- 1Center for Sport Medicine, The First Affiliated Hospital, Zhejiang University School of Medicine, Hangzhou, China
- 2Li Dak Sum & Yip Yio Chin Center for Stem Cell and Regenerative Medicine, Zhejiang University School of Medicine, Hangzhou, China
- 3Spine Lab, Department of Orthopedic Surgery, The First Affiliated Hospital, Zhejiang University School of Medicine, Hangzhou, China
For the fact that articular cartilage is a highly organized and avascular tissue, cartilage defects are limited to spontaneously heal, which would subsequently progress to osteoarthritis. Many methods have been developed to enhance the ability for cartilage regeneration, among which magnetically actuated manipulation has attracted interests due to its biocompatibility and non-invasive manipulation. Magnetically actuated manipulation that can be achieved by introducing magnetic nanoparticles and magnetic field. This review summarizes the cutting-edge research on the chondrogenic enhancements via magnetically actuated manipulation, including cell labeling, cell targeting, cell assembly, magnetic seeding and tissue engineering strategies.
Introduction
When articular cartilage is lost, it has limited self-regeneration potential due to its exquisite structural design and avascular nature, which would subsequently progress to osteoarthritis.
To date, various strategies have been developed to enhance cartilage regeneration, including microfracture, autologous chondrocyte implantation (ACI), and stem cell therapy (Zhang et al., 2016). These methods provide advantages of mimicking some features found in native cartilage tissue (Enea et al., 2015; Gobbi and Whyte, 2016), but the phenomenon of deterioration in cartilage quality was unavoidable (Agung et al., 2006). In addition, simply seeding a scaffold with MSCs or other cells cannot reach sufficient cell density and cell condensation, which impeding the tissue regeneration (Shimizu et al., 2006). With the development of the biotechnology, cell manipulation based on magnetic force, in other words, magnetically actuated cell manipulation (MAM), which can be achieved by the synergy between magnetic nanoparticles (MNPs) and magnetic field, has attracted increasing attention (Delyagina et al., 2011; Xia et al., 2018). In magnetic field, after introducing MNPs into cells or materials, magnetic force could direct cellular and matrix organization. Thus, the method confers several advantages, including remoting control ability, providing a sufficient cell density, promoting cell concentration and adhesion, which are essential for cell differentiation and tissue regeneration (Ochi et al., 2014). Furthermore, biomedical applications based on MAM would hold promises for promoting cartilage regeneration.
Here, we reviewed the state-of-art research of magnetically actuated manipulation for cartilage regeneration. First, we introduce the influence of MAM on the biological behaviors of cells, including cell labeling and the approaches that make the cells magnetically responsive. Then, the applications of MAM in vitro, in vivo, and in clinical trial were further discussed. Finally, current limitations and future perspectives on MAM were given.
Effects of MAM on the Biological Behaviors of Cells
MAM is achieved by the synergy between magnetic response element and magnetic field. As a magnetic response element, MNPs [e.g., superparamagnetic iron oxide (SPIOs) and paramagnetic molecules] have been used for cell labeling (Bulte, 2009). MNPs were mainly localized in phagocytic vacuoles, which could stably incorporate within cells (Son et al., 2015; Goncalves et al., 2017). Thus, MNPs were randomly dispersed but not accumulated intracellularly (Bakhru et al., 2012), and internalized MNPs were cleared through excretion routes or utilized by endogenous iron metabolism (Charlton et al., 2016), which avoiding toxicity. It was indicated that below 30 ug/ml, MNPs did not impair cell viability or metabolic cell activity (van Buul et al., 2011). In vivo assays indicated the accumulation of MNPs in the liver and spleen, and found that the MNPs did not modify any of the toxicological parameters with the increase of MNPs dose (Sharifi et al., 2012; Li et al., 2013; Ruiz et al., 2016). Furthermore, Mejias et al. (2013) observed the long-term toxicity of MNPs in vivo, and indicated that MNP did not compromise mouse survival, although acute toxicity were found 30 days after administration in some mouse, these were transient. Besides, the stemness and differentiation potential of MSCs was also not affected after MNPs labeling (Mohanty et al., 2018). In addition, MNPs could accelerate cell cycle progression by regulating the expression of cell cycle protein (Huang et al., 2009), and increase cells growth by reducing intracellular H2O2 through intrinsic peroxidase-like activity (Zhang et al., 2015). Furthermore, internalized MNPs did not particularly influence reactive oxygen species formation (Goncalves et al., 2017), but nanoparticles-mediated reactive oxygen species could influence the activation of the motigen-actived protein kinase pathways, which was important for promoting cell differentiation (Son et al., 2011; Rauch et al., 2013). Wang et al. (2016) indicated that MNPs could activate MAPK signal pathway to enhance osteogenic or chondrogenic differentiation of MSCs. And Hu et al. (2018) also found that cells labeled with MNPs had greater angiogenic functions, which could enhance bone or cartilage regeneration.
As another component, magnetic fields could regulate the biological behaviors of cells, such as the morphology, proliferation and differentiation, which may be relate to magnetomechanical interactions and radical pair effects when magnetic fields interactions with the cells (Miyakoshi, 2005; Zhang et al., 2014). Besides, magnetic fields could uncage bioactive factors by the magnetic nanoswitch to temporally regulate stem cell adhesion, differentiation, and mechanosensing (Kang et al., 2018). High-frequency magnetic fields would inhibit chondrocyte proliferation and induce cell apoptosis (Hsieh et al., 2008). However, a low-frequency magnetic field could be able to control the direction of magnetic labeled MSCs (m-MSCs) with a certain number, and no adverse influence was observed on chondrogenesis (Jaberi et al., 2011; Fayol et al., 2013; Goncalves et al., 2017). Furthermore, magnetic fields can moderate mast cell-mediated inflammatory reactions and accelerate clearance of M2 macrophages that is associated with inflammation resolving (Santos et al., 2015, 2016), and cell adhesion molecules upregulated when m-MSCs exposed to a MF (Caplan, 2007; van Buul et al., 2011).
