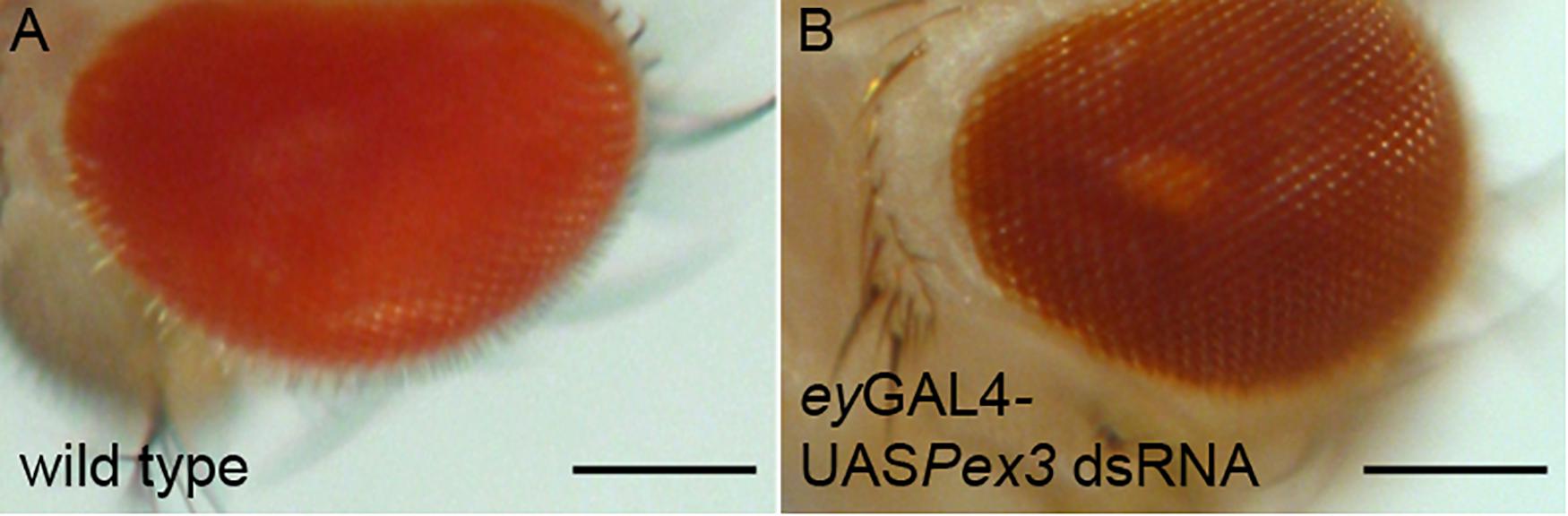
Figure 1. Knockdown of Pex3 in the Drosophila eye phenocopies the Rosy phenotype. (A) Wild type Drosophila eye pigment is a bright red-orange color. (B) Reduction of Pex3 activity specifically in the eye by a combination of eyeless(ey) GAL4 and a UAS inducible transgene expressing a dsRNA targeting Pex3 mRNA causes the characteristic dark red pigmentation associated with ry mutations. Bar = 300 μm.
Identifying and Characterizing Drosophila Pex Proteins
Pex proteins play key roles in peroxisome proliferation (Figure 2) including: forming the vesicles that supply new peroxisome membrane; inserting proteins into the peroxisome membrane including the transmembrane pores through which enzymes are recruited into the peroxisome matrix; recycling of Pex proteins that direct enzymes into peroxisomes and fission of existing peroxisomes (reviewed in Mast et al., 2020). The source of vesicles that supply membrane to new/growing peroxisomes in yeast cells is the ER (Hoepfner et al., 2005). In mammalian cells the source of vesicles supplying membrane to proliferating peroxisomes is controversial, with ER and/or mitochondria origin proposed (Rucktaschel et al., 2010; Kim, 2017; Sugiura et al., 2017). In mammalian cells, Pex13 and Pex14 are inserted post-translationally into the membrane by the combined action of Pex3 and Pex19 to form the “importomer” complex, which, combined with the activity of Pex2, Pex10, and Pex12 provides the pore through which enzymes are imported into the matrix. Cargos are recognized and carried through the importomer by Pex5, which is then recycled back to the cytoplasm by the activity of Pex1/Pex6 (reviewed in Fujiki et al., 2020). Most enzymes that are recognized by Pex5 and directed to the importomer have a variant of the PTS1 (serine–lysine–leucine, SKL) at their C-terminal end. Yeast and mammalian cells have a second peroxisome-targeting signal, PTS2 is present on some peroxisomal enzymes (e.g., thiolase) which is recognized by PEX7 which binds a variant of PEX5 (Glover et al., 1994; Subramani, 1998).
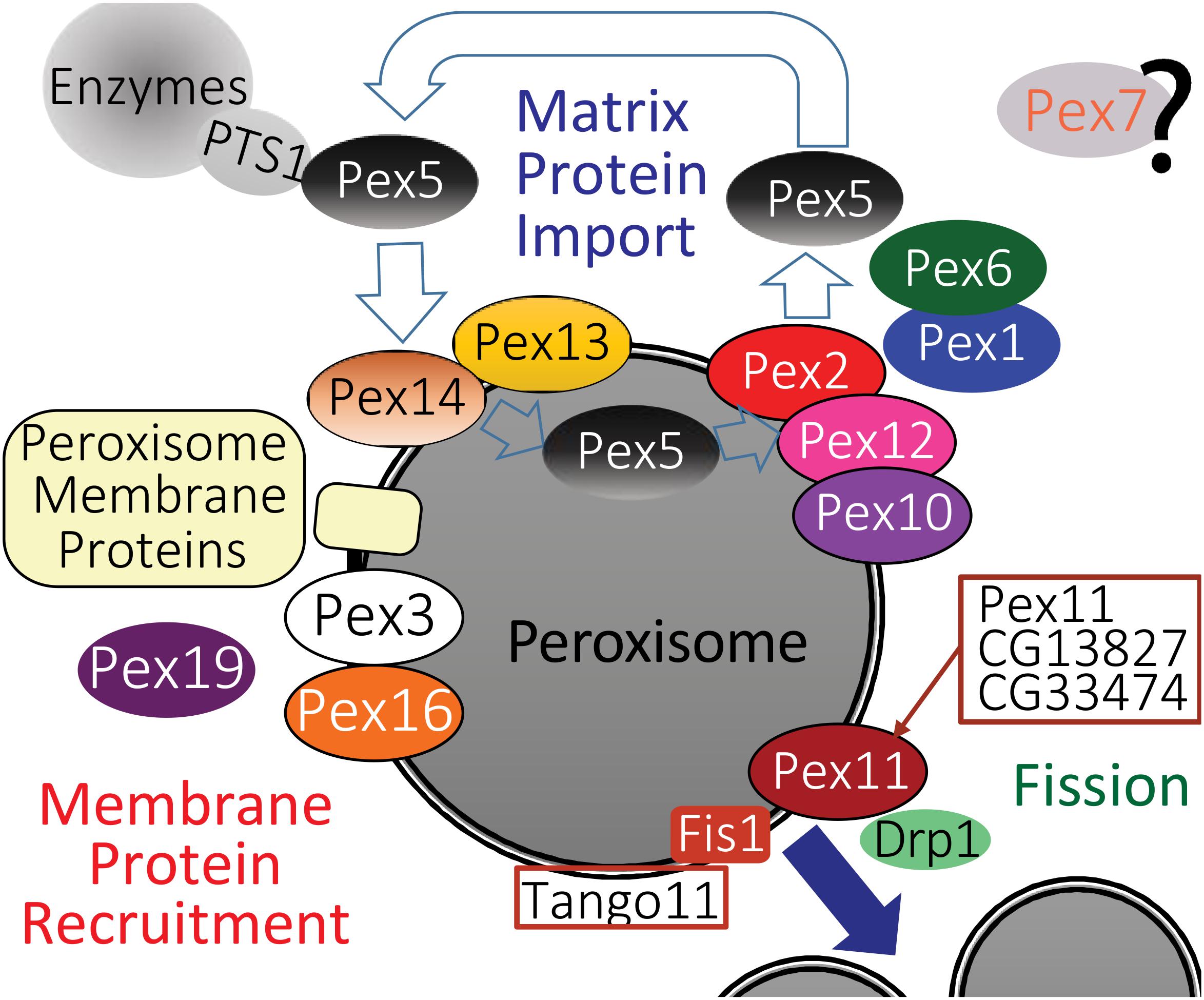
Figure 2. A model for the likely role of the Drosophila homologs of peroxisome biogenesis factors, based on a recent one for mammalian peroxisome proteins by Fujiki et al. (2020). Homologs of Pex proteins (Table 1) required for recruitment and insertion of peroxisome membrane proteins (Table 2), as well as Pex3, Pex16, and Pex19 are all conserved in Drosophila. The peroxisome fission machinery also appears conserved as there are three Drosophila Pex11 proteins (Pex11, CG13827, and CG3374). Fis1 is the homolog of Fission, mitochondrial 1 (FIS1), Tango11 is homologous to Mitochondrial fission factor (MFF) and Drp1 is the homolog of Dynamin 1 like (DNM1L), all of which are required for peroxisome fission in mammals. Drosophila peroxisomal matrix protein import in flies appears dependent on PTS1, mediated by Pex5 binding to PTS1 (Table 3), although some matrix proteins do not have a PTS1 motif (Table 4). The docking complex proteins Pex13 and Pex14 are conserved in Drosophila, as is the RING-E3-ligase complex of Pex2, Pex10, and Pex12. It is therefore likely that Pex5-bound cargos are imported, then Pex5 is recycled to the cytosol by Pex1 and Pex6 in a manner similar to mammals. A Pex7 homolog exists in Drosophila, but its role in peroxisome function is unclear.
The release of a largely complete Drosophila genome sequence in the early 2000s facilitated sequence-similarly based identification of genes and proteins homologous to those other organisms (Adams et al., 2000). This led to several publications identifying potential homologs of human or yeast PEX and other peroxisome-related proteins by predicted amino acid sequence homology (Chen et al., 2010; Mast et al., 2011; Faust et al., 2012). The fly genome was found to have several conserved Pex genes encoding proteins highly similar to their human or yeast counterparts. These included Pex1, Pex2, Pex3, Pex5, Pex6, Pex7, Pex10, Pex11, Pex12, Pex13, Pex14, Pex16 and Pex19 (Table 1) (Chen et al., 2010; Mast et al., 2011).
Confirmation of functional conservation of the import role of the predicted Drosophila Pex genes came first via RNAi-based knockdown screen in S2 cells stably expressing a GFP-peroxisomal targeting signal 1 (PTS1) fusion protein reporter that would be imported into peroxisomes with a functional importomer complex resulting in a punctate cytoplasmic GFP signal (Kural et al., 2005; Mast et al., 2011). The phenotypes induced by RNAi treatment targeting each putative Pex gene fell broadly into two categories. The first was cytosolic mislocalization of a GFP-PTS1 fusion protein (Pex1, Pex2, Pex5, Pex6, Pex12, Pex13, Pex14, Pex16, Pex19), identifying defects in protein import. The second category was aberrant peroxisome morphology/number (Pex3, Pex11), implying defects in proliferation. No effect on GFP-PTS1 transport into peroxisomes was observed for dsRNA knockdown of the gene homologous to Pex7 (Mast et al., 2011). Drosophila genes with weak similarity to yeast Pex20p or Pex23p were also identified, but RNAi treatment targeting these two genes did not cause a defective peroxisome import phenotype (Mast et al., 2011).
Identification of Other Peroxisome Factors
Identification of the Drosophila Pex homologs was followed by a homology based prediction of other peroxisome-linked genes such as those encoding peroxisomal membrane proteins, fission factors and matrix enzymes. Faust et al. (2012) identified multiple potential orthologs for many of the peroxisome proteins, based on the presence of a PTS1 domain or homology to known peroxisome proteins in yeast or mammals. Baron et al. (2016) systematically tested the peroxisome localization of each of these predicted peroxisomal proteins encoded by these, confirming the subset that was targeted to peroxisomes, and likely represented bona-fide peroxisome factors (Tables 2–4; reviewed in Anderson-Baron and Simmonds, 2018). Notably, in S2 cells, Pex3 and Pex19 were localized to peroxisomes at a level less than was expected, but functional tests suggested this was likely due to the epitope tags used for visualization (Baron et al., 2016). Functional conservation has also been demonstrated experimentally for Pex3. When expressed in S2 cells yeast Pex3 was functional, and that the N-terminal domain of Pex3 directed sorting of other Pex proteins and peroxisome membrane proteins to ER sub-domains that likely represent a source of vesicles that are incorporated into peroxisomes (Fakieh et al., 2013).
Confirmation of conserved protein–protein interactions between fly Pex proteins has largely come from large-scale whole-proteome projects that were not looking at Pex proteins specifically (Tables 1, 3). One good example of these type of communal and open-science nature of the Drosophila community is the FlyBi project1, a large-scale effort to map all potential protein interactions in an unbiased yeast 2-hybrid screen with the goal of a whole proteome binary interaction network for Drosophila. Pex3 and Pex19 were shown to interact by FlyBi, suggesting they act to target peroxisome membrane proteins to the membrane in a manner similar to mammalian cells (Figure 2). Similarly, Pex5 and Pex14 were identified as interacting as part of a large scale mass spectrometry screen (Guruharsha et al., 2011), suggesting they conserve their respective functions as a cargo binding protein (Pex5) and import channel (Pex14) in Drosophila cells (Figure 2).
