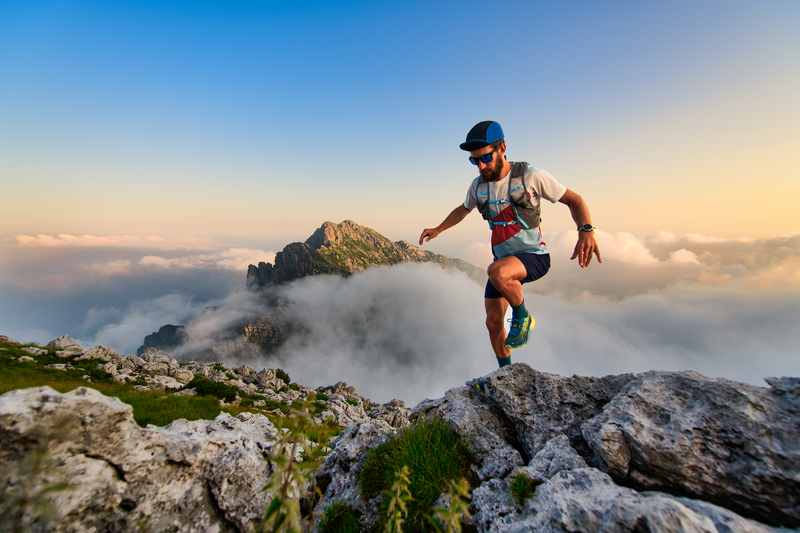
94% of researchers rate our articles as excellent or good
Learn more about the work of our research integrity team to safeguard the quality of each article we publish.
Find out more
ORIGINAL RESEARCH article
Front. Cell Dev. Biol. , 23 June 2020
Sec. Molecular and Cellular Oncology
Volume 8 - 2020 | https://doi.org/10.3389/fcell.2020.00504
Focused ultrasound (FUS) is a rapidly developing stimulus technology with the potential to uncover novel mechanosensory dependent cellular processes. Since it is non-invasive, it holds great promise for future therapeutic applications in patients used either alone or as a complement to boost existing treatments. For example, FUS stimulation causes invasive but not non-invasive cancer cell lines to exhibit marked activation of calcium signaling pathways. Here, we identify the membrane channel PANNEXIN1 (PANX1) as a mediator for activation of calcium signaling in invasive cancer cells. Knockdown of PANX1 decreases calcium signaling in invasive cells, while PANX1 overexpression enhances calcium elevations in non-invasive cancer cells. We demonstrate that FUS may directly stimulate mechanosensory PANX1 localized in endoplasmic reticulum to evoke calcium release from internal stores. This process does not depend on mechanosensory stimulus transduction through an intact cytoskeleton and does not depend on plasma membrane localized PANX1. Plasma membrane localized PANX1, however, plays a different role in mediating the spread of intercellular calcium waves via ATP release. Additionally, we show that FUS stimulation evokes cytokine/chemokine release from invasive cancer cells, suggesting that FUS could be an important new adjuvant treatment to improve cancer immunotherapy.
Cancer cell invasion and tumor metastasis play a critical role in cancer mortality. The mechanisms by which malignant tumors leave the primary tumor site, invade, and metastasize to other organs are complex, interrelated and only partially understood. Calcium signaling, however is known to be critical in these processes. To develop a functional assay of cancer cell invasion potential, we recently used focused ultrasound (FUS) stimulation to probe the altered calcium signaling pathways exhibited by invasive cancer cells. FUS stimulation caused invasive, but not non-invasive cancer cell lines, to exhibit marked calcium signaling suggesting a novel means to determine the invasion potential. We validated this using a Matrigel invasion assay, demonstrating that the degree of invasion correlates well with the degree of FUS-dependent Ca2+ signaling (Hwang et al., 2013; Weitz et al., 2017). FUS stimuli evoke widespread Ca2+ oscillatory dynamics in several invasive cancer cell lines (breast MDA-MB-231, prostate PC-3 and bladder T24/83), but not in non-invasive cells of the same cancer type (MCF-7, BPH-1, and RT112/84) suggesting that this is a general property of invasive cells (Hwang et al., 2013; Weitz et al., 2017). Also, different FUS stimulation frequencies result in similar responses indicating that Ca2+ signaling is independent of stimulation frequency (3-, 38-, or 200-MHz).