Application of MAM In Vitro for Cartilage Regeneration
In general, we identified four technique notes explaining MAM, including cell targeting, magnetic seeding, magnetic scaffolds, cell assembly and other tissue engineering strategies. Table 1 and Figures 1, 2 briefly summarized the strategies of magnetically actuated manipulation.
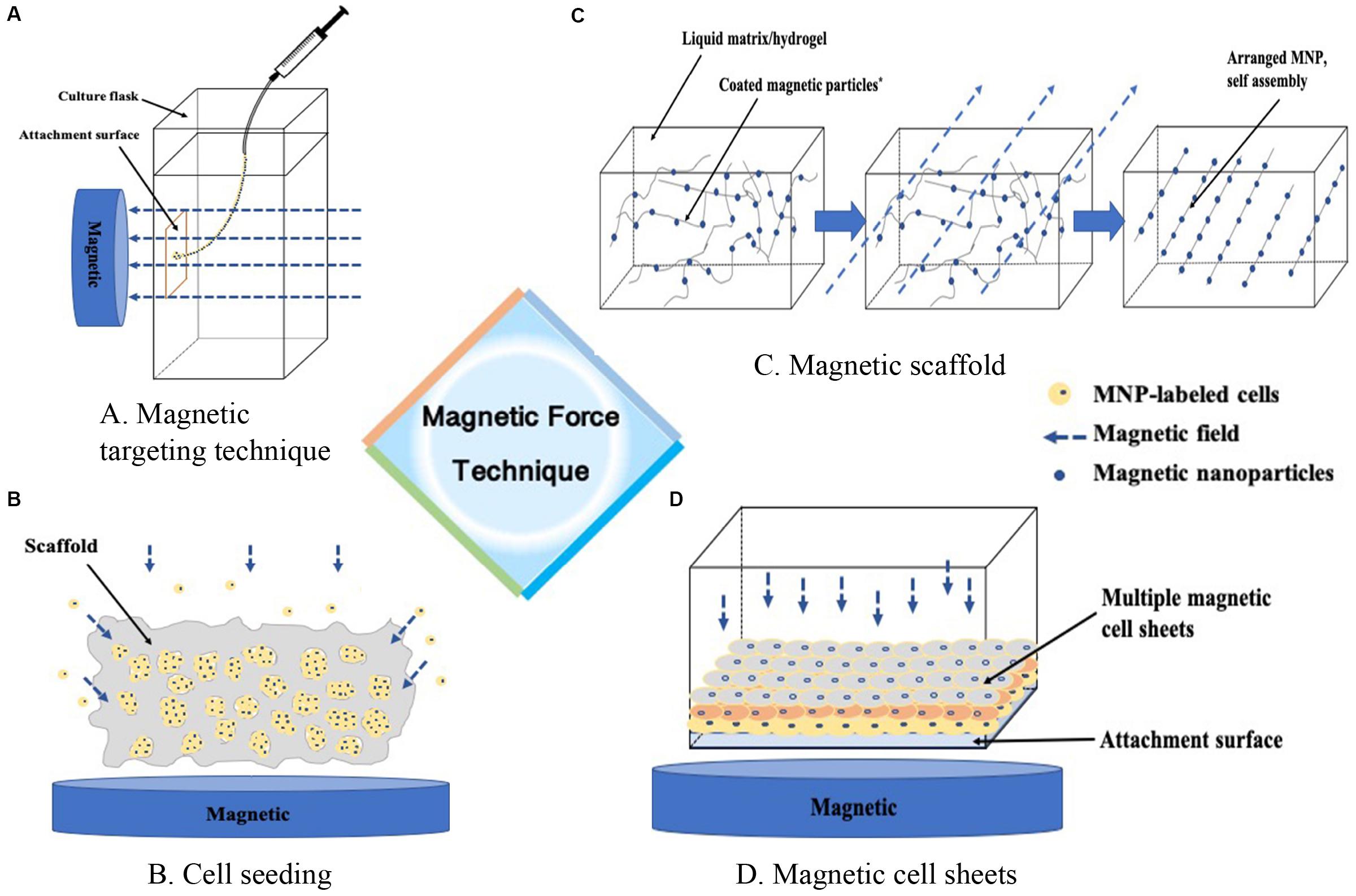
Figure 1. Magnetic force technique. (A) Guiding m-cells to a targeted site, (B) Enhancing the seeding efficacy of the cells into the scaffold, (C) Formation of ECM proteins assembly as building blocks in a liquid matrix or hydrogel, (D) Guiding cells into sheet-like structures to stack layer-by-layer, or containing different cocultured cell types.