Drosophila Has PEX7 but Not PTS2-Mediated Peroxisome Import
A major difference in peroxisome function between Drosophila and yeast or mammals is the absence of PTS2-mediated import. The majority of peroxisome matrix enzymes in Drosophila, including homologs of those with canonical PTS2-bearing cargo proteins in yeast or mammals (i.e., thiolase, SCPx), have a C-terminal PTS1 motif or conserved equivalent in flies (Table 3) (Faust et al., 2012). Analysis of the Drosophila proteome failed to detect the N-terminal PTS2 motif in any likely peroxisome trafficked proteins, and fluorescent microscopy data showed a PTS2-mCherry construct was unable to localize to peroxisomes in S2 cells (Faust et al., 2012). Supporting this, a reporter chimera of the putative PTS2-dependent alkylglycerone phosphate synthase homolog, encoded by CG10253, co-localized with the peroxisome marker PMP34-Cerulean only when the reporter was at the N-terminus, suggesting CG10253 encoded a peroxisomal matrix protein with a C-terminal PTS (Faust et al., 2012).
Other species like C. elegans have also lost the PTS2 pathway but also lack a Pex7 homolog (Motley et al., 2000). Although PTS2 mediated protein targeting cannot be identified in Drosophila, the presence of a Pex7 homolog of raises questions as to its role in terms of peroxisomes. Drosophila Pex7 is a functional homolog of mammalian PEX7 as it can substitute for human PEX7 to rescue PTS2-mediated peroxisome protein import in human PEX7 mutant cells (Di Cara et al., 2019). A Pex7 reporter fusion partially localized to peroxisomes (Baron et al., 2016). Pex7 was shown to be required for the ROS burst that precedes the Drosophila immune response (Di Cara et al., 2017). In terms of development, Pex7 mutants show reduced viability and CNS defects. However, these defects are more mild than those linked to mutations in Pex5 (Di Cara et al., 2019).
A clue for Pex7 activity in Drosophila may come from its pattern of expression. Compared to the relatively ubiquitous expression of Drosophila Pex genes in the early embryo Pex7 is expressed at relatively high levels in only a small subset of cells thought to be of neural lineage (Pridie and Simmonds, 2020). This suggests a more specialized, potentially cell-lineage specific role. This role appears to be linked with peroxisome activity as phenotypes associated with targeted Pex7 RNAi in the gut were rescued by ubiquitous Catalase over-expression. Also, loss of Pex7 affects the overall redox state at the cellular and whole-organism levels (Di Cara et al., 2018). This suggests that Pex7 plays a role in peroxisomal ROS management, affecting enzymes of the Catalase enzymatic pathway, e.g., SOD. The likely scenario is that Drosophila Pex7 likely retains a peroxisome-related function unrelated to PTS2-mediated import. What this role could be remains unknown, but the unique situation in Drosophila where Pex7 is present but PTS2 import is not provides a unique platform to determine what it could be. It is particularly intriguing to consider that this putative Pex7 function outside of PTS2 cargo recognition may also be active in other organisms.
Regulation of Peroxisome Volume or Number in Drosophila
Although localization of homologous Drosophila proteins to peroxisomes has been largely confirmed experimentally (Tables 1–4), conservation of their functional roles for the most part has not. This includes the mechanisms regulating peroxisome volume and number. In steady state conditions, homeostatic feedback mechanisms are thought to keep the relative volume and number of peroxisomes constant. However, peroxisome volume and number responds to changes to the cellular environment. This response can be increased volume or number, or reduction in peroxisome abundance via macroautophagy (reviewed in Chang et al., 1999; Germain and Kim, 2020). This mechanisms underlying pexophagy have been shown most clearly in yeast (Motley and Hettema, 2007). Peroxisome proliferation can be correlated to changes in expression of Pex genes as well as other peroxisome-linked genes. The volume and number of fly peroxisomes responds to changes in peroxisome-linked gene expression. S2 cells cultured under standard conditions have between 60 and 80 peroxisomes with an average volume of 173 nm3 (Baron et al., 2016). Altering the levels of Drosophila peroxisome factors, including Pex proteins, membrane proteins or peroxisomal enzymes often had significant effects on volume or number (Baron et al., 2016).
Transcriptional Regulation of Pex and Peroxisome Related Genes
Regulation of the transcription of peroxisome-linked genes in Drosophila is currently not well characterized. Peroxisomes can be induced to proliferate by excess fatty acids and their analogs (reviewed in Yan et al., 2005). In mammalian cells, this occurs in part by inducing increased expression of genes encoding peroxisome proliferation factors regulated by conserved, lipid-sensing members of a nuclear hormone receptor superfamily called peroxisome-proliferator activated receptors (PPARs; reviewed in Bocos et al., 1995). Upon ligand binding, the three PPAR variants (α, β/δ, γ) form heterodimers with the retinoid X-receptor (RXR) to express enzymes involved in β-oxidation and other reactions to regulate lipid metabolism (reviewed in Kliewer et al., 2001). In mice, both PPARα and PPARγ regulate expression of Pex genes, however, the link between PPARs and peroxisome proliferation in humans is less clear (Dubois et al., 2017; Mirza et al., 2019).
PPAR homologs do not seem to be present in the Drosophila genome, but other nuclear hormone receptors likely act in a similar fashion. Drosophila Hnf4 generally regulates β-oxidation in the larva (Palanker et al., 2009). PPARα is highly expressed in liver and kidney and Hnf4 is likewise highly expressed in the analogous fly tissues, the oenocytes and Malpighian tubules, respectively (Palanker et al., 2009). Both PPARα and Hnf4 are activated by LCFAs to induce expression of fatty acid β-oxidation enzymes (Göttlicher et al., 1992; Braissant et al., 1996; Palanker et al., 2009). The fly analog of PPARγ is less clear, but candidates may be inferred from phenotypes associated with their roles in the CNS. PPARγ is implicated in mitigating the neurodegenerative phenotype in ALS through its anti-inflammatory role (Li et al., 2000; Kiaei, 2008). Mice ALS models carrying mutations in SOD1, and Drosophila ALS models overexpressing TDP-43, both displayed ALS-like neurodegeneration that was rescued by the PPARγ agonist pioglitazone (Kiaei, 2008; Joardar et al., 2015). The phylogenetic relationship with human nuclear receptors predicts E75 and E78 of Drosophila as one of the closest potential PPARγ homologs (King-Jones and Thummel, 2005). Accordingly, in Drosophila ALS models, TDP-43 induction in glial and motor neurons, but not in musculature, was shown to rescue locomotor deficits upon agonist introduction when both E75 and E78 were present, suggesting functional conservation (Joardar et al., 2015). Notably, spargel (srl) the Drosophila ortholog of PPARγ co-activator 1α (PGC1-α) is upregulated in Pex19 mutant cells (Bulow et al., 2018), suggesting that an analogous pathway plays a role in feedback regulation of peroxisome abundance.
Effect of Peroxisome Enzyme Abundance on Peroxisomes
The effect of peroxisomal enzyme abundance on peroxisome volume or number is variable. Elevated levels of phosphomevalonate kinase (Pmvk), mitochondrial ubiquitin ligase activator of NF-κB (Mul1), carnitine O−octanoyltransferase (Crot) and α−methylacyl−CoA racemase (Amacr) lead to an average four-fold increase of normal peroxisome volumes. Conversely, Dhsr4 and ScpX lead to decreased peroxisome volume when overexpressed in S2 cells (Baron et al., 2016). The molecular mechanism underlying this decreased volume is likely indirect via feedback caused by altered metabolism of certain peroxisomal lipid species. One particular class of enzyme that responds to changes in lipid metabolism is fatty acyl-CoA reductase (FAR) family proteins. These participate in an early rate-limiting step of plasmalogen synthesis and the cellular levels of FAR proteins are directly dependent on plasmalogen level (Honsho et al., 2013). FAR1 upregulation is observed in mice when plasmalogen synthesis was genetically inhibited, which was not associated with change in peroxisome abundance (Kimura et al., 2018; Merkling et al., 2019). Drosophila has 17 potential FAR orthologs of which only one has been examined experimentally. The one that has, Sgroppino, localizes to a subset of peroxisomes (Merkling et al., 2019). Accordingly, Sgroppino was not shown to affect Drosophila peroxisome number. However, mutations in genes encoding other peroxisome enzymes do have a strong effect on peroxisome proliferation in mammalian cells. For example, fibroblasts from patients with mutations in enzymes involved in β-oxidation pathway, acyl-CoA oxidase and multifunctional enzyme type 2/17-beta-hydroxysteroid dehydrogenase 4 (MFE-2/HSD17B4) show a discernible reduction in peroxisome abundance (Chang et al., 1999). Elevated expression of Drosophila Mfe2 (a.k.a. DBP) did not seem to affect peroxisome volume but did reduce peroxisome abundance (Baron et al., 2016). Although, the ectopic overexpression of gain-of-function mutant form of human ACOX1 in flies is reported to have no discernible effect on peroxisome numbers relative to overexpression of its wild type counterpart (Chung et al., 2020). Thus, the relationship between the relative abundance of various peroxisome enzymes and the volume or number of peroxisomes in Drosophila remains to be established.
Regulation of Peroxisome Fission
The roles of factors regulating peroxisome fission (Figure 2) have not yet been systematically studied in flies. The primary effectors of peroxisome fission are Drp1, Fis1, Mff, and PEX11A/B (Schrader et al., 2016). Drosophila has homologs of all of these proteins (Figure 2). Increased levels of the Pex11 A/B homolog in S2 cells (Pex11) caused a two-fold increase in number and a corresponding reduction in volume (Baron et al., 2016). There are two predicted PEX11C homologs in Drosophila, (CG13827 and CG33474, Table 1). Other homologs of the peroxisome fission apparatus were identified independently as part of forward genetic screens for factors affecting mitochondrial proliferation. RNAi screens in S2 cells for regulators of mitochondrial fission identified Tango11 as the homolog of mitochondrial fission factor (MFF, Figure 2) (Gandre-Babbe and van der Bliek, 2008). In human cells, the knockdown of MFF resulted in changes in peroxisome morphology similar to those observed for knockdown of dynamin-related protein (DRP, encoded by DNM1L) and hindered interaction of mitochondrial fission 1 protein (FIS1) with PEX11C (Figure 2) (Gandre-Babbe and van der Bliek, 2008; Koch and Brocard, 2012). Characterization of Drp1 came specifically from employing flies to model human genetic disorders. A study using Drosophila was conducted to model the cellular impact of patients with infantile encephalopathy caused by DNM1L mutation rescued larval lethal Drp1 mutants by expressing the human DNM1L homolog via transgenic insertion (Chao et al., 2016). In transgenic larvae expressing a dominant-negative DNM1L variant, peroxisomes in salivary glands were enlarged in volume and reduced in number (Koch et al., 2003; Chao et al., 2016; Assia Batzir et al., 2019). Overexpression of Drosophila Fis1 causes an increase in peroxisome number (Baron et al., 2016) but has not been otherwise characterized in terms of a role in peroxisome proliferation. However, Fis1 has been shown to play an analogous role in mitochondrial proliferation (Liu et al., 2012). The relationship between Pex11C and Fis1 also appears to be somewhat different in flies. In yeast, Pex11C is a positive regulator of peroxisomal fission (Chang et al., 2015), whereas overexpression of Drosophila PEX11C homolog CG13827 led to enlarged peroxisomes (Baron et al., 2016). Thus, the potential roles of Pex11 homologs in flies in terms of peroxisome fission (Figure 2) needs further exploration.
Pexophagy
In each cell the biogenesis of peroxisomes is balanced by removal of old/excess peroxisomes. In mammalian cells, the population of peroxisomes is turned over approximately every 2 days (Huybrechts et al., 2009). In yeast or mammalian cells, defective or excess peroxisomes are removed by autophagy (pexophagy, reviewed in Germain and Kim, 2020). Some aspects of this process look to be conserved in Drosophila. Recently, HSPA9 (GRP75, Heat Shock Protein Family A Hsp70 Member 9) was identified as a suppressor of pexophagy in mammalian cells (Jo et al., 2020). The role is conserved in Drosophila as Gal4-UAS mediated RNAi of Hsc70-5, encoding the fly HSPA9 homolog also induced a reduction in the number of peroxisomes in third instar myocytes (Jo et al., 2020). Other aspects of pexophagy, including the effect of loss of the autophagy related (ATG) genes and their effect on fly peroxisomes has yet to be shown. Similarly, the role of Drosophila Pex proteins such as Pex2, Pex3, Pex11 or Pex14 or ubiquitination which have been shown to have roles in targeting peroxisomes for degradation in yeast or mammalian cells has not yet been examined directly.
Other Pathways Regulating Peroxisome Volume or Number
Finally, the traditional fly forward genetic screen techniques are also uncovering other potential peroxisome regulatory factors in addition to those homologous to known regulatory factors in other species. A recent small-scale forward genetic screen in whole animals using number, volume and morphology of peroxisomes (marked by GFP-PTS1) as a readout (Graves et al., 2020) looked at only at the X-chromosome (∼15% of the fly genome). This screen identified a number of genes not previously implicated in peroxisome proliferation. Several genes encoding transcription factors not previously linked to peroxisomes such as: fs(1)h, CG17829, mxc or Smox were identified (Graves et al., 2020). These potential candidates may be acting analogously to PPARs or more likely represent independent pathways of peroxisome regulation. Of particular note, this screen only tested a limited number of genes on one chromosome and did not identify several known peroxisome regulators on the X such as Pex5, suggesting there are likely many additional novel peroxisome-linked genes to be identified using these methods.