Focused ultrasound stimulation of invasive cells also results in a time dependent propagation of an extracellular calcium wave spreading away from cells located at the transducer focus. The mechanism(s) for extracellular calcium wave propagation is unclear, it does not depend on ultrasonic surface waves or gap junctions (Weitz et al., 2017). Additional pharmacological studies in invasive cancer cells suggested the involvement of IP3 receptors (IP3Rs) or TRP channels (Weitz et al., 2017), as suggested by others (Diver et al., 2001; Bootman et al., 2002; Xu et al., 2005). Non-focused US also stimulates calcium signaling mediated by the Piezo1 mechanosensitive ion channel directly coupled to microbubbles (Pan et al., 2018). Other ER-localized mechanosensitive channels (i.e., Msy1 and Msy in fission yeast) regulate intracellular Ca2+ and cell volume for survival upon hypo-osmotic shock (Nakayama et al., 2012).
Focused ultrasound technology has also been proposed for use in cancer therapy and particularly immunotherapy. High-intensity (>5 W/cm2) continuous FUS generates a systemic immune stimulatory effect resulting in tumor ablation (Lu et al., 2009). Pulsed FUS (i.e., non-continuous stimulus to minimize heat generation) (Hersh et al., 2016) may induce a more refined cellular/molecular immune response (Ziadloo et al., 2012) by initiating inflammatory responses which boost cancer immunotherapy (Curley et al., 2017; Mauri et al., 2018). FUS may thus offer a new approach to overcome cancer immune-resistance, a well-known limitation preventing more wide-spread clinical adoption of successful immunotherapies such as CAR T cells (Caliendo et al., 2019; Tokarew et al., 2019). A detailed understanding of the mechanistic aspects of FUS-response mechanisms however is currently lacking.
In this study we establish a new role for the mechanosensitive PANX1 hemichannel (Bao et al., 2004) in mediating Ca2+ signaling in invasive cancer cells. PANX1 localizes to both plasma membrane (PM) as well as endoplasmic reticulum (ER) (Vanden Abeele et al., 2006). Mechano-sensitive responses have previously been described in neurons, including retinal ganglion cells (Xia et al., 2012) and other cell types. Our results suggest that FUS can directly stimulate ER localized PANX1 in invasive PC-3 cancer cells to generate Ca2+ release from intracellular ER stores, independently of extracellular Ca2+ entry. This is a newly described role of PANX1 as a regulator of calcium ion exchange between the ER and cytoplasm, suggesting a new working model of how FUS interacts with cancer cells to initiate and propagate Ca2+ signaling. In addition, our results suggest that continued development of FUS technology could provide not only a new way to probe mechanosensitive functions of signaling pathways located in specific intracellular compartments but also to harness the potential to regulate adjunct immune cell responses through the coupling of mechanosensory stimulus to chemokine/cytokine release profiles.
PC-3 prostate cancer lines and HEK 293T cells were used in this study. PC-3 cells were purchased from ATCC and HEK 293T cells obtained from Dr. Fabien Pinaud at University of Southern California. Both cells were cultured in DMEM. The medium was supplemented with 10% FBS and 2 mM L-glutamine, and Penicillin/Streptomycin. All cell lines were tested to be free of mycoplasma contamination using a mycoplasma PCR detection kit (Sigma). Cell lines were authenticated using short tandem repeat (STR) analysis by the University of Arizona Genetics Core. PC-3 cells are highly invasive cell lines, while HEK cells were used as non-invasive because of poor transfection efficiency of BPH-1.