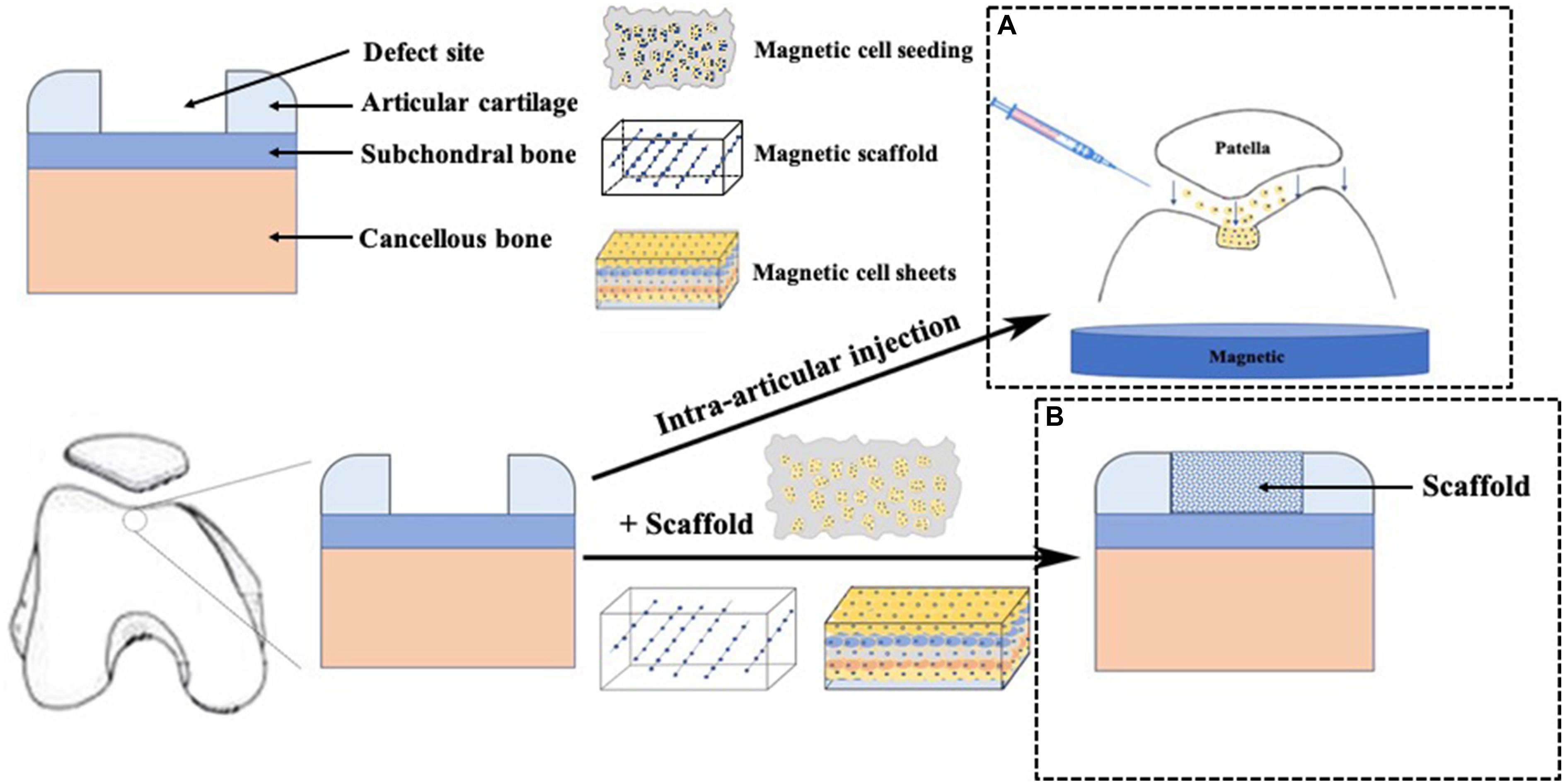
Figure 2. Application of magnetic force technique in cartilage repair. (A) cells labeled with MNPs were intra-articular injected and accumulated to the targeted cartilage defect sites using an external magnetic force, (B) A scaffold was placed in the cartilage defect site.
Guiding Cells to a Targeted Defect Site
Precise guidance of individual cells have great potential to provide convenient cellular microenvironment (Guillotin and Guillemot, 2011). Cells with magnetic force provides minimized interference with the biochemical functions of cells. We defined this procedure as magnetic targeting delivery (MTD). This technique avoided excessive number of MSCs that generated free bodies of scar tissue (Agung et al., 2006). In stead, a relatively small number of MSCs were enough to accumulate to a desired area (Hori et al., 2011; Kamei et al., 2013). Mahmoud et al. (2016) indicated that 2 × 105 m-MSCs were suitable to attain complete repair of the cartilage repair (3 mm in diameter and 4 mm in depth). Unlike intra-articular magnet that needs to be removed after translation, the external magnetic force system with less invasiveness is easy to handle, and could be completed under arthroscopy. In addition, m-MSCs exposed to MF could adhere to the target site physically, and then upregulate the expression of adhesion proteins such as integrin α2, integrin α6 and integrin β3 (Nakamae et al., 2010). Even removing the external MF, m-MSCs could still remain at the defect site (Hori et al., 2011). After the MF exposure, m-MSCs have a good adhesion, like cell sheets covering to the defect surface, which can favor cell communication and signaling among cells that are capable of spontaneous matrix formation and further promote chondrogenesis and cartilage regeneration (Goncalves et al., 2017; Vidiasheva et al., 2018). Thus, MTD could make a potential homogeneous distribution of m-MSCs to cover the defect area with high adhesion rate, which have trended toward promoted chondrogenic differentiation and cartilage regeneration. However, the application of MTD was only suitable for cartilage defect in some specific sites, such as the center of the patella and the center of lateral or medial tibial plateau, due to the fact that magnetic force is generated in only one direction and magnetic force becomes weak far from the surface of magnetic device (Kamei et al., 2013). Besides, with the friction of knee motion, there existed a risk of attached cells peeling from the defect site after MTD.
Enhancing the Seeding Efficiency of Cells Into the Scaffold
Obviously, cells and scaffolds are the essential parts of tissue engineering. The complex structure of scaffolds would cause difficulties in cell seeding, which resulted in a non-uniform and inadequate migration of cells into the scaffold (Melchels et al., 2010). It is well established that cell compaction was a critical step for cell differentiation, and the use of scaffolds could provide a suitable environment for cell proliferation and differentiation. However, a small number of MSCs seeding and lack of cell condensation into scaffold restricted chondrogenic differentiation, but another application of MAM in cell culture is magnetic force-based cell seeding into the scaffolds (MSS), which could enhance seeding efficacies to reach a sufficient cell density to promote cell condensation (Sensenig et al., 2012; Luciani et al., 2016). One study reported that magnetic labeled cells remained within pores of the scaffolds were tightly aggregated with magnetic confinement, while only individual cells were observed further from the magnetic (Luciani et al., 2016). Furthermore, MSS not only increased the number of cell attached to the scaffold, but also enhanced infiltration and distribution (Thevenot et al., 2008). Besides, MSS could enhance the rate and extent of Col II and aggrecan expression, which was favorable to chondrogenic differentiation (Fayol et al., 2013; Brady et al., 2017; Su et al., 2017). Considering the fact that the use of MTD is limited to some specific site of the knee joint, MSS seems to be more appropriate for the treatment of larger and full-thickness lesions without limitations of cartilage defect sites. However, the procedure of MSS needs surgical opening of the knee joint, which is more complicated and invasive than MTD.