While there are clearly some key differences in terms of regulation of proliferation and assembly factors, i.e., lack of a conserved PTS2 import pathway and no direct PPAR homologs, what is equally clear is that most peroxisome-linked genes and function are sufficiently conserved between Drosophila and mammals. There is similar conservation in mitochondrial regulatory pathways (reviewed in Sen and Cox, 2017) as well as in lipid metabolism (reviewed in Kühnlein, 2012). While these similarities support direct modeling of human peroxisome diseases in flies, the unexplored differences in flies will also likely provide valuable insight into novel aspects of peroxisome biogenesis and function.
Conservation of Peroxisome Metabolic Activity in Drosophila Cells
The effects of mutation of Pex and other peroxisome linked genes on systemic metabolism and development provides some indication that peroxisome metabolism in Drosophila is more similar to that in mammals than to yeast. Pex3 mutants had no detectable peroxisomes in their Malpighian tubule cells and died as larvae (Nakayama et al., 2011). Pex3 mutants were hypersensitive to starvation with a strong reduction in lipid catabolism (Faust et al., 2014). Mutations in Pex19 similarly showed reduced viability and dietary lipid sensitivity (Bulow et al., 2018). Pex16 mutant adults have reduced body weight (Nakayama et al., 2011). Pex2 and Pex16 mutants were viable, but had increased sensitivity to glucose starvation suggesting reduced ability to metabolize lipids for energy (Wangler et al., 2017a). The number of peroxisomes in the Malpighian tubules is reduced in Pex16 mutants and there is a two-fold elevation in VLCFA (>C24) levels compared to wild type. Mutations of Pex16 does not affect circulating levels of MCFAs (<C18). There are viable and lethal mutations in Pex10. Viable Pex10 males are sterile with defects in testes development. Adding VLCFAs to the diet, enhances the testes defect phenotype while addition of LCFAs has no effect (Chen et al., 2010). These same VLCFA-dependent testes phenotypes are also seen with Pex14 mutations (Chen et al., 2010). Pex5 mutants have an overabundance of VLCFAs compared to wild type flies (Di Cara et al., 2018, 2019). The somewhat specific response of Pex mutants in terms of VLCFA levels, supports a model that peroxisomes in flies are responsible for VLCFA metabolism, analogous to their role in mammalian cells.
Management of Reactive Oxygen Species
A major peroxisome function is management of ROS. The linkage of Drosophila peroxisomal ROS-managing enzymes has largely been studied in the course of studying the effects of free radicals on aging. The activities of the antioxidant enzymes SOD, catalase, and glutathione reductase were examined across the lifespan of adult male Drosophila, and found to exhibit individual patterns of change with aging (Sohal et al., 1990). Measures of oxidative stress increased with age, as did total SOD activity, while catalase activity decreased sharply up to 10 days before death (Sohal et al., 1990). Over-expressing catalase and SOD in flies decreased overall oxidative stress, improving old-age fly activity and oxygen consumption rates, and enhancing longevity (Orr and Sohal, 1994). Similarly, the reduced longevity and motor deficits induced by peroxides generated from overexpression of the Drosophila homolog of CG5009 (ACOX1) in wrapping glia was rescued by over-expression of catalase (Chung et al., 2020). Over-expressing human SOD1 in Drosophila motor neurons had a similar effect, suggesting age-related increases in oxidative stress had significant impact on nervous system function, and demonstrating conservation of SOD (Parkes et al., 1998a). Caloric restriction in flies was found to reduce peroxisome proliferation and improve longevity whereas mitochondrial ROS gene expression had the opposite effect (Zhou et al., 2012). Finally, Di Cara et al. (2018) did show direct effects on ROS management in S2 cells where Pex5 has been targeted by RNAi. Together, these data suggested that the ROS management activities of peroxisomes were conserved between flies and humans. Further, it appears that Drosophila peroxisomes are the primary modulators of a diet-responsive oxidative state as tissue- and age-specific sensitivities to ROS were impacted in opposite ways in mitochondria and peroxisomes. From these initial studies, it may also be inferred that lipid metabolism, a major source of diet-related peroxisomal ROS in other species, is largely conserved between Drosophila and mammals.
Lipid Oxidation
An analysis of the Drosophila proteome predicted multiple homologs for each of the five enzymes of the mammalian β-oxidation pathway, though none of the fly versions have been well characterized (Faust et al., 2012). Reporter chimeras of a predicted ACOX ortholg, CG17544 and Mtpα, co-localized with peroxisome marker PMP34-Cerulean in S2 cells. Several other predicted proteins had a variant PTS1 at their C-terminus, suggesting they also localized to peroxisomes (Faust et al., 2012). One predicted L-bifunctional protein, CG3415, had its quaternary structure resolved by X-ray scattering and was found to be very similar to that of human MFE-2, a known peroxisomal β-oxidation enzyme (Mehtala et al., 2013). A comprehensive screen of all predicted Drosophila peroxisomal proteins by Baron et al. (2016) found the most putative β-oxidation enzyme homologs identified by Faust et al. (2012) were greater than 75% co-localized with the peroxisome marker GFP-PTS1.
The functional roles for peroxisomes in terms of lipid β-oxidation in Drosophila appears to be similar to that of mammals, VLCFA levels were elevated in flies with Pex2 or Pex10 loss of function mutations (Chen et al., 2010). A metabolomics approach was used by Wangler et al. (2017a) to examine biochemical pathways affected by Pex2 and Pex16 mutation. They observed Pex16 mutants were extremely sensitive to low sugar food, directly linking Pex16 to glucose metabolism. Gas chromatography and mass spectrometry of Pex16 mutants revealed changes in the abundance of C24, C26, C28, and C30 species of VLCFAs (Wangler et al., 2017a). Similarly Pex5 mutant embryos showed an increase in VLCFAs, especially C24 (Di Cara et al., 2019).
A prerequisite for fatty acid β-oxidation is activation of fatty acids through ligation to coenzyme A (CoA), which is catalyzed by acyl-CoA synthetase (ACS; Krisans et al., 1980; Singh et al., 1992). Drosophila ACS, is encoded by the Acsl gene. Acsl was confirmed to regulate the conversion of VLCFA C16:1 to C16:1-CoA (Huang et al., 2016). Acsl loss resulted in overgrowth of neuromuscular junctions and increased phosphoethanolamine ceramide levels, the Drosophila equivalent of sphingomyelin, in motor neurons. These phenotypes were rescued by human ACSL4 expression, establishing functional homology (Huang et al., 2016). There are several ACOX homologs in Drosophila although the closest homolog to ACOX1 is CG5009. Loss of activity of CG5009 resulted in glial/axonal loss, reduced lifespan, impaired synaptic transmission and pupal death (Chung et al., 2020). These phenotypes were rescued by expression of human ACOX1. This strongly suggested Drosophila acyl-Coenzyme A oxidase at 57D genes are conserved in terms of the rate-limiting, peroxide-producing, peroxisomal-localized first step of VLCFA β-oxidation performed by their mammalian counterpart. The remaining homologs of peroxisomal lipid oxidation enzymes identified by Faust et al. (2012) await functional characterization in terms of their role in lipid metabolism.
Bulow et al. (2018) showed that homozygous Pex19 mutation results in an increase in free fatty acids, leading to lipotoxicity via altered abundance in MCFAs due to down-regulated mitochondrial lipolysis. Restoring MCFA abundance by dietary supplementation rescued the phenotype in flies and PEX19 patient cells (Sellin et al., 2018). Similar changes in MCFA abundance and altered mitochondrial lipolysis were observed in Pex2, Pex3 and Drp1 mutant larvae (Chao et al., 2016; Bulow et al., 2018; Sellin et al., 2018). In the case of Drp1 mutants, aberrant mitochondrial morphology was observed in nervous and muscle tissue (Chao et al., 2016). Given the changes in lipid metabolism observed in Pex mutants, it would seem that the roles for peroxisomes and mitochondria in terms of β-oxidation in Drosophila appear to be more similar to mammals than to yeast.
Lipid Synthesis
What is known regarding the conservation of this peroxisome activity in Drosophila lipid synthesis activity is limited largely to sequence-based homology prediction of the constituent enzymes. The exception is the cytosolic DHAP reductase/GPD1 homolog Gpdh1, whose oxidase (dehydrogenase) activity has been characterized (O’Brien and Macintyre, 1972; Niesel et al., 1980). The ability of Gpdh1 to reduce DHAP, as required by the ether lipid synthesis pathway, has not been explicitly observed in Drosophila. The human homolog GPD1 does have this capability (Reyes et al., 2015). Several homologs were predicted for other mammalian ether lipid synthesis enzymes by Faust et al. (2012). One of these was the screen for factors that affect Drosophila response to viral infection identified the potential FAR homolog Sgroppino described above (Faust et al., 2012; Merkling et al., 2019). FAR proteins function by reducing fatty acyl-CoA into a fatty alcohol molecule, however, this function has yet to be directly confirmed in Drosophila. What has been shown is that loss of Sgroppino interfered with β-oxidation in adults resulting in increased levels of triacylglycerol (TAG) and increased body mass (Merkling et al., 2019). Similarly, trafficking of Gpdh1 to peroxisomes has not been confirmed, though a variant PTS1 was identified at the C-terminus of Gpdh1 (Wojtas et al., 1997).
Developmental Defects Linked to Peroxisome Dysfunction in Drosophila
There seems to be a varied phenotypic spectrum associated with mutations in Pex and other peroxisome-linked genes during development. The mRNA encoding most Pex genes is expressed ubiquitously during early embryo development, although some (Pex7, Pex13, Pex14, Pex19) display differences in relative mRNA levels between various cell lineages (Leader et al., 2018; Pridie and Simmonds, 2020). However, likely due to a large maternal contribution of peroxisomes, fly embryos develop into larvae even when homozygous for most Pex gene mutations. Embryos homozygous for a Pex1 null mutation had a severe effect on larval growth when the animals were raised on a diet with yeast as the primary food source (Mast et al., 2011). Pex1 mutants also have defects in sperm production (Chen et al., 2010). However, insertion of a transposable element into Pex6 does not affect viability or fertility (Bellen et al., 2004). Loss of Pex3 eliminates peroxisome formation and mutants die before pupariation (Nakayama et al., 2011). An insertional mutation of Pex5 is lethal at or before pupariation with increased cell death. Pex7 mutants are viable (Di Cara et al., 2019; Pridie and Simmonds, 2020). Mutations of genes encoding proteins of the importomer Pex2, Pex10 and Pex12 are viable but are associated with sterility phenotypes. Flies with Pex13 mutations survive to adult although there are some effects on fertility (Chen et al., 2010). Currently no mutations of Pex14 are available. PEX16 is essential for peroxisome biogenesis in humans, Pex16 maternal zygotic mutant embryos retained some peroxisome-like structures into the larval stage while over-expression of Pex16 led to fewer and larger peroxisomes (Nakayama et al., 2011). The major developmental phenotypes of Pex16 loss are male sterility and the ry eye phenotype (Nakayama et al., 2011). Similar to Pex5, Pex19 mutant flies die before the end of the larval stages, with some reaching adulthood. However, if Pex19 activity is eliminated in the oocyte and the zygote then mutants die as embryos (Bulow et al., 2018).
Mutations Affecting Potential Peroxisome Fission Factors
Mutations affecting potential peroxisome fission factors (Figure 2) have not been well characterized. Mutation or dsRNA knockdown of Pex11, CG13827 or CG33474 are largely viable and fertile (Thurmond et al., 2019). The only reported phenotype is mild defects in sensory bristle patterning with tissue-specific Gal4/UAS driven expression of dsRNAs targeting CG13827 (Mummery-Widmer et al., 2009). Mutations in Drp1 and Tango11 cause lethality before the end of larval stages and targeted RNAi in neurons leads to similar alterations in bristle morphology as CG13827 altered mitochondrial morphology (Mummery-Widmer et al., 2009; Zhou et al., 2019). Targeted expression of dsRNAs targeting Fis1 in motor neurons leads to defects in axonal transport (Liu et al., 2012).
Peroxisome Membrane Proteins
There are relatively few mutants of genes encoding fly homologs of peroxisome membrane proteins (Table 2) and most UAS-RNAi lines or existing mutants are viable (Thurmond et al., 2019). There are several mutants for ABCD which are viable and fertile, but targeted expression of UAS-RNAi targeting ABCD causes defects in the developing CNS and retina (Gordon et al., 2018). Expression of UAS-RNAi constructs targeting Orct in the fat body in third instar larvae cause a lipid storage phenotype (Fan et al., 2017). Similarly, there are few available mutants in genes encoding homologs of peroxisome enzymes (Tables 3, 4). Of those that are available, most are viable and fertile (Thurmond et al., 2019). However, mutations in Cat, Hmgcr, Acsl, Lon or Sod1 have been isolated and all are lethal although in the case of Lon, this may be caused by effects on mitochondrial function (Griswold et al., 1993; Perrimon et al., 1996; Parkes et al., 1998b; Zhang et al., 2009; Liu et al., 2014; Pareek et al., 2018). Flies with homozygous recessive mutations in Ascl or Nos have CNS defects (Zhang et al., 2009; Liu et al., 2014; Rabinovich et al., 2016) and Mfe2 mutants cause a small larva phenotype (Zhou et al., 2019). Finally, flies with homozygous Cat, Mul1 and Mtpα mutations are viable but have a reduced lifespan (Missirlis et al., 2003; Kishita et al., 2012; Doktór et al., 2019). Generally developmental defects associated with Pex gene mutations in Drosophila appear to be most prevalent in the CNS and PNS, gonad, innate immunity, gut development/repair as well as an interesting link to aging.