Cells were plated on 35-mm culture dishes, or 24-well culture plates to a density of 106 or 105 cells per dish or well. All cells were stained with cell membrane permeant Fluo-4 AM (Thermo Fisher Scientific), a fluorescent reporter of intracellular calcium activity. Staining was performed by incubating dishes/wells with 1 μM Fluo-4 AM for 30 min immediately prior to imaging. Following calcium dye loading, cells were washed with and maintained in external buffer solution consisting of 140 mM NaCl, 2.8 mM KCl, 1 mM MgCl2, 2 mM CaCl2, 10 mM HEPES, and 10 mM D-glucose, adjusted to pH 7.3 and 290–300 mOsm.
Lipofectamine 3000 (Invitrogen) was used for cDNA construct transfection experiments. cDNA constructs used are WT PANX1-EGFP and mt PANX1-mRFP that are provided by Dr. Tavazoie (Furlow et al., 2015). FL WT PANX1 with no EGFP fusion (WT PANX1) was made for Fluo-4 AM Ca2+ detection, after deletion of EGFP using a standard sub-cloning method. For siRNA transfection, we used DharmaFect (Thermo Scientific) according to the manufacturer’s instructions. siRNA specifically designed to target FL PANX1 (si-PANX1) was purchased from Dharmacon (GE Healthcare) (D-018253-02) (Supplementary Figure S5). Control siRNA (negative) was obtained from GE Healthcare (D-001810-10).
Expression of mRNA was quantified by qRT-PCR as described previously (Lee et al., 2015). Primers are the followings: for PANX1, forward 5′-agcccacggagcccaagttca and reverse 5′-gcgcgaaggccagcgaga, for GAPDH and CyclophilinA, they are described in our previous paper (Lee et al., 2015).
A single-element, lithium niobite (LiNbO3), press-focused 46 MHz (f-number = 2, focal length = 6 mm) transducer was fabricated in house as described previously (Lam et al., 2013) and used in most experiments. In addition, a PZT, pressed-focused 3-MHz transducer (f-number = 1.5, focal length = 4 mm) was also tested. To drive the transducers, sinusoidal bursts from a signal generator (SG382; Stanford Research Systems) were fed to a 50-dB power amplifier (525LA; Electronics & Innovation) whose output was used to excite the transducer. For the 46-MHz transducer, amplitude was tested at different input Vp–p, pulse repetition frequency (PRF) at 1 kHz, and duty cycle at 5%, in PC-3 and HEK cells (Figures 1E–G, Supplementary Figure S2). The acoustic output of the 46-MHz transducer was measured with a needle hydrophone (HGL-0085; Onda). Figure 1C shows the measured beam width of the focused ultrasound to be ∼70 mm, which may focus on ∼6 cells. Using the standard cell stimulation parameters provided above [12 Vp-p (40 mV) amplitude, 1 kHz PRF, and 5% duty cycle], the intensity and pressure at the focus were measured by the hydrophone.
Figure 1. Schematic of our experimental system and effect of FUS stimulation amplitude on PC-3 Ca2+ response. (A) A 46-MHz, single-element, LiNbO3, press-focused transducer was used for FUS stimulation and focused with a pulse-echo receiver. Cells were imaged using epi-florescence microscopy in the presence of a Ca2+ indicator. (B) Photograph of the 46-MHz transducer used in most experiments. (C) The beam width produced by the transducer was measured by hydrophone and was ∼70 μm. (D) Typical voltage waveform used to drive the transducer. Carrier frequency had an amplitude of 46 MHz (12 Vp–p), pulse repetition frequency was 1 kHz and duty cycle was 5%. (E,F) Effect of FUS stimulation amplitude on PC-3 Ca2+ response. Standard stimulus parameters (D) were used while varying the transducer input voltage. All stated voltages represent peak-to-peak amplitude (Vp-p). Values in parentheses indicate the mV and Ispta at each voltage, as measured by a hydrophone. (E) 2-D histograms showing the percentage of responding cells over time. (F) Scatter plots showing the time at which each individual cell first responds. (G) Quantitative percentage of responding cells. n > 3 biological replicates. Error bars, SEM., *P < 0.05; **P < 0.01; ***P < 0.001 by a one-tailed t-test. n represents biological replicates.