Formation of Magnetic Scaffolds
MAM was also used to assemble a 3D culture structure. MNPs are cross-linked with several extracellular matrix (ECM) materials, such as alginate solution, polyvinyl alcohol, hydrogel and other ECM proteins, and then an external magnetic field was applied during forming the scaffold structure. The scaffold structure can be modulated by an alteration in the MNPs concentration and the direction and intensity of magnetic fields to mimic the native cartilage ECM structure (Margolis et al., 2018). The magnetically guided assembled scaffold, without changing the bulk stiffness, could program aligned nanofibers with several degrees of anisotropy in scaffold structure and synthesized relevant ECM molecules, which would affect seeded cells and further development (Kim and Tanner, 2016; Margolis et al., 2018). Besides, the magnetic hydrogel, without any toxic or infectious agents, was able to capture and retain magnetic-labeled cells, maintain cell phenotype and function, delivery biomaterials of therapeutic agents and cells via minimally invasive procedures, and can be used to obtain high-density cell coverage on the defect sites for cartilage repair (Bonnier et al., 2015; Xu et al., 2019). Moreover, it could support the formation of a biologically cell layer that protect the material from inflammation (Arias et al., 2018). And Wong et al. (2017) demonstrated a monolayer of RGD-bearing magnetic nanoparticles to tune the tether mobility of RGD on substrate by active mechanotransduction signaling via magnetic force, and found that MSCs cultured on this magnetic scaffold have better adhesion, spreading and osteogenic differentiation. In addition, magnetic scaffolds, with superior structure and mechanical performance, are conductive to cell adhesion and proliferation (Zhao et al., 2019). If used more effectively, magnetic scaffold with seeded cells could stimulate chondrocyte-related gene expression (Huang et al., 2018), which could be further used in cartilage repair. However, the procedure of magnetic scaffold is more complicated than MTD and MSS.
Guiding Cells Assembly Into Sheet-Like Structures to Stack Layer-by-Layer
Current 3D cell culture methods are dependent on biocompatible scaffolds, which require complex syntheses and fabrication steps. Cell sheet technology can delivery cells in an ECM context to guide the cells upon implantation without any scaffolds. Magnetic-labeled cells could be aggregated into spheroids at a targeted location on the attachment surface to form 3D arrangements in a convenient microenvironment via MAM which is an available procedure to mimic tissue properties of cells (Souza et al., 2010; Ghosh et al., 2016). Additionally, MNPs exposed to MF had the ability to make m-cells cohere together in order to reproduce different 3D geometries and cellular compositions (Souza et al., 2010). Furthermore, the layers of cell sheets could be brought into close proximity by magnetic force (Koto et al., 2017). Thus, magnetic cell sheets (MagCSs) can be used for the formation of scaffold-free 3D cell culture and cellular assemble.
The MagCSs, largely maintained by the formation of cell-to-cell junctions and secretion of ECM proteins, are free from the restriction of scaffolds, which could avoid the adverse effects of scaffolds (Gil et al., 2015). In MagCSs, the cell monolayer could be detached spontaneously via magnetic levitation and still keep an intact cell sheet. In addition, the shape of cell sheets could be precisely controlled via different magnet patterns, and it could also be manipulated to facilitate magnetized cell sheets transfer and scaffold-free tissue formation through the application of magnets (Penland et al., 2017). With the application of magnetic technology, the cell monolayer could be precisely controlled to stack layer-by-layer to form multilayer sheets or containing different cocultured cell types, which cannot be achieved by classical method of cell-sheet preparation (Ito et al., 2007; Zhang et al., 2017). Additionly, the MagCSs had a potential of angiogenesis in ischemic tissues that were attributable to an increased expression of vascular endothelial growth factor and reduced apoptosis in ischemic tissues (Ishii et al., 2011). Besides, Zhang et al. (2017) reported that multilayered cell sheets incorporated with growth factors via MAM could induce bone formation. Furthermore, they also successfully constructed a composite tissue (two-layer chondrogenic and osteogenic cell sheets) using MAM to mimic an integrated osteochondral complex with a cartilage-bone junction, and cells in respective sheets were observed to differentiate into chondrocytes and osteoblasts, respectively. If used more effectively, MagCSs, free from the restriction of scaffolds, could match the thickness and the shape of cartilage lesion, and mimic the natural composition of cartilage tissue, which could be further used in cartilage repair.
The Effects of MAM In Vivo in Cartilage Regeneration
The first detailed in vivo study of MAM was reported by Kobayashi et al. (2008), who evaluated its efficacy and safety in animal models with osteochondral defects. They injected 5 × 106 m-MSCs and accumulated to the cartilage defect with an external magnetic force or 10 min. The process of accumulation were observed via arthroscopic evaluation. At 12 weeks after surgery, the arthroscopic scores and histological scores (Wakitani scoring system) were significantly better in the MAM group than in the MSC group and control group. Besides, Kamei et al. (2013) reported the efficacy of MTD in 16 mini-pig cartilage defect models, and found that the adhesion rate of m-MSCs transplanted to the cartilage defect site was increased by MTD, compared to the case treated with the gravity adhesion technique. Moreover, transplanted m-MSCs still retained their chondrogenic potential. And Mahmoud et al. (2016) who evaluated 26 white rabbits treated with MTD showed efficient cartilage repair even in a case of severe chronic osteochondral defect. In addition, Hori et al. (2011) demonstrated that transplant magnetic-synovium-derived cells (m-SDCs) to the defect site have chondrogenic potential with thick layers of chondrocyte-like cells in the defects and better Wakitani scale and modified O’Driscoll scale at 12 weeks after surgery. And Kotaka et al. (2017) transplanted the magnetically labeled pluripotent stem cells (m-iPS) into the osteochondral defect site of a nude rate in the presence of MF, and indicated that MTD of m-iPS could improve the cartilage regeneration. For safety evaluation after injection of m-MSCs into the knee joint, assessments of in vivo kinetics of transplanted m-MSCs revealed that m-MSCs were not present in any major organs (brain, heart, lungs, liver, kidneys, spleen) after intraarticular administration, regardless of magnetic targeting or not (Ikuta et al., 2015).