Neural/Neuromuscular Development
Neural development is strongly affected in flies with Pex gene mutations. Several neuronal lineages showed disorganized structure in the developing Pex1 mutant embryo (Mast et al., 2011). Pex5 mutants showed defects in the embryonic CNS (Di Cara et al., 2019). Although loss of Pex19 function is generally lethal, rare adults do survive (escapers). These adult eascapers had elevated apoptotic activity in their optic lobes, suggesting neurodegeneration, as well as poor negative geotaxis and an inability to fly or inflate their wings (Bulow et al., 2018). Pex3 RNAi and loss-of-function mutation resulted in a lethal partially eclosed (dead) phenotype. This inability to break out of the pupal case was traced to defects in adult muscle function, thought to be due to defect neuromuscular junction activity (Faust et al., 2014). Notably, loss of Acsl resulted in the same phenotypes as Pex3 mutation, however, the mechanism was traced to compromised neuromuscular junctions due to neurodegeneration of motor neurons and glia, rather than musculature defects (Huang et al., 2016).
Neuronal degeneration is also seen with mutations affecting ACS function in flies. There are two Drosophila genes encoding homologs of the human ACS protein family, bubblegum (bgm) and heimdall (hll, aka doublebubble). Both are most similar to human ACS bubblegum family member 2 protein. bgm/hll double knockout resulted in animals with neurodegeneration and VLCFA accumulation somewhat similar to that of adrenoleukodystrophy (Wiesinger et al., 2013; Sivachenko et al., 2016). Activated fatty acid molecules, such as very long-chain acyl-CoA ester, are a substrate for the fatty acid transporter ATP-binding cassette sub-family D member 1 (ABCD1; Wiesinger et al., 2013). Knockdown of the potential Drosophila ABCD1/2 homolog showed optic lobe neurodegeneration very similar to that observed in the Bgm/Hll double knockout strain (reviewed in Gordon et al., 2018).
Given that mutation in many Pex and peroxisome enzyme genes cause CNS/PNS defects, an intriguing possibility is that ROS is used commonly as a signaling molecule during development. The role of ROS as a paracrine signal in CNS/PNS development has not been shown. However, ROS is used as a paracrine signal in at least one fly cell lineage, the cardiomyocyte (Lim et al., 2014). The adult Drosophila heart is a linear tube made of two columns of cardiomyocytes (CMs) encased in a sheath of non-muscle pericardial cells (PCs) called a pericardium. In mammals there is cross-talk between the various PC and CM subtypes that is essential for the heart to respond to physiological and pathological cues (reviewed in Tian and Morrisey, 2012). Elements of that relationship are preserved in Drosophila, as PCs influence both myocardial development and heart function (Lim et al., 2014). Adult PCs have higher ROS abundance than CMs in vivo. ROS reduction in PCs resulted in increased arrhythmia (Lim et al., 2014). Reducing ROS activity by increased expression of Catalase or Sod1 lead to hearts that were narrower at 1 week post-eclosion, and by 4 weeks post-eclosion the diastolic diameter had become so great that the heart tubes were enlarged (Lim et al., 2014). The phenotype was not observed when ROS levels were altered in CMs, nor did altering PC ROS levels affect CM ROS levels, indicating the phenotype was not due to ROS diffusion from PC-to-CM (Lim et al., 2014). A possibility that will need to be considered is the use of ROS as a paracrine signal in fly development. The ROS-mediated paracrine signaling observed in cardiomyocytes, which would likely involve peroxisome activity and it will be particularly interesting to see if similar mechanisms are involved in cell signaling in the developing CNS/PNS or other organs.
Gonad Development
Homozygous Pex2, Pex10 and Pex12 null mutants were viable and survived to adulthood, however, males were sterile and females had reduced fertility. This was caused by a defect in sperm development correlated to increased VLCFA abundance, which was proposed to cause a failure in cytokinesis during meiosis (Chen et al., 2010). Adult Pex16 mutant males also had the same sterility phenotype as Pex2 and Pex10 adult males, though the phenotypic defect was traced to arrested spermatocyte development, not cytokinesis failure (Nakayama et al., 2011). Given the sterility phenotypes associated with these Pex genes, it would seem that multiple steps of sperm development are acutely sensitive to fatty acid levels regulated by peroxisomes. At this point, little is known about the specific mechanistic requirements for peroxisome activity in the Drosophila ovaries. However, it has been shown recently that there are distinct differences in TAG metabolism between male and female flies (Wat et al., 2020). Whether there are corresponding sex-specific differences in peroxisome activity, especially in the gonads, remains to be determined.
Immunity
In the Drosophila circulatory system, hemocytes circulate in a blood-like fluid called hemolymph. Circulating hemocytes act analogously to mammalian macrophages as the principal effectors of the Drosophila immune response (reviewed in Parsons and Foley, 2016). In mammals, when a macrophage initiates phagocytosis it releases ROS to damage the pathogen being engulfed (reviewed in Thomas, 2017). Drosophila S2 cells are thought to be of hemocyte lineage (Cherbas et al., 2011). Peroxisomes in S2 cells were observed associating with phagosomes surrounding E. coli actively moving to the site of the nascent phagocytic cup (Di Cara et al., 2017). RNAi knockdown of Pex5 or Pex7 compromised uptake of E. coli and C. albicans due to defects in actin organization surrounding the phagocytic cup, and defects in ROS management. Over-expression of Catalase rescued phagocytosis in cells treated with dsRNA targeting Pex7, but not when Pex5 was targeted (Di Cara et al., 2017). This same effect was observed in primary hemocytes. Adult flies with hemocyte-specific Gal4/UAS expression dsRNAs targeting Pex5 or Pex7 mRNAs died within 7 days of infection. The Pex5 RNAi-treated flies were also more sensitive to injury (Di Cara et al., 2017). A similar response was seen adult Drosophila midgut microbial response when Pex5 was RNAi targeted resulted (Di Cara et al., 2018).
Gut Development and Repair
Studies in Drosophila have shown clearly that peroxisome function is essential to development and tissue homeostasis in the intestine. Gal4/UAS mediated expression of Pex5 dsRNAs in these organs resulted in lethally high levels of autophagy due to AMPK-dependent TOR kinase inhibition (Di Cara et al., 2018). Gut epithelia with dysfunctional peroxisomes had increased abundance of non-esterified fatty acids and increased redox stress, severely disrupting gut homeostasis (Di Cara et al., 2018). Loss of Pex2 or Pex10 in the Drosophila gut blocked intestinal stem (progenitor) cells from maturing into mature enterocytes in damaged midgut (Du et al., 2020). Through a combination of mutant analysis, Gal4/UAS mediated transgene expression and dsRNA knockdown Du et al. (2020) showed that peroxisomes are required in a cell autonomous manner for stem cell differentiation. Comparitive mRNA-seq identified the likely mechanism for increased peroxisome numbers in the midgut was an enhancement in RAB-7 maturation to promote stem cell/progenitor cell differentiation through the JAK/STAT-SOX21A signaling pathway (Du et al., 2020). Notably, this requirement for increased peroxisome activity for repair of damaged intestinal epithelia in Drosophila is conserved in mice and humans as well (Du et al., 2020). This suggests a potentiality that peroxisomes, along with mitochondria and other organelles like the endosomal pathway coordinately integrate lipid metabolism with other internal and external metabolic signals in the gut to maintain organ homeostasis, especially after injury (reviewed in Bellec and Cordero, 2020).
Aging
Ectopic Catalase over-expression in mitochondria and the cytosol were found to increase adult Drosophila oxidative stress resistance but not improve longevity (Mockett et al., 2003). In terms of system-wide changes, flies have proven to be particularly amenable to RNA expression RNA-Seq of dietary restricted flies with enhanced lifespan compared to wild type flies showed elevated expression of Catalase (Cat), Pex16, ry, Dodecenoyl-CoA delta-isomerase (Dci) and Sod (Li et al., 2019). A similar experiment showed changes in the relative levels of Pex1, Pex2, Pex5, Pex6, Pex7, Pex10, Pex11, Pex16, and Pex19 mRNA in aged flies compared to those treated with Paraquat, a herbicide that strongly induces ROS (Huang et al., 2019). Together, these findings suggest enhanced peroxisome biogenesis and up-regulated expression of peroxisomal ROS management enzymes improved longevity, whereas increased mitochondrial ROS gene expression only improved tolerance to oxidative stress, i.e., the ROS generated by oxidative phosphorylation. Like what was shown in terms of gut homeostasis, peroxisome function plays a role in aging-related inflammation induced cardiac disease. Huang et al. (2020) showed that reduction in peroxisome protein import in oenocytes, either experimentally by reduction of factors regulating peroxisome import of Pex1, Pex5, or Pex14 activity, disrupted ROS homeostasis cause by Paraquat treatment or due to aging, led to cardiac arrhythmia (Huang et al., 2020). Notably, the age-related effect on the heart could be suppressed by Gal4 > UAS-Pex5 expression in the oenocytes and that the effect was mediated by induction of the cytokine Unpaired 3, a JAK/STAT ligand (Hombría et al., 2005). Again, this newly discovered role for peroxisome function seems to be conserved between flies and mammals (Huang et al., 2020).
There are likely multiple yet-to-be discovered roles for peroxisomes in integrating multiple signals to help mediate tissue homeostasis. The mechanisms identified so far range from regulating ROS or lipid metabolism to more direct effects on secretion of cell-signaling molecules. Notably, sex-specific differences peroxisome-linked effects on aging have been identified. Female flies with non-lethal mutations in Pex1 or Pex13 had reduced peroxisome proliferation, reduced cellular peroxide levels and, paradoxically, increased lifespan compared to males (Zhou et al., 2012). What will be particularly interesting as this field develops is understanding the coordination between peroxisomes and other organelles like mitochondria, lipid droplets, the endosomal pathway and lysosomes in mediating these coordinating events in the specialized cells that make up these different organs. Knockdown of Pex13 in the fat body by RNAi causes mitochondrial fragmentation supporting functional linkage between mitochondria and peroxisomes (Zhou et al., 2019). Similarly, it will be interesting to see if peroxisomes in these cells have evolved correspondingly specialized activities, including sex-specific differences.
Measuring the Dynamic Movement of Peroxisome Movement in S2 Cells
The study of peroxisome movement in S2 cells, is distinct from the functional characterization of peroxisomes as described above. S2 cells have been used to study Drosophila protein localization to peroxisomes as well as peroxisome metabolism or function in microbial phagocytosis. However, unique aspects of their microtubule organization has been leveraged to study peroxisome transport on the cytoskeleton. In yeast and mammalian cells, peroxisomes are subject to constant relocation involving dynamic elements of the cytoskeleton (Subramani, 1998). Electron microscopy of Chinese hamster ovary cells revealed multiple peroxisome-microtubule contact sites (Rapp et al., 1996). In vivo kinetic analysis determined chemical disruption of both microtubule and actin filaments reduced peroxisome movement, as did ATP depletion, implying peroxisomes were moved by motor proteins (Rapp et al., 1996). Drosophila peroxisomes appear to move along microtubules like they do in mammalian cells, rather than the actin/myosin transport mechanism characteristic of yeast peroxisomes (reviewed in Neuhaus et al., 2016).
The establishment of a S2 cell line stably expressing GFP-PTS1, has become a model of choice for in vivo peroxisome dynamics studies. When S2 cells are grown on surfaces coated and treated with Cytochalasin D they extend processes less than one micrometer in diameter with bundles of microtubules orientated in one direction. Combined, these two approaches allow extremely precise measurement of the movement of peroxisomes along microtubules in live cells (Kural et al., 2005). Using this system Kinesin-1 and Dynein heavy chain were found to be the motor proteins responsible for salutatory peroxisome movement along microtubules in Drosophila (Kural et al., 2005). Peroxisome-associated microtubules contributed to individual organelle movement in a motor protein-dependent manner, and opposite-polarity motors interacted to promote bi-directional peroxisome movement (Kulic et al., 2008; Ally et al., 2009). Kinesin heavy chain anchors Kinesin-1 to peroxisomes, and thus regulates retrograde peroxisome movement. Using single particle tracking of peroxisomes at millisecond time resolution, the saltatory movement of peroxisomes via a plus-minus motor tug of war along microtubules was observed. The precise measurements facilitated by the microtubule organization of S2 cells showed that the saltatory pattern was due to cross-linking to multiple microtubules which communicated via the peroxisome membrane (De Rossi et al., 2017). S2 cell studies are also starting shed light on other potential regulatory factors affecting peroxisome movement on microtubules. A whole-genome dsRNA screen for factors affecting peroxisome transport found that the Ser/Thr kinase Darkener of Apricot, bound to eukaryotic translation elongation factor 1γ, were critical regulators of microtubule-based transport of peroxisomes (Serpinskaya et al., 2014).
Summary: A Rosy Future for Flies as a Model System to Study Peroxisomal Disorders
The localization and function of most Drosophila peroxisome protein homologs has been validated experimentally, in S2 cells and in many cases in whole animals. However, there are still several enzymes where a peroxisomal localization has not been validated experimentally, especially those that do not have a canonical PTS1 targeting sequence (Table 4). It may be that these enzymes are cytoplasmic and metabolites pass in and out of the peroxisome or alternatively, it may be that the tagging approach used by Baron et al. (2016) interfered with localization. Validation of a peroxisomal role/localization will require alternative approaches. Similarly, there a few missing homologs to key enzymatic pathway steps known to occur in peroxisomes in yeast in humans. It may be that other proteins can substitute functionally or alternatively that these steps are missing in flies. Screens for metabolic requirements, focused on peroxisomal function would help clarify these missing steps.