A custom microscope system was used to image cellular fluorescence while performing simultaneous ultrasonic stimulation as described previously (Hwang et al., 2013; Weitz et al., 2017). Petri dishes or plates containing cells were placed on the stage of an inverted epifluorescence microscope (Olympus IX70), and the ultrasound transducer was lowered into the external buffer solution. A motorized three-axis micromanipulator was used to position the transducer in focus with the cell monolayer. In each experiment, live-cell fluorescence imaging was performed for 240 s (and sometimes, 300 s), with the ultrasound stimulus being delivered continuously between t = 50 and 200 s. Excitation light was provided by a mercury arc lamp and filtered through an excitation bandpass filter (488 ± 20 nm). Fluorescence emitted from the calcium dye was filtered through an emission bandpass filter (530 ± 20 nm) and recorded at 1 Hz (30% exposure duty cycle) with a digital CMOS camera (ORCA-Flash2.8; Hamamatsu). All imaging was performed at 4× magnification in order to capture activity from hundreds or thousands of cells simultaneously. For each cell line, simulation and imaging experiments were replicated in at least two different dishes of cells, and over least three independent fields of view per dish. Experiments involving pharmacological blockers were limited to a single field of view per dish. Figures show representative data obtained from one field of view.
Data were post-processed to determine the calcium response of every imaged cell as described previously (Weitz et al., 2017). Cell locations were identified automatically with CellProfiler image analysis software (Carpenter et al., 2006) and used to extract the raw fluorescence intensities of each cell. These intensities were exported to MATLAB (MathWorks) in order to calculate each cell’s normalized change in fluorescence (ΔF/F) during every imaging frame. Responding cells were defined as those that exhibited a ΔF/Fmax greater than 3.5 times the pre-stimulus root-mean-square noise level. Two types of plots were generated for each 240 s experiment: a histogram showing the percentage of responding cells over time and a scatter plot indicating the time at which each cell first responded to the stimulus. Responding cells in these plots were arranged with respect to their distance from the transducer focus. The cell response index (CRI) was obtained as described previously (Hwang et al., 2013).
To investigate the mechanism of ultrasound-induced calcium rise in invasive cancer cells, PC-3 cells were stimulated in the presence of various pharmacological agents. We tested several different blockers, each applied separately (Supplementary Table S1). Blockers were dissolved in the external buffer solution 15–30 min before performing imaging and ultrasound stimulation. Cellular responses were measured before adding the blockers and in the presence of blockers.
Cells were seeded in quadruplicate at 100,000–200,000 cells per well in 24-well plates and grown overnight. Each well was then washed with 1 ml external buffer solution (EBS). For PANX1 inhibition, cells were incubated at room temperature for 10 min in EBS supplemented with one of the following reagents: CBX (500 μM), probenecid (2 mM; Life Technologies), 10Panx1 (100 μM) or an equivalent dose of the appropriate vehicle control (EBS or scrambled peptide). The wash or pretreatment solution was then aspirated, replaced with 1 ml EBS for 10 min, collected and transferred to microcentrifuge tubes, and then spun at 86 g for 2 min at room temperature. 50 or 100 μl of supernatants was transferred to 96-well plates and ATP was measured using the CellTiter-Glo Luminescent Cell Viability Assay (Promega) according to the manufacturer’s instructions.
Cells expressing fluorescently tagged proteins were fixed in 4% paraformaldehyde, stained with DAPI (Thermo Fisher) and mounted using ProLong Gold antifade reagent (Thermo Fisher), and imaged using Leica TCS microscope.
TIRF microscopy images were acquired on an inverted Nikon Eclipse Ti-E microscope, equipped with a 100 × 1.49 NA objective (Nikon), an iXon EMCCD camera (Andor), laser lines at 405, 488, 561, and 647 nm (Agilent), a multiband pass ZET405/488/561/647× excitation filter (Chroma), a quad-band ZT405/488/561/647 dichroic mirror (Chroma), and appropriate emission filters for imaging of mRFP (600/50 nm, Chroma) and GFP (525/50 nm, Chroma). Illumination was performed by TIRF to ensure exclusive illumination of the plasma membrane.