The Application of MAM in Clinical Trial
However, only one study reported MAM applicated in clinical cartilage repair. Kamei et al. (2018) evaluated five patients treated with MAM at 48 weeks follow-up. The average defect size was 2 cm2 area. Exposing to a 1.0 T MF, 1 × 107 m-MSCs containing in 5 ml of saline were injected into the knee joint. After 48 weeks follow-up, their data showed that all five patients were satisfied with the clinical outcomes, reporting a significant improvement of IKDC and KOOS score. MRI and arthroscopy finding showed complete coverage of the defect sites in three patients. Swelling of treated knee joint occurred in three patients, two of who resolved within 2 weeks, but one still remained at 48 weeks. No other adverse events were observed during treatment.
Conclusion and Further Perspectives
Benefiting from the advances in magnetically actuated manipulation for cartilage regeneration has attracted increasing interests. The current state-of-art research indicating the great potential of magnetic strategies for cartilage tissue engineering. Different strategies of magnetically actuated manipulation were summarized into the following aspects: MTD could deliver m-cells to the targeted cartilage defect sites with high adhesion rate; MSS could condense m-cells into the scaffold with magnetic confinement; magnetic scaffold could provide superior structure and mechanical performance for cell adhesion and proliferation; and MagCSs, with multilayer cell sheets or containing different cell types, could match the thickness and the shape of cartilage lesion without any restriction of scaffolds.
However, there are still several challenges to be addressed before its further application. In future, it should answer the following questions: what is the influence of dispersed or localized form of MNPs in conjunction with a MF on the intracellular modulation; what is the mechanisms of chondrogenic differentiation after cell targeting, magnetic seeding or MagCSs formation, whether it has similar outcomes as those of ACI or other one-step procedures, and whether it could relieve pain and shorten the rehabilitation process. To date, in vivo studies reveal the potential of MAM to stimulate chondrocyte-related gene expression and to promote chondrocyte-like cells regeneration. However, the ability to mimic or regenerate native extracellular matrix, which is essential for cartilage repair, should be further researched.
Author Contributions
CZ, Y-ZC, X-JL, and YW conceived the study. CZ and Y-ZC wrote the manuscript. All authors contributed to the article and approved the submitted version.
Funding
This work was supported by Natural Science Foundation of China (81902225), Zhejiang Provincial Natural Science Foundation of China (LQ18H060002), and Zhejiang Medical and Health Science and Technology Project (2019KY384).
Conflict of Interest
The authors declare that the research was conducted in the absence of any commercial or financial relationships that could be construed as a potential conflict of interest.
References
Agung, M., Ochi, M., Yanada, S., Adachi, N., Izuta, Y., Yamasaki, T., et al. (2006). Mobilization of bone marrow-derived mesenchymal stem cells into the injured tissues after intraarticular injection and their contribution to tissue regeneration. Knee Surg. Sports Traumatol. Arthrosc. 14, 1307–1314. doi: 10.1007/s00167-006-0124-8
Arias, S. L., Shetty, A., Devorkin, J., and Allain, J. P. (2018). Magnetic targeting of smooth muscle cells in vitro using a magnetic bacterial cellulose to improve cell retention in tissue-engineering vascular grafts. Acta Biomater. 77, 172–181. doi: 10.1016/j.actbio.2018.07.013
Bakhru, S. H., Altiok, E., Highley, C., Delubac, D., Suhan, J., Hitchens, T. K., et al. (2012). Enhanced cellular uptake and long-term retention of chitosan-modified iron-oxide nanoparticles for MRI-based cell tracking. Int. J. Nanomedicine 7, 4613–4623. doi: 10.2147/ijn.s28294
Bonnier, F., Keating, M. E., Wrobel, T. P., Majzner, K., Baranska, M., Garcia-Munoz, A., et al. (2015). Cell viability assessment using the Alamar blue assay: a comparison of 2D and 3D cell culture models. Toxicol. In Vitro 29, 124–131. doi: 10.1016/j.tiv.2014.09.014
Brady, M. A., Talvard, L., Vella, A., and Ethier, C. R. (2017). Bio-inspired design of a magnetically active trilayered scaffold for cartilage tissue engineering. J. Tissue Eng. Regen. Med. 11, 1298–1302. doi: 10.1002/term.2106
Bulte, J. W. (2009). In vivo MRI cell tracking: clinical studies. AJR Am. J. Roentgenol. 193, 314–325. doi: 10.2214/ajr.09.3107
Caplan, A. I. (2007). Adult mesenchymal stem cells for tissue engineering versus regenerative medicine. J. Cell Physiol. 213, 341–347. doi: 10.1002/jcp.21200
Charlton, J. R., Pearl, V. M., Denotti, A. R., Lee, J. B., Swaminathan, S., Scindia, Y. M., et al. (2016). Biocompatibility of ferritin-based nanoparticles as targeted MRI contrast agents. Nanomedicine 12, 1735–1745. doi: 10.1016/j.nano.2016.03.007