Drosophila screens have identified several novel aspects of peroxisome genes that need further examination. One example of this is a potential role for localized regulation of translation of mRNAs encoding peroxisome proteins. Fly embryos are strongly dependent on regulated mRNA localization and translation. The first 90 min of embryo development occurs largely without zygotic gene transcription but requires a large store of energy stored as lipid in the yolk, which would likely maintenance of the peroxisome population via proliferation and pexophagy. Many Pex mRNAs show highly specific, often punctate, patterns in the large cytoplasm of the oocyte or syncytial early embryo suggesting post-transcriptional gene regulation (reviewed in Hughes and Simmonds, 2019).
Another major unanswered question is the variable requirement/activities of peroxisomes in different organs/cell lineages. Notably, patients with Pex mutations often have variable defects in organ development or function, especially the CNS (reviewed in Faust et al., 2005). The simple body plan and CNS/PNS structure of fly larvae and adults, combined with the facile Gal4/UAS mediated tissue-specific gene targeting provides a facile platform to begin to dissect the requirements for peroxisome activity in general in the CNS and other organs. The same approach can be used to probe the tissue-specific effects of mutations in Pex genes or those encoding specific peroxisome enzymes. Flies also represent an excellent animal to examine the coordinated roles of peroxisomes, mitochondria and lipid droplets in terms of lipid homeostasis. Notably, mutation or tissue-specific knockdown of gene encoding enzymes like Mtpα, Orct or CG9527 (ACOX) that localize to fly peroxisomes lead to changes in the size or morphology of lipid droplets. Similarly, targeted knockdown or mutation of Pex1, Pex13, Pex19, Crat, Mfe2 and Mul1 lead to changes in mitochondrial morphology. The linkage between peroxisome activity and mitochondrial function is particularly intriguing given the discovery that MCFAs can suppress the effects of Pex19 mutation, including those effect on mitochondria (Sellin et al., 2018).
Helping to answer these and other novel aspects of fly peroxisomes will be new reagents/technologies becoming available to study peroxisomes in flies. Community-wide efforts have generated extensive collections of new Pex and other peroxisome gene mutants which are then made available to the greater academic research community. A good example is the TRiP Toolbox generated by the Harvard Medical School DRSC/TRiP Functional Genomics Resources group (Perkins et al., 2015; Hu et al., 2017; Zirin et al., 2020). The TRiP Toolbox combines the targeting capacity of the Gal4-UAS binary expression system with lines expressing specific gene targeting dsRNAs as well as CRISPR-Cas9 to produce targeted in vivo mutagenesis of a gene of interest (reviewed in Lin et al., 2015). This system can be used for targeted gene knockout (TRiP-KO) or, using a deactivated variant of Cas9 (dCas9), targeted gene over-expression (TRiP-OE). Similarly, the collection of tissue-targeted dsRNA expressing UAS-lines continues to expand, providing a simple and effective way to knock down peroxisome-linked genes in specific tissues or to express modified forms of these genes mirroring human mutations. Similarly, it is relatively easy to profile fatty acids in a tissue or whole organism basis, further supporting the ease of use of Drosophila for studying metabolic disorders (Parisi et al., 2011; Carvalho et al., 2012; Sellin et al., 2020). This utility of flies to explore the pathophysiological effects of genes linked to rare genetic diseases, including the developmental defects associated with peroxisome disorders is now supported by multiple groups worldwide. Some examples of these consortiums include: the Undiagnosed Diseases Network, the Centers for Mendelian Genomics and the Canadian Rare Diseases Models and Mechanisms Network (Ramoni et al., 2017; Posey et al., 2019; Boycott et al., 2020; Taruscio et al., 2020); reviewed in Wangler et al. (2017b). The unique aspects of fly development, ample genetic tools and a large support system of technological advances and reagents will continue to provide new avenues of exploration to improve our understanding of peroxisomes. Thus, the ‘re’-emergence of flies as an effective and facile model system for studying peroxisomes portends a ‘rosy future.’
Author Contributions
All authors contributed substantially in terms of the concept and content of this review.
Funding
This work was supported by a grant to AS from the Natural Sciences and Engineering Research Council of Canada (NSERC) grant number (RGPIN-2017-05885).
Conflict of Interest
The authors declare that the research was conducted in the absence of any commercial or financial relationships that could be construed as a potential conflict of interest.
Acknowledgments
We would like to thank Dr. Sarah Hughes and Dr. Matthew Anderson-Baron for critical reading of this manuscript. We apologize to those whose work we could not include in this review due to limited space.
Abbreviations
ALS, amyotrophic lateral sclerosis; CNS, central nervous system; LCFA, long-chain fatty acid; MCFA, medium chain fatty acid; PNS, peripheral nervous system; PTS, peroxisome targeting signal; ROS, reactive oxygen species; UAS, upstream activating sequence; VLCFA, very long-chain fatty acid.
Footnotes
References
Adams, M. D., Celniker, S. E., Holt, R. A., Evans, C. A., Gocayne, J. D., Amanatides, P. G., et al. (2000). The genome sequence of Drosophila melanogaster. Science 287, 2185–2195. doi: 10.1126/science.287.5461.2185
Ally, S., Larson, A. G., Barlan, K., Rice, S. E., and Gelfand, V. I. (2009). Opposite-polarity motors activate one another to trigger cargo transport in live cells. J. Cell Biol. 187, 1071–1082. doi: 10.1083/jcb.200908075
Anderson-Baron, M., and Simmonds, A. J. (2018). Peroxisome protein prediction in Drosophila melanogaster. Sub Cell. Biochem. 89, 235–258. doi: 10.1007/978-981-13-2233-4_10
Androschuk, A., Al-Jabri, B., and Bolduc, F. V. (2015). From learning to memory: what flies can tell us about intellectual disability treatment. Front. Psychiatry 6:85. doi: 10.3389/fpsyt.2015.00085
Angermüller, S., Bruder, G., Völkl, A., Wesch, H., and Fahimi, H. D. (1987). Localization of xanthine oxidase in crystalline cores of peroxisomes. A cytochemical and biochemical study. Eur. J. Cell Biol. 45, 137–144.
Assia Batzir, N., Bhagwat, P. K., Eble, T. N., Liu, P., Eng, C. M., Elsea, S. H., et al. (2019). De novo missense variant in the GTPase effector domain (GED) of DNM1L leads to static encephalopathy and seizures. Cold Spring Harb. Mol. Case Stud. 5:a003673. doi: 10.1101/mcs.a003673
Baron, M. N., Klinger, C. M., Rachubinski, R. A., and Simmonds, A. J. (2016). A systematic cell-based analysis of localization of predicted Drosophila peroxisomal proteins. Traffic 17, 536–553. doi: 10.1111/tra.12384
Baum, B., and Cherbas, L. (2008). Drosophila cell lines as model systems and as an experimental tool. Methods Mol. Biol. 420, 391–424. doi: 10.1007/978-1-59745-583-1_25
Beard, M. E., and Holtzman, E. (1987). Peroxisomes in wild-type and rosy mutant Drosophila melanogaster. Proc. Natl. Acad. Sci. U.S.A. 84, 7433–7437. doi: 10.1073/pnas.84.21.7433
Beira, J. V., and Paro, R. (2016). The legacy of Drosophila imaginal discs. Chromosoma 125, 573–592. doi: 10.1007/s00412-016-0595-4
Bellec, K., and Cordero, J. B. (2020). The peroxisome: a new player in intestinal epithelial repair. Dev. Cell 53, 131–132. doi: 10.1016/j.devcel.2020.03.023
Bellen, H. J., Levis, R. W., Liao, G., He, Y., Carlson, J. W., Tsang, G., et al. (2004). The BDGP gene disruption project: single transposon insertions associated with 40% of Drosophila genes. Genetics 167, 761–781. doi: 10.1534/genetics.104.026427
Beller, M., Bulankina, A. V., Hsiao, H. H., Urlaub, H., Jäckle, H., and Kühnlein, R. P. (2010). PERILIPIN-dependent control of lipid droplet structure and fat storage in Drosophila. Cell Metab. 12, 521–532. doi: 10.1016/j.cmet.2010.10.001
Bharucha, K. N. (2009). The epicurean fly: using Drosophila melanogaster to study metabolism. Pediatr. Res. 65, 132–137. doi: 10.1203/PDR.0b013e318191fc68
Bocos, C., Göttlicher, M., Gearing, K., Banner, C., Enmark, E., Teboul, M., et al. (1995). Fatty acid activation of peroxisome proliferator-activated receptor (PPAR). J. Steroid Biochem. Mol. Biol. 53, 467–473. doi: 10.1016/0960-0760(95)00093-f
Boycott, K. M., Campeau, P. M., Howley, H. E., Pavlidis, P., Rogic, S., Oriel, C., et al. (2020). The canadian rare diseases models and mechanisms (RDMM) network: connecting understudied genes to model organisms. Am. J. Hum. Genet. 106, 143–152. doi: 10.1016/j.ajhg.2020.01.009
Braissant, O., Foufelle, F., Scotto, C., Dauça, M., and Wahli, W. (1996). Differential expression of peroxisome proliferator-activated receptors (PPARs): tissue distribution of PPAR-alpha, -beta, and -gamma in the adult rat. Endocrinology 137, 354–366. doi: 10.1210/endo.137.1.8536636
Brand, A. H., and Perrimon, N. (1993). Targeted gene expression as a means of altering cell fates and generating dominant phenotypes. Development 118, 401–415.
Bulow, M. H., Wingen, C., Senyilmaz, D., Gosejacob, D., Sociale, M., Bauer, R., et al. (2018). Unbalanced lipolysis results in lipotoxicity and mitochondrial damage in peroxisome-deficient Pex19 mutants. Mol. Biol. Cell 29, 396–407. doi: 10.1091/mbc.E17-08-0535
Carvalho, M., Sampaio, J. L., Palm, W., Brankatschk, M., Eaton, S., and Shevchenko, A. (2012). Effects of diet and development on the Drosophila lipidome. Mol. Syst. Biol. 8:600. doi: 10.1038/msb.2012.29
Chang, C. C., South, S., Warren, D., Jones, J., Moser, A. B., Moser, H. W., et al. (1999). Metabolic control of peroxisome abundance. J. Cell Sci. 112(Pt 10), 1579–1590.