For ICS, the cells are fixed with 100% methanol at −20°C for 10 min. After washing with PBS, they were permeabilized with 1% Triton X-100 at 37°C for 30 min. After blocking, add primary C-terminus anti-PANX1 (Santa Cruz Biotech.) or N-terminus anti-PANX1 (Alomone Labs) was added and incubated at 4°C at 12 h followed by addition of second anti-mouse-PE (Santa Cruz) at room temperature for 30 min. After washing, the cover slips containing cells were mounted and observed using a confocal microscope (Leica).
One day after FUS stimulation, cell culture supernates were collected and centrifuged. Seven hundred μl of supernates are applied for human XL cytokine array (R&D systems) according to the manufacturer’s instructions. Pixel densities on developed X-ray film were collected and analyzed using a transmission-mode scanner and image analysis software (Image Studio Lite).
In general results are expressed as mean ± s.e.m. Statistical analysis of multiple groups used one-way ANOVA, and Dunnet’s correction for multiple comparisons (GraphPad Prism, V8). Two group comparisons were tested using the Student’s t-test (one- and two-tailed) in Excel (v2016).
To further clarify the mechanism(s) of FUS-dependent Ca2+response, our usual stimulus protocol used a 46-MHz, single-element, LiNbO3, press-focused transducer focused via a pulse-echo receiver coupled with epi-fluorescence microscopy to assess both intra- and intercellular changes in Ca2+ dynamics (Figures 1A–D, see also section “Materials and Methods” for additional details). In our previous work we demonstrated that the magnitude of the FUS-induced Ca2+ response did not depend on the frequency of stimulation (Weitz et al., 2017). Here we examine its dependence on stimulus amplitude (i.e., intensity). Increasing voltage during FUS stimulation of PC-3 cells results in a larger Ca2+ response (i.e., a dose–response relationship as shown in Figures 1E–G). Standard stimulus parameters (see section “Materials and Methods,” Figure 1) were used while varying the transducer input voltage (Figures 1E,F). Stimulation at ∼1 Vp–p, 2.7 Vp-p, and 12 Vp-p evoked calcium activity in ∼20%, ∼50%, and >80% of cells, respectively (Figure 1G). We stimulated at 12 Vp-p for the remainder of this study, while keeping pulse repetition frequency (PRF) at 1 kHz and duty cycle at 5%. A 3-MHz stimulus was also effective in PC-3 cells (Supplementary Figure S1), reconfirming the independence of stimulus frequency in eliciting Ca2+ responses (Weitz et al., 2017).
Our previous studies (Weitz et al., 2017) suggested ER localized IP3 receptors or PM localized TRP channels were involved in mediating invasive cancer cell FUS-dependent Ca2+ responses.
Here we use PC-3 cells as a model of an invasive cancer cell type and compared FUS-dependent Ca2+ responses to those in a non-invasive HEK293 cell line. Non-responsive HEK cells were chosen as an appropriate control line in this study, rather than previously used BPH-1 cells, since they were much easier to transfect than BPH-1 (∼90% vs. <5% transfection efficiency). As expected, FUS stimulation evoked strong Ca2+ responses in PC-3 cells but not in non-invasive HEK cells (Figures 2A,B, Supplementary Figure S2, and Supplementary Videos S1, S2). In PC-3 cells, three distinct stimulus-dependent Ca2+ patterns are observed in individual cells in the presence of normal external Ca2+: Ca2+ oscillation, double Ca2+ spikes or a single spike (Figure 2C). We tested if PC-3 responses were mediated by Ca2+ influx by severely reducing or eliminating extracellular Ca2+ (0 or 20 μM vs. normal 2 mM). FUS stimulation in low or no external Ca2+ still exhibited Ca2+ response, but only a single spike pattern was observed, in PC-3 cells (Figure 2D, Bottom). We additionally investigated Ca2+ influx blockers to assess their effects on FUS-dependent Ca2+ dynamics. Surprisingly, treatment of PC- 3 cells with two different Ca2+ influx blockers (BTP2 or SKF96365) still showed all three patterns of Ca2+ response (Supplementary Figure S3 and Table 1) rather than the single spike when external Ca2+ is absent or low. This suggests that the specific route of Ca2+ entry may determine the specificity of subsequent response patterns. Notably, FUS stimulation in normal external Ca2+ (i.e., Ca2+ influx) following thapsigargin (TG) treatment completely abolished all Ca2+ responses (Figure 2E). TG is an agent that depletes intracellular Ca2+ stores. Our results indicate that external Ca2+ influx is not necessary for a FUS induced single spike Ca2+ response in PC-3 cells, suggest that this response is likely due to release from an internal storage site and differs from pharmacologically blocking 2 different PM Ca2+ channels. The mechanism of Ca2+ entry may thus play an important role in mediating complex Ca2+ dynamics following mechanosensory stimulation.