Delyagina, E., Li, W., Ma, N., and Steinhoff, G. (2011). Magnetic targeting strategies in gene delivery. Nanomedicine 6, 1593–1604. doi: 10.2217/nnm.11.143
Enea, D., Cecconi, S., Calcagno, S., Busilacchi, A., Manzotti, S., and Gigante, A. (2015). One-step cartilage repair in the knee: collagen-covered microfracture and autologous bone marrow concentrate. A pilot study. Knee 22, 30–35. doi: 10.1016/j.knee.2014.10.003
Fayol, D., Frasca, G., Le Visage, C., Gazeau, F., Luciani, N., and Wilhelm, C. (2013). Use of magnetic forces to promote stem cell aggregation during differentiation, and cartilage tissue modeling. Adv. Mater. 25, 2611–2616. doi: 10.1002/adma.201300342
Ghosh, S., Kumar, S. R., Puri, I. K., and Elankumaran, S. (2016). Magnetic assembly of 3D cell clusters: visualizing the formation of an engineered tissue. Cell Prolif. 49, 134–144. doi: 10.1111/cpr.12234
Gil, S., Correia, C. R., and Mano, J. F. (2015). Magnetically labeled cells with surface-modified fe3 o4 spherical and rod-shaped magnetic nanoparticles for tissue engineering applications. Adv. Healthc. Mater. 4, 883–891. doi: 10.1002/adhm.201400611
Gobbi, A., and Whyte, G. P. (2016). One-stage cartilage repair using a hyaluronic acid-based scaffold with activated bone marrow-derived mesenchymal stem cells compared with microfracture: five-year follow-up. Am. J. Sports Med. 44, 2846–2854. doi: 10.1177/0363546516656179
Goncalves, A. I., Rodrigues, M. T., and Gomes, M. E. (2017). Tissue-engineered magnetic cell sheet patches for advanced strategies in tendon regeneration. Acta Biomater. 63, 110–122. doi: 10.1016/j.actbio.2017.09.014
Guillotin, B., and Guillemot, F. (2011). Cell patterning technologies for organotypic tissue fabrication. Trends Biotechnol. 29, 183–190. doi: 10.1016/j.tibtech.2010.12.008
Hori, J., Deie, M., Kobayashi, T., Yasunaga, Y., Kawamata, S., and Ochi, M. (2011). Articular cartilage repair using an intra-articular magnet and synovium-derived cells. J. Orthop. Res. 29, 531–538. doi: 10.1002/jor.21267
Hsieh, C. H., Lee, M. C., Tsai-Wu, J. J., Chen, M. H., Lee, H. S., Chiang, H., et al. (2008). Deleterious effects of MRI on chondrocytes. Osteoarthr. Cartil. 16, 343–351. doi: 10.1016/j.joca.2007.07.001
Hu, S., Zhou, Y., Zhao, Y., Xu, Y., Zhang, F., Gu, N., et al. (2018). Enhanced bone regeneration and visual monitoring via superparamagnetic iron oxide nanoparticle scaffold in rats. J. Tissue Eng. Regen. Med. 12, e2085–e2098. doi: 10.1002/term.2641
Huang, D. M., Hsiao, J. K., Chen, Y. C., Chien, L. Y., Yao, M., Chen, Y. K., et al. (2009). The promotion of human mesenchymal stem cell proliferation by superparamagnetic iron oxide nanoparticles. Biomaterials 30, 3645–3651. doi: 10.1016/j.biomaterials.2009.03.032
Huang, J., Liang, Y., Jia, Z., Chen, J., Duan, L., Liu, W., et al. (2018). Development of magnetic nanocomposite hydrogel with potential cartilage tissue engineering. ACS Omega 3, 6182–6189. doi: 10.1021/acsomega.8b00291
Ikuta, Y., Kamei, N., Ishikawa, M., Adachi, N., and Ochi, M. (2015). In vivo kinetics of mesenchymal stem cells transplanted into the knee joint in a rat model using a novel magnetic method of localization. Clin. Transl. Sci. 8, 467–474. doi: 10.1111/cts.12284
Ishii, M., Shibata, R., Numaguchi, Y., Kito, T., Suzuki, H., Shimizu, K., et al. (2011). Enhanced angiogenesis by transplantation of mesenchymal stem cell sheet created by a novel magnetic tissue engineering method. Arterioscler Thromb. Vasc. Biol. 31, 2210–2215. doi: 10.1161/atvbaha.111.231100
Ito, A., Jitsunobu, H., Kawabe, Y., and Kamihira, M. (2007). Construction of heterotypic cell sheets by magnetic force-based 3-D coculture of HepG2 and NIH3T3 cells. J. Biosci. Bioeng. 104, 371–378. doi: 10.1263/jbb.104.371
Jaberi, F. M., Keshtgar, S., Tavakkoli, A., Pishva, E., Geramizadeh, B., Tanideh, N., et al. (2011). A moderate-intensity static magnetic field enhances repair of cartilage damage in rabbits. Arch. Med. Res. 42, 268–273. doi: 10.1016/j.arcmed.2011.06.004
Kamei, G., Kobayashi, T., Ohkawa, S., Kongcharoensombat, W., Adachi, N., Takazawa, K., et al. (2013). Articular cartilage repair with magnetic mesenchymal stem cells. Am. J. Sports Med. 41, 1255–1264. doi: 10.1177/0363546513483270
Kamei, N., Ochi, M., Adachi, N., Ishikawa, M., Yanada, S., Levin, L. S., et al. (2018). The safety and efficacy of magnetic targeting using autologous mesenchymal stem cells for cartilage repair. Knee Surg. Sports Traumatol. Arthrosc. 26, 3626–3635. doi: 10.1007/s00167-018-4898-2
Kang, H., Jung, H. J., Wong, D. S. H., Kim, S. K., Lin, S., Chan, K. F., et al. (2018). Remote control of heterodimeric magnetic nanoswitch regulates the adhesion and differentiation of stem cells. J. Am. Chem. Soc. 140, 5909–5913. doi: 10.1021/jacs.8b03001
Kim, J., and Tanner, K. (2016). Three-dimensional patterning of the ECM microenvironment using magnetic nanoparticle self assembly. Curr. Protoc. Cell Biol. 70, 25.3.1–25.3.14. doi: 10.1002/0471143030.cb2503s70