Chang, J., Klute, M. J., Tower, R. J., Mast, F. D., Dacks, J. B., and Rachubinski, R. A. (2015). An ancestral role in peroxisome assembly is retained by the divisional peroxin Pex11 in the yeast Yarrowia lipolytica. J. Cell Sci. 128, 1327–1340. doi: 10.1242/jcs.157743
Chao, Y. H., Robak, L. A., Xia, F., Koenig, M. K., Adesina, A., Bacino, C. A., et al. (2016). Missense variants in the middle domain of DNM1L in cases of infantile encephalopathy alter peroxisomes and mitochondria when assayed in Drosophila. Hum. Mol. Genet. 25, 1846–1856. doi: 10.1093/hmg/ddw059
Chen, H., Liu, Z., and Huang, X. (2010). Drosophila models of peroxisomal biogenesis disorder: peroxins are required for spermatogenesis and very-long-chain fatty acid metabolism. Hum. Mol. Genet. 19, 494–505. doi: 10.1093/hmg/ddp518
Cherbas, L., Willingham, A., Zhang, D., Yang, L., Zou, Y., Eads, B. D., et al. (2011). The transcriptional diversity of 25 Drosophila cell lines. Genome Res. 21, 301–314. doi: 10.1101/gr.112961.110
Chintapalli, V. R., Terhzaz, S., Wang, J., Al Bratty, M., Watson, D. G., Herzyk, P., et al. (2012). Functional correlates of positional and gender-specific renal asymmetry in Drosophila. PLoS One 7:e32577. doi: 10.1371/journal.pone.0032577
Chung, H. L., Wangler, M. F., Marcogliese, P. C., Jo, J., Ravenscroft, T. A., Zuo, Z., et al. (2020). Loss- or gain-of-function mutations in ACOX1 cause axonal loss via different mechanisms. Neuron 106, 589–606. doi: 10.1016/j.neuron.2020.02.021
De Rossi, M. C., Wetzler, D. E., Bensenor, L., De Rossi, M. E., Sued, M., Rodriguez, D., et al. (2017). Mechanical coupling of microtubule-dependent motor teams during peroxisome transport in Drosophila S2 cells. Biochim. Biophys. Acta Gen. Subj. 1861, 3178–3189. doi: 10.1016/j.bbagen.2017.09.009
Dent, C. E., and Philpot, G. R. (1954). Xanthinuria, an inborn error (or deviation) of metabolism. Lancet 266, 182–185. doi: 10.1016/s0140-6736(54)91257-x
Di Cara, F., Bulow, M. H., Simmonds, A. J., and Rachubinski, R. A. (2018). Dysfunctional peroxisomes compromise gut structure and host defense by increased cell death and Tor-dependent autophagy. Mol. Biol. Cell 29, 2766–2783. doi: 10.1091/mbc.E18-07-0434
Di Cara, F., Rachubinski, R. A., and Simmonds, A. J. (2019). Distinct roles for peroxisomal targeting signal receptors Pex5 and Pex7 in Drosophila. Genetics 211, 141–149. doi: 10.1534/genetics.118.301628
Di Cara, F., Sheshachalam, A., Braverman, N. E., Rachubinski, R. A., and Simmonds, A. J. (2017). Peroxisome-mediated metabolism is required for immune response to microbial infection. Immunity 47, 93–106. doi: 10.1016/j.immuni.2017.06.016
Doktór, B., Damulewicz, M., and Pyza, E. (2019). Effects of MUL1 and PARKIN on the circadian clock, brain and behaviour in Drosophila Parkinson’s disease models. BMC Neurosci. 20:24. doi: 10.1186/s12868-019-0506-8
Du, G., Xiong, L., Li, X., Zhuo, Z., Zhuang, X., Yu, Z., et al. (2020). Peroxisome elevation induces stem cell differentiation and intestinal epithelial repair. Dev. Cell 53, 169–184. doi: 10.1016/j.devcel.2020.03.002
Dubois, V., Eeckhoute, J., Lefebvre, P., and Staels, B. (2017). Distinct but complementary contributions of PPAR isotypes to energy homeostasis. J. Clin. Iinvest. 127, 1202–1214. doi: 10.1172/jci88894
Fakieh, M. H., Drake, P. J., Lacey, J., Munck, J. M., Motley, A. M., and Hettema, E. H. (2013). Intra-ER sorting of the peroxisomal membrane protein Pex3 relies on its luminal domain. Biol. Open 2, 829–837. doi: 10.1242/bio.20134788
Fan, W., Lam, S. M., Xin, J., Yang, X., Liu, Z., Liu, Y., et al. (2017). Drosophila TRF2 and TAF9 regulate lipid droplet size and phospholipid fatty acid composition. PLoS Genet. 13:e1006664. doi: 10.1371/journal.pgen.1006664
Faust, J. E., Manisundaram, A., Ivanova, P. T., Milne, S. B., Summerville, J. B., Brown, H. A., et al. (2014). Peroxisomes are required for lipid metabolism and muscle function in Drosophila melanogaster. PLoS One 9:e100213. doi: 10.1371/journal.pone.0100213
Faust, J. E., Verma, A., Peng, C., and McNew, J. A. (2012). An inventory of peroxisomal proteins and pathways in Drosophila melanogaster. Traffic 13, 1378–1392. doi: 10.1111/j.1600-0854.2012.01393.x
Faust, P. L., Banka, D., Siriratsivawong, R., Ng, V. G., and Wikander, T. M. (2005). Peroxisome biogenesis disorders: the role of peroxisomes and metabolic dysfunction in developing brain. J. Inheri. Metab. Dis. 28, 369–383. doi: 10.1007/s10545-005-7059-y
Feany, M. B., and Bender, W. W. (2000). A Drosophila model of Parkinson’s disease. Nature 404, 394–398. doi: 10.1038/35006074
Feiguin, F., Godena, V. K., Romano, G., D’Ambrogio, A., Klima, R., and Baralle, F. E. (2009). Depletion of TDP-43 affects Drosophila motoneurons terminal synapsis and locomotive behavior. FEBS Lett. 583, 1586–1592. doi: 10.1016/j.febslet.2009.04.019
Fujiki, Y., Abe, Y., Imoto, Y., Tanaka, A. J., Okumoto, K., Honsho, M., et al. (2020). Recent insights into peroxisome biogenesis and associated diseases. J. Cell Sci. 133:jcs236943. doi: 10.1242/jcs.236943
Gandre-Babbe, S., and van der Bliek, A. M. (2008). The novel tail-anchored membrane protein Mff controls mitochondrial and peroxisomal fission in mammalian cells. Mol. Biol. Cell 19, 2402–2412. doi: 10.1091/mbc.E07-12-1287
Gautam, N. K., Verma, P., and Tapadia, M. G. (2017). Drosophila malpighian tubules: a model for understanding kidney development, function, and disease. Results Probl. Cell Different. 60, 3–25. doi: 10.1007/978-3-319-51436-9_1
Germain, K., and Kim, P. K. (2020). Pexophagy: a model for selective autophagy. Intern. J. Mol. Sci. 21:578. doi: 10.3390/ijms21020578
Glover, J. R., Andrews, D. W., Subramani, S., and Rachubinski, R. A. (1994). Mutagenesis of the amino targeting signal of Saccharomyces cerevisiae 3-ketoacyl-CoA thiolase reveals conserved amino acids required for import into peroxisomes in vivo. J. Biol. Chem. 269, 7558–7563.
Gordon, H. B., Valdez, L., and Letsou, A. (2018). Etiology and treatment of adrenoleukodystrophy: new insights from Drosophila. Dis. Models Mech. 11:286. doi: 10.1242/dmm.031286
Göttlicher, M., Widmark, E., Li, Q., and Gustafsson, J. A. (1992). Fatty acids activate a chimera of the clofibric acid-activated receptor and the glucocorticoid receptor. Proc. Natl. Acad. Sci. U.S.A. 89, 4653–4657. doi: 10.1073/pnas.89.10.4653
Gratz, S. J., Rubinstein, C. D., Harrison, M. M., Wildonger, J., and O’Connor-Giles, K. M. (2015). CRISPR-Cas9 genome editing in Drosophila. Curr. Protoc. Mol. Biol. 111, 31.32.31–31.32.20. doi: 10.1002/0471142727.mb3102s111
Graves, H. K., Jangam, S., Tan, K. L., Pignata, A., Seto, E. S., Yamamoto, S., et al. (2020). A genetic screen for genes that impact Peroxisomes in Drosophila identifies candidate genes for human disease. G3 10, 69–77. doi: 10.1534/g3.119.400803
Griswold, C. M., Matthews, A. L., Bewley, K. E., and Mahaffey, J. W. (1993). Molecular characterization and rescue of acatalasemic mutants of Drosophila melanogaster. Genetics 134, 781–788.
Grönke, S., Beller, M., Fellert, S., Ramakrishnan, H., Jäckle, H., and Kühnlein, R. P. (2003). Control of fat storage by a Drosophila PAT domain protein. Curr. Biol. 13, 603–606. doi: 10.1016/s0960-9822(03)00175-1
Günther, M. N., Nettesheim, G., and Shubeita, G. T. (2016). Quantifying and predicting Drosophila larvae crawling phenotypes. Sci. Rep. 6:27972. doi: 10.1038/srep27972
Guruharsha, K. G., Rual, J. F., Zhai, B., Mintseris, J., Vaidya, P., Vaidya, N., et al. (2011). A protein complex network of Drosophila melanogaster. Cell 147, 690–703. doi: 10.1016/j.cell.2011.08.047
Gutierrez, E., Wiggins, D., Fielding, B., and Gould, A. P. (2007). Specialized hepatocyte-like cells regulate Drosophila lipid metabolism. Nature 445, 275–280. doi: 10.1038/nature05382
Haataja, T. J., Koski, M. K., Hiltunen, J. K., and Glumoff, T. (2011). Peroxisomal multifunctional enzyme type 2 from the fruitfly: dehydrogenase and hydratase act as separate entities, as revealed by structure and kinetics. Biochem. J. 435, 771–781. doi: 10.1042/bj20101661
Hadorn, E., and Schwinck, I. (1956). A mutant of Drosophila without isoxanthopterine which is non-autonomous for the red eye pigments. Nature 177, 940–941. doi: 10.1038/177940a0
Hales, K. G., Korey, C. A., Larracuente, A. M., and Roberts, D. M. (2015). Genetics on the fly: a primer on the Drosophila model system. Genetics 201, 815–842. doi: 10.1534/genetics.115.183392
Hartenstein, V. (1993). Atlas of Drosophila Development. Cold Spring Harbor, NY: Cold Spring Harbor Laboratory Press.
Hehlert, P., Hofferek, V., Heier, C., Eichmann, T. O., Riedel, D., Rosenberg, J., et al. (2019). The ?/?-hydrolase domain-containing 4- and 5-related phospholipase Pummelig controls energy storage in Drosophila. J. Lipid Res. 60, 1365–1378. doi: 10.1194/jlr.M092817
Hilliker, A. J., Duyf, B., Evans, D., and Phillips, J. P. (1992). Urate-null rosy mutants of Drosophila melanogaster are hypersensitive to oxygen stress. Proc. Natl. Acad. Sci. U.S.A. 89, 4343–4347. doi: 10.1073/pnas.89.10.4343
Hoepfner, D., Schildknegt, D., Braakman, I., Philippsen, P., and Tabak, H. F. (2005). Contribution of the endoplasmic reticulum to peroxisome formation. Cell 122, 85–95. doi: 10.1016/j.cell.2005.04.025
Hombría, J. C., Brown, S., Häder, S., and Zeidler, M. P. (2005). Characterisation of Upd2, a Drosophila JAK/STAT pathway ligand. Dev. Biol. 288, 420–433. doi: 10.1016/j.ydbio.2005.09.040
Honsho, M., Asaoku, S., Fukumoto, K., and Fujiki, Y. (2013). Topogenesis and homeostasis of fatty acyl-CoA reductase 1. J. Biol. Chem. 288, 34588–34598. doi: 10.1074/jbc.M113.498345
Hu, Y., Comjean, A., Roesel, C., Vinayagam, A., Flockhart, I., Zirin, J., et al. (2017). FlyRNAi.org-the database of the Drosophila RNAi screening center and transgenic RNAi project: 2017 update. Nucleic Acids Res. 45, D672–D678. doi: 10.1093/nar/gkw977
Huang, K., Chen, W., Zhu, F., Li, P. W., Kapahi, P., and Bai, H. (2019). RiboTag translatomic profiling of Drosophila oenocytes under aging and induced oxidative stress. BMC Genom. 20:50. doi: 10.1186/s12864-018-5404-4
Huang, K., Miao, T., Chang, K., Kim, J., Kang, P., Jiang, Q., et al. (2020). Impaired peroxisomal import in Drosophila oenocytes causes cardiac dysfunction by inducing upd3 as a peroxikine. Nat. Commun. 11:2943. doi: 10.1038/s41467-020-16781-w
Huang, Y., Huang, S., Lam, S. M., Liu, Z., Shui, G., and Zhang, Y. Q. (2016). Acsl, the Drosophila ortholog of intellectual-disability-related ACSL4, inhibits synaptic growth by altered lipids. J. Cell Sci. 129, 4034–4045. doi: 10.1242/jcs.195032
Hughes, S. C., and Simmonds, A. J. (2019). Drosophila mRNA localization during later development: past, present, and future. Front. Genet. 10:135. doi: 10.3389/fgene.2019.00135
Huybrechts, S. J., Van Veldhoven, P. P., Brees, C., Mannaerts, G. P., Los, G. V., and Fransen, M. (2009). Peroxisome dynamics in cultured mammalian cells. Traffic 10, 1722–1733. doi: 10.1111/j.1600-0854.2009.00970.x
Ichikawa, M., Nishino, T., Nishino, T., and Ichikawa, A. (1992). Subcellular localization of xanthine oxidase in rat hepatocytes: high-resolution immunoelectron microscopic study combined with biochemical analysis. J. Histochem. Cytochem. 40, 1097–1103. doi: 10.1177/40.8.1619276
Jo, D. S., Park, S. J., Kim, A. K., Park, N. Y., Kim, J. B., Bae, J. E., et al. (2020). Loss of HSPA9 induces peroxisomal degradation by increasing pexophagy. Autophagy 22, 1–15. doi: 10.1080/15548627.2020.1712812
Joardar, A., Menzl, J., Podolsky, T. C., Manzo, E., Estes, P. S., Ashford, S., et al. (2015). PPAR gamma activation is neuroprotective in a Drosophila model of ALS based on TDP-43. Hum. Mol. Genet. 24, 1741–1754. doi: 10.1093/hmg/ddu587
Kiaei, M. (2008). Peroxisome proliferator-activated receptor-gamma in amyotrophic lateral sclerosis and Huntington’s disease. PPAR Res. 2008:418765. doi: 10.1155/2008/418765
Kim, P. (2017). Peroxisome biogenesis: a union between two organelles. Curr. Biol. 27, R271–R274. doi: 10.1016/j.cub.2017.02.052
Kimura, T., Kimura, A. K., Ren, M., Berno, B., Xu, Y., Schlame, M., et al. (2018). Substantial decrease in plasmalogen in the heart associated with tafazzin deficiency. Biochemistry 57, 2162–2175. doi: 10.1021/acs.biochem.8b00042
King-Jones, K., and Thummel, C. S. (2005). Nuclear receptors–a perspective from Drosophila. Nat. Rev. Genet. 6, 311–323. doi: 10.1038/nrg1581
Kishita, Y., Tsuda, M., and Aigaki, T. (2012). Impaired fatty acid oxidation in a Drosophila model of mitochondrial trifunctional protein (MTP) deficiency. Biochem. Biophys. Res. Commun. 419, 344–349. doi: 10.1016/j.bbrc.2012.02.026
Kliewer, S. A., Xu, H. E., Lambert, M. H., and Willson, T. M. (2001). Peroxisome proliferator-activated receptors: from genes to physiology. Recent Prog. Horm. Res. 56, 239–263. doi: 10.1210/rp.56.1.239
Koch, A., Thiemann, M., Grabenbauer, M., Yoon, Y., McNiven, M. A., and Schrader, M. (2003). Dynamin-like protein 1 is involved in peroxisomal fission. J. Biol. Chem. 278, 8597–8605. doi: 10.1074/jbc.M211761200
Koch, J., and Brocard, C. (2012). PEX11 proteins attract Mff and human Fis1 to coordinate peroxisomal fission. J. Cell Sci. 125(Pt 16), 3813–3826. doi: 10.1242/jcs.102178
Krisans, S. K., Mortensen, R. M., and Lazarow, P. B. (1980). Acyl-CoA synthetase in rat liver peroxisomes. Computer-assisted analysis of cell fractionation experiments. J. Biol. Chem. 255, 9599–9607.