Figure 2. Ca2+ dynamics in invasive and non-invasive FUS stimulated cancer cells. (A) Background-subtracted fluorescence images show strong Ca2+ signaling in invasive PC-3 (right) but not non-invasive HEK (left) cells. (B) Top, 2-D histograms showing the percentage of responding cells over time. Vertical red and green dotted lines indicate FUS stimulus onset (50 s) and offset (200 s) times, respectively. Bottom, scatter plots showing the time of the first response in individual cells following stimulus. (C) Typical Ca2+ responses in invasive PC-3 cells exhibit either an oscillating (left), double (center) or single (right) spike pattern. (D) Ca2+ responses are present in PC-3 cells in external no or 20 μM (low) Ca2+ concentration. (E) Thapsigargin (TG) treatment in the normal external Ca2+ concentration (2 mM) drastically reduces the Ca2+ response.
To further investigate the complex pattern of Ca2+ signaling following FUS stimulation, we tested Ca2+ release from an internal storage site. IP3 Receptors are known to mediate Ca2+ release from ER or sarcoplasmic reticulum (SR) stores (Mery et al., 2005; Sasse et al., 2007; Rizaner et al., 2016). Treatment of cells with Xestospongin C (an IP3R inhibitor) partly inhibits the FUS-induced Ca2+ response (Figure 3). This suggests that other Ca2+ channels may also mediate release form internal stores. One potential candidate for such a role is PANX1. PM localized PANX1 is well studied for its role in ATP release but PANX1 is also localized to the ER where its function(s) is unknown (except for involvement in Ca2+ leaks, Vanden Abeele et al., 2006).
Figure 3. Treatment of PC-3 cells with 10PX1 (PANX1 inhibitor) abolishes the normal FUS-induced Ca2+ oscillation response but uncovers single Ca2+ transients. (A) Left column, the cells exhibited strong Ca2+ responses at 20 min after 200 μM scrambled peptide application as a control. Center column, cells were stimulated at 20 min after 200 μM 10Panx1 peptide (10PX1) application, and the responses were partly reduced. Right column, 20 min after 2 μM Xestospongin C (XC) application, the responses were also partly reduced. Two representative cells were shown in each treatment. (B) Quantitative CRI values of the inhibitor treatments. n = 3 (XC), or n = 6 (SC, 10PX1). Error bars, s.e.m., ANOVA, Dunnet’s correction, exact p-values. (C) Fluorescence patterns in cells that first responded to the stimulus after the treatments. Two representative cells are shown by ΔF/F. (D) Fluorescence patterns in several cells that first responded to the stimulus after the treatments; Scrambled (9 cells), 10PX1 (5 cells) and XC (6 cells).