Kobayashi, T., Ochi, M., Yanada, S., Ishikawa, M., Adachi, N., Deie, M., et al. (2008). A novel cell delivery system using magnetically labeled mesenchymal stem cells and an external magnetic device for clinical cartilage repair. Arthroscopy 24, 69–76. doi: 10.1016/j.arthro.2007.08.017
Kotaka, S., Wakitani, S., Shimamoto, A., Kamei, N., Sawa, M., Adachi, N., et al. (2017). Magnetic targeted delivery of induced pluripotent stem cells promotes articular cartilage repair. Stem Cells Int. 2017:9514719. doi: 10.1155/2017/9514719
Koto, W., Shinohara, Y., Kitamura, K., Wachi, T., Makihira, S., and Koyano, K. (2017). Porcine dental epithelial cells differentiated in a cell sheet constructed by magnetic nanotechnology. Nanomaterials 7:322. doi: 10.3390/nano7100322
Li, L., Jiang, L.-L., Zeng, Y., and Liu, G. (2013). Toxicity of superparamagnetic iron oxide nanoparticles: research strategies and implications for nanomedicine. Chinese Phys. B 22:127503. doi: 10.1088/1674-1056/22/12/127503
Luciani, N., Du, V., Gazeau, F., Richert, A., Letourneur, D., Le Visage, C., et al. (2016). Successful chondrogenesis within scaffolds, using magnetic stem cell confinement and bioreactor maturation. Acta Biomater. 37, 101–110. doi: 10.1016/j.actbio.2016.04.009
Mahmoud, E. E., Kamei, G., Harada, Y., Shimizu, R., Kamei, N., Adachi, N., et al. (2016). Cell magnetic targeting system for repair of severe chronic osteochondral defect in a rabbit model. Cell Transpl. 25, 1073–1083. doi: 10.3727/096368915x689613
Margolis, G., Polyak, B., and Cohen, S. (2018). Magnetic induction of multiscale anisotropy in macroporous alginate scaffolds. Nano Lett. 18, 7314–7322. doi: 10.1021/acs.nanolett.8b03514
Mejias, R., Gutierrez, L., Salas, G., Perez-Yague, S., Zotes, T. M., Lazaro, F. J., et al. (2013). Long term biotransformation and toxicity of dimercaptosuccinic acid-coated magnetic nanoparticles support their use in biomedical applications. J. Control Release 171, 225–233. doi: 10.1016/j.jconrel.2013.07.019
Melchels, F. P., Barradas, A. M., van Blitterswijk, C. A., de Boer, J., Feijen, J., and Grijpma, D. W. (2010). Effects of the architecture of tissue engineering scaffolds on cell seeding and culturing. Acta Biomater. 6, 4208–4217. doi: 10.1016/j.actbio.2010.06.012
Miyakoshi, J. (2005). Effects of static magnetic fields at the cellular level. Prog. Biophys. Mol. Biol. 87, 213–223. doi: 10.1016/j.pbiomolbio.2004.08.008
Mohanty, S., Jain, K. G., Nandy, S. B., Kakkar, A., Kumar, M., Dinda, A. K., et al. (2018). Iron oxide labeling does not affect differentiation potential of human bone marrow mesenchymal stem cells exhibited by their differentiation into cardiac and neuronal cells. Mol. Cell. Biochem. 448, 17–26. doi: 10.1007/s11010-018-3309-9
Nakamae, T., Adachi, N., Kobayashi, T., Nagata, Y., Nakasa, T., Tanaka, N., et al. (2010). The effect of an external magnetic force on cell adhesion and proliferation of magnetically labeled mesenchymal stem cells. Sports Med. Arthrosc. Rehabil. Ther. Technol. 2:5. doi: 10.1186/1758-2555-2-5
Ochi, M., Nakasa, T., Kamei, G., Usman, M. A., and Mahmoud, E. (2014). Regenerative medicine in orthopedics using cells, scaffold, and microRNA. J. Orthop. Sci. 19, 521–528. doi: 10.1007/s00776-014-0575-6
Penland, N., Choi, E., Perla, M., Park, J., and Kim, D. H. (2017). Facile fabrication of tissue-engineered constructs using nanopatterned cell sheets and magnetic levitation. Nanotechnology 28:075103. doi: 10.1088/1361-6528/aa55e0
Rauch, J., Kolch, W., Laurent, S., and Mahmoudi, M. (2013). Big signals from small particles: regulation of cell signaling pathways by nanoparticles. Chem. Rev. 113, 3391–3406. doi: 10.1021/cr3002627
Ruiz, A., Mancebo, A., Beola, L., Sosa, I., and Gutierrez, L. (2016). Dose–response bioconversion and toxicity analysis of magnetite nanoparticles. IEEE Magn. Lett. 7, 1–5. doi: 10.1109/lmag.2016.2535414
Santos, L., Silva, M., Goncalves, A. I., Pesqueira, T., Rodrigues, M. T., and Gomes, M. E. (2016). In vitro and in vivo assessment of magnetically actuated biomaterials and prospects in tendon healing. Nanomedicine 11, 1107–1122. doi: 10.2217/nnm-2015-0014
Santos, L. J., Reis, R. L., and Gomes, M. E. (2015). Harnessing magnetic-mechano actuation in regenerative medicine and tissue engineering. Trends Biotechnol. 33, 471–479. doi: 10.1016/j.tibtech.2015.06.006
Sensenig, R., Sapir, Y., MacDonald, C., Cohen, S., and Polyak, B. (2012). Magnetic nanoparticle-based approaches to locally target therapy and enhance tissue regeneration in vivo. Nanomedicine 7, 1425–1442. doi: 10.2217/nnm.12.109
Sharifi, S., Behzadi, S., Laurent, S., Forrest, M. L., Stroeve, P., and Mahmoudi, M. (2012). Toxicity of nanomaterials. Chem. Soc. Rev. 41, 2323–2343. doi: 10.1039/c1cs15188f
Shimizu, K., Ito, A., and Honda, H. (2006). Enhanced cell-seeding into 3D porous scaffolds by use of magnetite nanoparticles. J. Biomed. Mater. Res. B Appl. Biomater. 77, 265–272. doi: 10.1002/jbm.b.30443