Kühnlein, R. P. (2012). Thematic review series: lipid droplet synthesis and metabolism: from yeast to man. Lipid droplet-based storage fat metabolism in Drosophila. J. Lipid Res. 53, 1430–1436. doi: 10.1194/jlr.R024299
Kulic, I. M., Brown, A. E., Kim, H., Kural, C., Blehm, B., Selvin, P. R., et al. (2008). The role of microtubule movement in bidirectional organelle transport. Proc. Natl. Acad. Sci. U.S.A. 105, 10011–10016. doi: 10.1073/pnas.0800031105
Kural, C., Kim, H., Syed, S., Goshima, G., Gelfand, V. I., and Selvin, P. R. (2005). Kinesin and dynein move a peroxisome in vivo: a tug-of-war or coordinated movement? Science 308, 1469–1472. doi: 10.1126/science.1108408
Lang, S., Hilsabeck, T. A., Wilson, K. A., Sharma, A., Bose, N., Brackman, D. J., et al. (2019). A conserved role of the insulin-like signaling pathway in diet-dependent uric acid pathologies in Drosophila melanogaster. PLoS Genet. 15:e1008318. doi: 10.1371/journal.pgen.1008318
Lasko, P. (2020). Patterning the Drosophila embryo: a paradigm for RNA-based developmental genetic regulation. Wiley Interdiscipl. Rev. RNA 2020:e1610. doi: 10.1002/wrna.1610
Le Bourg, E., and Lints, F. A. (1992). Hypergravity and aging in Drosophila melanogaster. 4, Climbing activity. Gerontology 38, 59–64. doi: 10.1159/000213307
Leader, D. P., Krause, S. A., Pandit, A., Davies, S. A., and Dow, J. A. T. (2018). FlyAtlas 2: a new version of the Drosophila melanogaster expression atlas with RNA-Seq, miRNA-Seq and sex-specific data. Nucleic Acids Res. 46, D809–D815. doi: 10.1093/nar/gkx976
Li, A. C., Brown, K. K., Silvestre, M. J., Willson, T. M., Palinski, W., and Glass, C. K. (2000). Peroxisome proliferator-activated receptor gamma ligands inhibit development of atherosclerosis in LDL receptor-deficient mice. J. Clin. Iinvest. 106, 523–531. doi: 10.1172/jci10370
Li, J. Q., Duan, D. D., Zhang, J. Q., Zhou, Y. Z., Qin, X. M., Du, G. H., et al. (2019). Bioinformatic prediction of critical genes and pathways involved in longevity in Drosophila melanogaster. Mol. Genet. Genom. 294, 1463–1475. doi: 10.1007/s00438-019-01589-1
Lim, H. Y., Wang, W., Chen, J., Ocorr, K., and Bodmer, R. (2014). ROS regulate cardiac function via a distinct paracrine mechanism. Cell Rep. 7, 35–44. doi: 10.1016/j.celrep.2014.02.029
Lin, S., Ewen-Campen, B., Ni, X., Housden, B. E., and Perrimon, N. (2015). In Vivo Transcriptional activation using CRISPR/Cas9 in Drosophila. Genetics 201, 433–442. doi: 10.1534/genetics.115.181065
Liu, S., Sawada, T., Lee, S., Yu, W., Silverio, G., Alapatt, P., et al. (2012). Parkinson’s disease-associated kinase PINK1 regulates miro protein level and axonal transport of mitochondria. PLoS Genetics 8:e1002537. doi: 10.1371/journal.pgen.1002537
Liu, Z., Huang, Y., Hu, W., Huang, S., Wang, Q., Han, J., et al. (2014). dAcsl, the Drosophila ortholog of Acyl-CoA synthetase long-chain family member 3 and 4, inhibits synapse growth by attenuating bone morphogenetic protein signaling via Endocytic recycling. J. Neurosci. 34, 2785–2796. doi: 10.1523/JNEUROSCI.3547-13.2014
Makki, R., Cinnamon, E., and Gould, A. P. (2014). The development and functions of oenocytes. Annu. Rev. Entomol. 59, 405–425. doi: 10.1146/annurev-ento-011613-162056
Mast, F. D., Li, J., Virk, M. K., Hughes, S. C., Simmonds, A. J., and Rachubinski, R. A. (2011). A Drosophila model for the Zellweger spectrum of peroxisome biogenesis disorders. Dis. Models Mech. 4, 659–672. doi: 10.1242/dmm.007419
Mast, F. D., Rachubinski, R. A., and Aitchison, J. D. (2020). Peroxisome prognostications: exploring the birth, life, and death of an organelle. J. Cell Biol. 219:e201912100. doi: 10.1083/jcb.201912100
Mehtala, M. L., Haataja, T. J., Blanchet, C. E., Hiltunen, J. K., Svergun, D. I., and Glumoff, T. (2013). Quaternary structure of human, Drosophila melanogaster and Caenorhabditis elegans MFE-2 in solution from synchrotron small-angle X-ray scattering. FEBS Lett. 587, 305–310. doi: 10.1016/j.febslet.2012.12.014
Merkling, S. H., Riahi, H., Overheul, G. J., Schenck, A., and van Rij, R. P. (2019). Peroxisome-associated sgroppino links fat metabolism with survival after RNA virus infection in Drosophila. Sci. Rep. 9:2065. doi: 10.1038/s41598-019-38559-x
Mirza, A. Z., Althagafi, I. I., and Shamshad, H. (2019). Role of PPAR receptor in different diseases and their ligands: physiological importance and clinical implications. Eur. J. Med. Chem. 166, 502–513. doi: 10.1016/j.ejmech.2019.01.067
Missirlis, F., Rahlfs, S., Dimopoulos, N., Bauer, H., Becker, K., Hilliker, A., et al. (2003). A putative glutathione peroxidase of Drosophila encodes a thioredoxin peroxidase that provides resistance against oxidative stress but fails to complement a lack of catalase activity. Biol. Chem. 384, 463–472. doi: 10.1515/BC.2003.052
Mockett, R. J., Bayne, A. C., Kwong, L. K., Orr, W. C., and Sohal, R. S. (2003). Ectopic expression of catalase in Drosophila mitochondria increases stress resistance but not longevity. Free Rad. Biol. Med. 34, 207–217. doi: 10.1016/s0891-5849(02)01190-5
Morgan, T., and Bridges, C. (1916). Sex-Linked Inheritance in Drosophila. Washington, DC: Carnegie Institution of Washington.
Motley, A. M., and Hettema, E. H. (2007). Yeast peroxisomes multiply by growth and division. J. Cell Biol. 178, 399–410. doi: 10.1083/jcb.200702167
Motley, A. M., Hettema, E. H., Ketting, R., Plasterk, R., and Tabak, H. F. (2000). Caenorhabditis elegans has a single pathway to target matrix proteins to peroxisomes. EMBO Rep. 1, 40–46. doi: 10.1038/sj.embor.embor626
Mummery-Widmer, J. L., Yamazaki, M., Stoeger, T., Novatchkova, M., Bhalerao, S., Chen, D., et al. (2009). Genome-wide analysis of Notch signalling in Drosophila by transgenic RNAi. Nature 458, 987–992. doi: 10.1038/nature07936
Nakayama, M., Sato, H., Okuda, T., Fujisawa, N., Kono, N., Arai, H., et al. (2011). Drosophila carrying pex3 or pex16 mutations are models of Zellweger syndrome that reflect its symptoms associated with the absence of peroxisomes. PLoS One 6:e22984. doi: 10.1371/journal.pone.0022984
Neuhaus, A., Eggeling, C., Erdmann, R., and Schliebs, W. (2016). Why do peroxisomes associate with the cytoskeleton? Biochim. Biophys. Acta 1863, 1019–1026. doi: 10.1016/j.bbamcr.2015.11.022
Niesel, D. W., Bewley, G. C., Miller, S. G., Armstrong, F. B., and Lee, C. Y. (1980). Purification and structural analysis of the soluble sn-glycerol-3-phosphate dehydrogenase isozymes in Drosophila melanogaster. J. Biol. Chem. 255, 4073–4080.
O’Brien, S. J., and Macintyre, R. J. (1972). The -glycerophosphate in Drosophila melanogaster, II. Genetic aspects. Genetics 71, 127–138.
Ordonez, D. G., Lee, M. K., and Feany, M. B. (2018). α-synuclein induces mitochondrial dysfunction through spectrin and the actin cytoskeleton. Neuron 97, 108–124.e106. doi: 10.1016/j.neuron.2017.11.036
Orr, W. C., and Sohal, R. S. (1994). Extension of life-span by overexpression of superoxide dismutase and catalase in Drosophila melanogaster. Science 263, 1128–1130. doi: 10.1126/science.8108730
Palanker, L., Tennessen, J. M., Lam, G., and Thummel, C. S. (2009). Drosophila HNF4 regulates lipid mobilization and beta-oxidation. Cell Metab. 9, 228–239. doi: 10.1016/j.cmet.2009.01.009
Pantazis, P., and Supatto, W. (2014). Advances in whole-embryo imaging: a quantitative transition is underway. Nat. Rev. Mol. Cell Biol. 15, 327–339. doi: 10.1038/nrm3786
Pareek, G., Thomas, R. E., Vincow, E. S., Morris, D. R., and Pallanck, L. J. (2018). Lon protease inactivation in Drosophila causes unfolded protein stress and inhibition of mitochondrial translation. Cell Death Discov. 5:51. doi: 10.1038/s41420-018-0110-1
Parisi, M., Li, R., and Oliver, B. (2011). Lipid profiles of female and male Drosophila. BMC Res. Notes 4:198. doi: 10.1186/1756-0500-4-198
Park, J., Lee, S. B., Lee, S., Kim, Y., Song, S., Kim, S., et al. (2006). Mitochondrial dysfunction in Drosophila PINK1 mutants is complemented by parkin. Nature 441, 1157–1161. doi: 10.1038/nature04788
Parkes, T. L., Elia, A. J., Dickinson, D., Hilliker, A. J., Phillips, J. P., and Boulianne, G. L. (1998a). Extension of Drosophila lifespan by overexpression of human SOD1 in motorneurons. Nat. Genet. 19, 171–174. doi: 10.1038/534
Parkes, T. L., Kirby, K., Phillips, J. P., and Hilliker, A. J. (1998b). Transgenic analysis of the cSOD-null phenotypic syndrome in Drosophila. Genome 41, 642–651. doi: 10.1139/gen-41-5-642
Parsons, B., and Foley, E. (2016). Cellular immune defenses of Drosophila melanogaster. Dev. Comparat. Immunol. 58, 95–101. doi: 10.1016/j.dci.2015.12.019
Perkins, L. A., Holderbaum, L., Tao, R., Hu, Y., Sopko, R., McCall, K., et al. (2015). The transgenic RNAi project at harvard medical school: resources and validation. Genetics 201, 843–852. doi: 10.1534/genetics.115.180208
Perrimon, N., Lanjuin, A., Arnold, C., and Noll, E. (1996). Zygotic lethal mutations with maternal effect phenotypes in Drosophila melanogaster. Genetics 144, 1681–1692.
Pfeiffer, B. D., Jenett, A., Hammonds, A. S., Ngo, T. T., Misra, S., Murphy, C., et al. (2008). Tools for neuroanatomy and neurogenetics in Drosophila. Proc. Natl. Acad. Sci. U.S.A. 105, 9715–9720. doi: 10.1073/pnas.0803697105
Phillips, J. P., Tainer, J. A., Getzoff, E. D., Boulianne, G. L., Kirby, K., and Hilliker, A. J. (1995). Subunit-destabilizing mutations in Drosophila copper/zinc superoxide dismutase: neuropathology and a model of dimer dysequilibrium. Proc. Natl. Acad. Sci. U.S.A. 92, 8574–8578. doi: 10.1073/pnas.92.19.8574
Posey, J. E., O’Donnell-Luria, A. H., Chong, J. X., Harel, T., Jhangiani, S. N., Coban Akdemir, Z. H., et al. (2019). Insights into genetics, human biology and disease gleaned from family based genomic studies. Genet. Med. 21, 798–812. doi: 10.1038/s41436-018-0408-7
Pridie, C., and Simmonds, A. (2020). The role of Peroxin 7 during Drosophila embryonic development. Genome 19, 1–19. doi: 10.1139/gen-2019-0207
Rabinovich, D., Yaniv, S. P., Alyagor, I., and Schuldiner, O. (2016). Nitric oxide as a switching mechanism between axon degeneration and regrowth during developmental remodeling. Cell 164, 170–182. doi: 10.1016/j.cell.2015.11.047
Ramoni, R. B., Mulvihill, J. J., Adams, D. R., Allard, P., Ashley, E. A., Bernstein, J. A., et al. (2017). The undiagnosed diseases network: accelerating discovery about health and Disease. Am. J. Hum. Genet. 100, 185–192. doi: 10.1016/j.ajhg.2017.01.006
Rapp, S., Saffrich, R., Anton, M., Jäkle, U., Ansorge, W., Gorgas, K., et al. (1996). Microtubule-based peroxisome movement. J. Cell Sci. 109(Pt 4), 837–849.