We treated PC-3 cells with 10Panx1 peptide, a PANX1 inhibitor (Furlow et al., 2015). This results in a decrease in the FUS stimulated Ca2+ response (Figure 3). Ca2+ oscillations and double transients were eliminated but not the single transients (Figures 3C,D). This result is remarkably similar to Xestospongin C treatment (Figures 3A–D; see also Supplementary Videos S3–S5). The primary difference between 10Panx1 and Xestospongin C-treated cells was in the timing of the single Ca2+ transients. 10Panx1treated cells had a ∼20 s delay after stimulation to onset (relative to the control) (Figures 3C,D, middle) while Xestospongin C had a slightly longer ∼30 s delay. In both cases the response was maintained for ∼30–40 s (Figures 3C,D, right). Treatment with a scrambled version of 10Panx1 exhibited the normal Ca2+ response (three patterns). Treatment with 2 additional PANX1 inhibitors probenecid and carbenoxolone (CBX) completely eliminated Ca2+ response (Supplementary Figure S4 and Table 1). These data indicate that both PANX1 and IP3Rs likely initiate and maintain FUS-induced Ca2+ oscillatory responses. Simultaneous addition of 10Panx1 and Xestospongin C did not further reduce the Ca2+response suggesting that the underlying mechanisms are complementary rather than independent (Supplementary Figure S4), suggesting that they may be part of the same response pathway.
We next investigated a role for ER (or SR) localized PANX1 as a mediator of Ca2+ release by reducing PANX1 expression in PC-3 cells using si-PANX1RNA knockdown. Following treatment FUS-induced Ca2+ oscillations were variably reduced ∼50–70% (Figures 4A,B). Single or double calcium transients were delayed by ∼20 s compared to the control response (Figure 4C and Supplementary Videos S6, S7). We additionally transfected non-FUS responsive HEK cells (see Figures 1E,F) with WT PANX1 (i.e., a full-length WT PANX11–425 sequence). This construct had no EGFP fusion since this interferes with the Ca2+ imaging assay (see section Materials and Methods). The WT PANX1 transfection converts HEK cells to robust FUS stimulation dependent Ca2+ responsiveness (Figures 4D,E). We also transfected HEK cells with a mutant form of PANX11–89 lacking the normal C-terminal amino acids which was fused to mRFP (mt PANX1-mRFP). Interestingly, mt PANX1-mRFP transfection resulted in spontaneous Ca2+ activity even before FUS stimulation as well as exhibiting robust FUS-induced Ca2+ responsiveness (Figures 4D,E and Supplementary Videos S8–S10). The FUS-induced response in mt PANX1-mRFP transfected cells however was reduced relative to WT PANX1-transfected HEK cells (Figure 4E). Taken together these results indicate that PANX1 appears to be both necessary to generate FUS dependent Ca2+ responsiveness in PC-3 cells and sufficient to convert non-responsive HEK cells to a responsive state as well as generating non-FUS Ca2+ internal release dependent (i.e., Ca2+ leaks, Vanden Abeele et al., 2006).
Figure 4. PANX1 expression appears to be both necessary and sufficient for intracellular Ca2+ responses. (A) si-PANX1 RNA treatment in PC3 cells reduced Ca2+ responses compared to si-negative RNA (scramble) as a control. (B) Quantitative cell response index (CRI) values of the si-PANX1 RNA treatments relative to the control. n = 3. Error bars, s.e.m., exact p-values by a two-tailed t-test. (C) Fluorescence patterns in cells that first responded to the FUS stimulus after the treatments. One representative cell (top) is shown with fluorescence patterns in ten cells (bottom). (D) HEK293T cells transfected with WT PANX1 or mt PANX11–89-mRFP (mt PANX1-mRFP) constructs showed Ca2+ responses while control HEK cells transfected with dsRED construct have no FUS-induced Ca2+ response. (E) Quantitative CRI values of the transfected cells. n = 3. Error bars, s.e.m., ANOVA, Dunnet’s correction, exact p values.