Son, B., Kim, H. D., Kim, M., Kim, J. A., Lee, J., Shin, H., et al. (2015). Physical stimuli-induced chondrogenic differentiation of mesenchymal stem cells using magnetic nanoparticles. Adv. Healthc. Mater. 4, 1339–1347. doi: 10.1002/adhm.201400835
Son, Y., Cheong, Y. K., Kim, N. H., Chung, H. T., Kang, D. G., and Pae, H. O. (2011). Mitogen-activated protein kinases and reactive oxygen species: how can ROS activate MAPK pathways? J. Signal. Transduct. 2011:792639. doi: 10.1155/2011/792639
Souza, G. R., Molina, J. R., Raphael, R. M., Ozawa, M. G., Stark, D. J., Levin, C. S., et al. (2010). Three-dimensional tissue culture based on magnetic cell levitation. Nat. Nanotechnol. 5, 291–296. doi: 10.1038/nnano.2010.23
Su, J. Y., Chen, S. H., Chen, Y. P., and Chen, W. C. (2017). Evaluation of magnetic nanoparticle-labeled chondrocytes cultivated on a type II collagen-Chitosan/Poly(Lactic-co-Glycolic) acid biphasic scaffold. Int. J. Mol. Sci. 18:87. doi: 10.3390/ijms18010087
Thevenot, P., Sohaebuddin, S., Poudyal, N., Liu, J. P., and Tang, L. (2008). Magnetic nanoparticles to enhance cell seeding and distribution in tissue engineering scaffolds. Proc. IEEE Conf. Nanotechnol. 2008, 646–649. doi: 10.1109/nano.2008.196
van Buul, G. M., Kotek, G., Wielopolski, P. A., Farrell, E., Bos, P. K., Weinans, H., et al. (2011). Clinically translatable cell tracking and quantification by MRI in cartilage repair using superparamagnetic iron oxides. PLoS One 6:e17001. doi: 10.1371/journal.pone.0017001
Vidiasheva, I. V., Abalymov, A. A., Kurochkin, M. A., Mayorova, O. A., Lomova, M. V., German, S. V., et al. (2018). Transfer of cells with uptaken nanocomposite, magnetite-nanoparticle functionalized capsules with electromagnetic tweezers. Biomater. Sci. 6, 2219–2229. doi: 10.1039/c8bm00479j
Wang, Q., Chen, B., Cao, M., Sun, J., Wu, H., Zhao, P., et al. (2016). Response of MAPK pathway to iron oxide nanoparticles in vitro treatment promotes osteogenic differentiation of hBMSCs. Biomaterials 86, 11–20. doi: 10.1016/j.biomaterials.2016.02.004
Wong, D. S., Li, J., Yan, X., Wang, B., Li, R., Zhang, L., et al. (2017). Magnetically tuning tether mobility of integrin ligand regulates adhesion, spreading, and differentiation of stem cells. Nano Lett. 17, 1685–1695. doi: 10.1021/acs.nanolett.6b04958
Xia, Y., Sun, J., Zhao, L., Zhang, F., Liang, X.-J., Guo, Y., et al. (2018). Magnetic field and nano-scaffolds with stem cells to enhance bone regeneration. Biomaterials 183, 151–170. doi: 10.1016/j.biomaterials.2018.08.040
Xu, J., Feng, Q., Lin, S., Yuan, W., Li, R., Li, J., et al. (2019). Injectable stem cell-laden supramolecular hydrogels enhance in situ osteochondral regeneration via the sustained co-delivery of hydrophilic and hydrophobic chondrogenic molecules. Biomaterials 210, 51–61. doi: 10.1016/j.biomaterials.2019.04.031
Zhang, C., Cai, Y. Z., and Lin, X. J. (2016). Autologous chondrocyte implantation: is it likely to become a saviour of large-sized and full-thickness cartilage defect in young adult knee? Knee Surg. Sports Traumatol. Arthrosc. 24, 1643–1650. doi: 10.1007/s00167-015-3643-3
Zhang, J., Ding, C., Ren, L., Zhou, Y., and Shang, P. (2014). The effects of static magnetic fields on bone. Prog. Biophys. Mol. Biol. 114, 146–152. doi: 10.1016/j.pbiomolbio.2014.02.001
Zhang, N., Lock, J., Sallee, A., and Liu, H. (2015). Magnetic nanocomposite hydrogel for potential cartilage tissue engineering: synthesis, characterization, and cytocompatibility with bone marrow derived mesenchymal stem cells. ACS Appl. Mater. Interfaces 7, 20987–20998. doi: 10.1021/acsami.5b06939
Zhang, W., Yang, G., Wang, X., Jiang, L., Jiang, F., Li, G., et al. (2017). Magnetically controlled growth-factor-immobilized multilayer cell sheets for complex tissue regeneration. Adv. Mater. 29:1703795. doi: 10.1002/adma.201703795
Keywords: magnetically actuated manipulation, magnetic nanoparticles, magnetic seeding, magnetic scaffolds, magnetic cell sheets, cartilage regeneration
Citation: Zhang C, Cai Y-Z, Lin X-J and Wang Y (2020) Magnetically Actuated Manipulation and Its Applications for Cartilage Defects: Characteristics and Advanced Therapeutic Strategies. Front. Cell Dev. Biol. 8:526. doi: 10.3389/fcell.2020.00526
Received: 09 March 2020; Accepted: 03 June 2020;
Published: 30 June 2020.
Edited by:
Claudia Tanja Mierke, Leipzig University, GermanyReviewed by:
Liming Bian, The Chinese University of Hong Kong, ChinaGang Li, The Chinese University of Hong Kong, China
Copyright © 2020 Zhang, Cai, Lin and Wang. This is an open-access article distributed under the terms of the Creative Commons Attribution License (CC BY). The use, distribution or reproduction in other forums is permitted, provided the original author(s) and the copyright owner(s) are credited and that the original publication in this journal is cited, in accordance with accepted academic practice. No use, distribution or reproduction is permitted which does not comply with these terms.
*Correspondence: You-Zhi Cai, caiyouzhi@zju.edu.cn; Yue Wang, wangyuespine@zju.edu.cn