Reyes, A. C., Koudelka, A. P., Amyes, T. L., and Richard, J. P. (2015). Enzyme architecture: optimization of transition state stabilization from a cation-phosphodianion pair. J. Am. Chem. Soc. 137, 5312–5315. doi: 10.1021/jacs.5b02202
Roy, S., Ernst, J., Kharchenko, P. V., Kheradpour, P., Negre, N., Eaton, M. L., et al. (2010). Identification of functional elements and regulatory circuits by Drosophila modENCODE. Science 330, 1787–1797. doi: 10.1126/science.1198374
Rucktaschel, R., Halbach, A., Girzalsky, W., Rottensteiner, H., and Erdmann, R. (2010). De novo synthesis of peroxisomes upon mitochondrial targeting of Pex3p. Eur. J. Cell Biol. 89, 947–954. doi: 10.1016/j.ejcb.2010.06.012
Schiller, S., Rosewich, H., Grunewald, S., and Gartner, J. (2020). Inborn errors of metabolism leading to neuronal migration defects. J. Inherit. Metab. Dis. 43, 145–155. doi: 10.1002/jimd.12194
Schneider, I. (1972). Cell lines derived from late embryonic stages of Drosophila melanogaster. J. Embryol. Exp. Morphol. 27, 353–365.
Schrader, M., Costello, J. L., Godinho, L. F., Azadi, A. S., and Islinger, M. (2016). Proliferation and fission of peroxisomes - An update. Biochim. Biophys. Acta 1863, 971–983. doi: 10.1016/j.bbamcr.2015.09.024
Schrader, M., Kamoshita, M., and Islinger, M. (2020). Organelle interplay-peroxisome interactions in health and disease. J. Inherit. Metab. Dis. 43, 71–89. doi: 10.1002/jimd.12083
Sellin, J., Fülle, J. B., Thiele, C., Bauer, R., and Bülow, M. H. (2020). Free fatty acid determination as a tool for modeling metabolic diseases in Drosophila. J. Insect Physiol. 2020:104090. doi: 10.1016/j.jinsphys.2020.104090
Sellin, J., Wingen, C., Gosejacob, D., Senyilmaz, D., Hänschke, L., Büttner, S., et al. (2018). Dietary rescue of lipotoxicity-induced mitochondrial damage in Peroxin19 mutants. PLoS Biol. 16:e2004893. doi: 10.1371/journal.pbio.2004893
Sen, A., and Cox, R. T. (2017). Fly models of human diseases: Drosophila as a model for understanding human mitochondrial mutations and disease. Curr. Top. Dev. Biol. 121, 1–27. doi: 10.1016/bs.ctdb.2016.07.001
Serpinskaya, A. S., Tuphile, K., Rabinow, L., and Gelfand, V. I. (2014). Protein kinase darkener of apricot and its substrate EF1gamma regulate organelle transport along microtubules. J. Cell Sci. 127(Pt 1), 33–39. doi: 10.1242/jcs.123885
Singh, I., Lazo, O., Dhaunsi, G. S., and Contreras, M. (1992). Transport of fatty acids into human and rat peroxisomes. Differential transport of palmitic and lignoceric acids and its implication to X-adrenoleukodystrophy. J. Biol. Chem. 267, 13306–13313.
Sivachenko, A., Gordon, H. B., Kimball, S. S., Gavin, E. J., Bonkowsky, J. L., and Letsou, A. (2016). Neurodegeneration in a Drosophila model of adrenoleukodystrophy: the roles of the bubblegum and double bubble acyl-CoA synthetases. Dis. Models Mech. 9, 377–387. doi: 10.1242/dmm.022244
Sohal, R. S., Arnold, L., and Orr, W. C. (1990). Effect of age on superoxide dismutase, catalase, glutathione reductase, inorganic peroxides, TBA-reactive material, GSH/GSSG, NADPH/NADP+ and NADH/NAD+ in Drosophila melanogaster. Mech. Age. Dev. 56, 223–235. doi: 10.1016/0047-6374(90)90084-s
St Johnston, D. (2002). The art and design of genetic screens: Drosophila melanogaster. Na. Rev. Genet. 3, 176–188. doi: 10.1038/nrg751
St Jules, R., Beard, M., and Holtzman, E. (1989). Cytochemical localization of a D-amino acid oxidizing enzyme in peroxisomes of Drosophila melanogaster. Tissue Cell 21, 661–671. doi: 10.1016/0040-8166(89)90077-3
St Jules, R., Setlik, W., Kennard, J., and Holtzman, E. (1990). Peroxisomes in the head of Drosophila melanogaster. Exper. Eye Res. 51, 607–617. doi: 10.1016/0014-4835(90)90092-9
Stessman, H. A. F., Willemsen, M. H., Fenckova, M., Penn, O., Hoischen, A., Xiong, B., et al. (2016). Disruption of POGZ is associated with intellectual disability and autism spectrum disorders. Am. J. Hum. Genet. 98, 541–552. doi: 10.1016/j.ajhg.2016.02.004
Subramani, S. (1998). Components involved in peroxisome import, biogenesis, proliferation, turnover, and movement. Physiol. Rev. 78, 171–188. doi: 10.1152/physrev.1998.78.1.171
Sugiura, A., Mattie, S., Prudent, J., and McBride, H. M. (2017). Newly born peroxisomes are a hybrid of mitochondrial and ER-derived pre-peroxisomes. Nature 542, 251–254. doi: 10.1038/nature21375
Taruscio, D., Baynam, G., Cederroth, H., Groft, S. C., Klee, E. W., Kosaki, K., et al. (2020). The undiagnosed diseases network international: five years and more! Mol. Genet. Metab. 129, 243–254. doi: 10.1016/j.ymgme.2020.01.004
Thomas, D. C. (2017). The phagocyte respiratory burst: historical perspectives and recent advances. Immunol. Lett. 192, 88–96. doi: 10.1016/j.imlet.2017.08.016
Thurmond, J., Goodman, J. L., Strelets, V. B., Attrill, H., Gramates, L. S., Marygold, S. J., et al. (2019). FlyBase 2.0: the next generation. Nucleic Acids Res. 47, D759–D765. doi: 10.1093/nar/gky1003
Tian, Y., and Morrisey, E. E. (2012). Importance of myocyte-nonmyocyte interactions in cardiac development and disease. Circ. Res. 110, 1023–1034. doi: 10.1161/circresaha.111.243899
Tolwinski, N. S. (2017). Introduction: Drosophila-a model system for developmental biology. J. Dev. Biol. 5:jdb5030009. doi: 10.3390/jdb5030009
Torroja, L., Chu, H., Kotovsky, I., and White, K. (1999). Neuronal overexpression of APPL, the Drosophila homologue of the amyloid precursor protein (APP), disrupts axonal transport. Curr. Biol. 9, 489–492. doi: 10.1016/s0960-9822(99)80215-2
Ugur, B., Chen, K., and Bellen, H. J. (2016). Drosophila tools and assays for the study of human diseases. Dis. Models Mech. 9, 235–244. doi: 10.1242/dmm.023762
Ui, K., Ueda, R., and Miyake, T. (1987). Cell lines from imaginal discs of Drosophila melanogaster. In Vitro Cell Dev. Biol. 23, 707–711. doi: 10.1007/bf02620984
Venken, K. J., Schulze, K. L., Haelterman, N. A., Pan, H., He, Y., Evans-Holm, M., et al. (2011). MiMIC: a highly versatile transposon insertion resource for engineering Drosophila melanogaster genes. Nat. Methods 8, 737–743. doi: 10.1038/nmeth.1662
Violante, S., Achetib, N., van Roermund, C. W. T., Hagen, J., Dodatko, T., Vaz, F. M., et al. (2019). Peroxisomes can oxidize medium- and long-chain fatty acids through a pathway involving ABCD3 and HSD17B4. FASEB J. 33, 4355–4364. doi: 10.1096/fj.201801498R
Wan, L., Dockendorff, T. C., Jongens, T. A., and Dreyfuss, G. (2000). Characterization of dFMR1, a Drosophila melanogaster homolog of the fragile X mental retardation protein. Mol. Cell. Biol. 20, 8536–8547. doi: 10.1128/mcb.20.22.8536-8547.2000
Wanders, R. J. (2013). Peroxisomes in human health and disease: metabolic pathways, metabolite transport, interplay with other organelles and signal transduction. Sub Cell. Biochem. 69, 23–44. doi: 10.1007/978-94-007-6889-5_2
Wangler, M. F., Chao, Y. H., Bayat, V., Giagtzoglou, N., Shinde, A. B., Putluri, N., et al. (2017a). Peroxisomal biogenesis is genetically and biochemically linked to carbohydrate metabolism in Drosophila and mouse. PLoS Genet. 13:e1006825. doi: 10.1371/journal.pgen.1006825
Wangler, M. F., Yamamoto, S., Chao, H. T., Posey, J. E., Westerfield, M., Postlethwait, J., et al. (2017b). Model organisms facilitate rare disease diagnosis and therapeutic research. Genetics 207, 9–27. doi: 10.1534/genetics.117.203067
Wangler, M. F., Yamamoto, S., and Bellen, H. J. (2015). Fruit flies in biomedical research. Genetics 199, 639–653. doi: 10.1534/genetics.114.171785
Wat, L. W., Chao, C., Bartlett, R., Buchanan, J. L., Millington, J. W., Chih, H. J., et al. (2020). A role for triglyceride lipase brummer in the regulation of sex differences in Drosophila fat storage and breakdown. PLoS Biol. 18:e3000595. doi: 10.1371/journal.pbio.3000595
Weavers, H., Prieto-Sánchez, S., Grawe, F., Garcia-López, A., Artero, R., Wilsch-Bräuninger, M., et al. (2009). The insect nephrocyte is a podocyte-like cell with a filtration slit diaphragm. Nature 457, 322–326. doi: 10.1038/nature07526
Whitworth, A. J., Theodore, D. A., Greene, J. C., Benes, H., Wes, P. D., and Pallanck, L. J. (2005). Increased glutathione S-transferase activity rescues dopaminergic neuron loss in a Drosophila model of Parkinson’s disease. Proc. Natl. Acad. Sci. U.S.A. 102, 8024–8029. doi: 10.1073/pnas.0501078102
Wiesinger, C., Kunze, M., Regelsberger, G., Forss-Petter, S., and Berger, J. (2013). Impaired very long-chain acyl-CoA β-oxidation in human X-linked adrenoleukodystrophy fibroblasts is a direct consequence of ABCD1 transporter dysfunction. J. Biol. Chem. 288, 19269–19279. doi: 10.1074/jbc.M112.445445
Wojtas, K., Slepecky, N., von Kalm, L., and Sullivan, D. (1997). Flight muscle function in Drosophila requires colocalization of glycolytic enzymes. Mol. Biol. Cell 8, 1665–1675. doi: 10.1091/mbc.8.9.1665
Wu, X. W., Lee, C. C., Muzny, D. M., and Caskey, C. T. (1989). Urate oxidase: primary structure and evolutionary implications. Proc. Natl. Acad. Sci. U.S.A. 86, 9412–9416. doi: 10.1073/pnas.86.23.9412
Yamamoto, S., Jaiswal, M., Charng, W. L., Gambin, T., Karaca, E., Mirzaa, G., et al. (2014). A Drosophila genetic resource of mutants to study mechanisms underlying human genetic diseases. Cell 159, 200–214. doi: 10.1016/j.cell.2014.09.002
Yan, M., Rayapuram, N., and Subramani, S. (2005). The control of peroxisome number and size during division and proliferation. Curr. Opin. Cell Biol. 17, 376–383. doi: 10.1016/j.ceb.2005.06.003
Yuan, W., Veenhuis, M., and van der Klei, I. J. (2016). The birth of yeast peroxisomes. Biochim. Biophys. Acta 1863, 902–910. doi: 10.1016/j.bbamcr.2015.09.008
Zhang, Y., Chen, D., and Wang, Z. (2009). Analyses of mental dysfunction-related ACSl4 in Drosophila reveal its requirement for Dpp/BMP production and visual wiring in the brain. Hum. Mol. Genet. 18, 3894–3905. doi: 10.1093/hmg/ddp332
Zhou, B., Yang, L., Li, S., Huang, J., Chen, H., Hou, L., et al. (2012). Midlife gene expressions identify modulators of aging through dietary interventions. Proc. Natl. Acad. Sci. U.S.A. 109, E1201–E1209. doi: 10.1073/pnas.1119304109
Zhou, J., Xu, L., Duan, X., Liu, W., Zhao, X., Wang, X., et al. (2019). Large-scale RNAi screen identified Dhpr as a regulator of mitochondrial morphology and tissue homeostasis. Sci. Adv. 5:eaax0365. doi: 10.1126/sciadv.aax0365
Keywords: Drosophila melanogaster, peroxisomes, embryo development, lipid metabolism, reactive oxygen species
Citation: Pridie C, Ueda K and Simmonds AJ (2020) Rosy Beginnings: Studying Peroxisomes in Drosophila. Front. Cell Dev. Biol. 8:835. doi: 10.3389/fcell.2020.00835
Received: 31 May 2020; Accepted: 04 August 2020;
Published: 25 August 2020.
Edited by:
Peter Kijun Kim, Hospital for Sick Children, CanadaReviewed by:
Seong-Kyu Choe, Wonkwang University, South KoreaShinya Yamamoto, Baylor College of Medicine, United States
Michael Schrader, University of Exeter, United Kingdom
Copyright © 2020 Pridie, Ueda and Simmonds. This is an open-access article distributed under the terms of the Creative Commons Attribution License (CC BY). The use, distribution or reproduction in other forums is permitted, provided the original author(s) and the copyright owner(s) are credited and that the original publication in this journal is cited, in accordance with accepted academic practice. No use, distribution or reproduction is permitted which does not comply with these terms.
*Correspondence: Andrew J. Simmonds, YW5kcmV3LnNpbW1vbmRzQHVhbGJlcnRhLmNh