We next established the cellular localization of PANX1 in PC-3 and HEK cells used in this study. HEK cells transfected with either a WT PANX1-EGFP fusion construct (WT PANX1-EGFP; Furlow et al., 2015) or mt PANX1-mRFP construct (Figure 5A) were imaged with wide field fluorescence microscopy. The mt PANX1-mRFP fluorescence is detected preferentially in perinuclear regions, consistent with expected ER localization (Furlow et al., 2015) while WT PANX1-EGFP fluorescence localized primarily to PM with a reduced signal localized to putative ER (Figure 5C). A similar result was obtained after staining PC-3 cells with anti-PANX1 antibodies that specifically recognize the N and C terminal located epitopes (Figure 5C). These observations, together with other studies (Vanden Abeele et al., 2006; Furlow et al., 2015), suggest that the C-terminus of PANX1 is important for PM localization and its absence results in mt PANX1-mRFP accumulating in ER. To further confirm this differential localization, we used high resolution total internal reflectance fluorescence (TIRF) microscopy of transfected HEK cells. WT PANX1-EGFP fluorescence is clearly detected by TIRF at PM while cells expressing mt PANX1-mRFP display little or no PM fluorescence (Figure 5E, the first column). Imaging the same cells using wide-field microscopy, both WT PANX1-EGFP and mt PANX1-mRFP signals are observed in the ER (Figure 5E, the middle columns). We conclude that WT PANX1-EGFP localizes to both ER and the PM, while mt PANX1-mRFP localization is restricted to ER. Combining this localization data with FUS-dependent stimulus data suggests that FUS may be capable of directly or indirectly stimulating ER-localized PANX1 to evoke the internal Ca2+ oscillations.
Figure 5. Localization of PANX1. (A,B) Schematic of fluorescent WT and mt PANX1 constructs (A) and the N and C-terminal epitopes recognized by anti-PANX1 antibodies (Ab) (B). (C) Localization of WT PANX1-EGFP and mt PANX1-mRFP in transfected HEK cells. (D) Localization of endogenous PANX1 in PC-3 cells using N- or C-terminal specific Abs. Nuclear DAPI stain is depicted as blue. (E) TIRF imaging on HEK cells transfected by WT PANX1-EGFP or mt PANX1-mRFP constructs. WT PANX1-EGFP localizes in the PM and the ER, while mt PANX1-mRFP only in the ER.
The cytoskeleton is believed to be important for the transmission of mechanical forces to internal cellular structures (Fletcher and Mullins, 2010; Cox et al., 2016) following US stimulation of mechanosensitive PM channels. PANX1 channels are mechanosensitive (Bao et al., 2004). We tested the role of cytoskeletal integrity in FUS stimulation by addition of cytoskeletal protein/process disrupters, including CytochalasinD (actin filaments), Nocodazole (microtubules), ML-7 and Blebbistatin (actomyosin contractility). FUS-evoked Ca2+ oscillatory responses appeared essentially normal in PC-3 cells when any of these disruptors were present (Figures 6A–C) and are thus not dependent on intact functional cytoskeletal proteins in invasive PC-3 cells. Additionally, this result suggests that FUS may be able to directly mechanostimulate ER localized PANX1. This result is in contrast with previous studies that identified an important role for cytoskeletal networks in transducing US stimuli (De Cock et al., 2015). An important difference between our high frequency non-contact focused US stimulus and most other studies is that the latter used low frequency US and required physical contact with the PM through microbubbles (Clapham, 2007; Carreras-Sureda et al., 2018; Burks et al., 2019). This could explain why an intact cytoskeleton appeared necessary for internal Ca2+ release in these other studies. We conclude from our results that FUS stimulation appears to be sufficient to result in internal mechanosensory activation of ER localized PANX1 and this coupling results in Ca2+ release from internal stores.
Figure 6. Effect of inhibitors of cytoskeletal support and actomyosin on FUS-induced Ca2+ responses in PC-3 cells. The responses are represented when cells were treated with mock, 2 μM CytochalasinD (CytoD), 5 μM ML-7, 5 μM Blebbistatin (Bleb), and 1 μM Nocodazole (Noc). (A) The percentage of responding cells over time. (B) The time at which each cell first responded to the stimulus. (C) Percentage of responding cells after the treatments. Cytoskeletal support and actomyosin did not affect FUS-induced calcium responses. None of them reduced the calcium responses, suggesting a distinctiveness of FUS. n = 4. Error bars, s.e.m., NS, not significant, by a one-tailed t-test.
We previously demonstrated that FUS-induced calcium waves were not caused by ultrasonic surface waves or gap junction-mediated paracrine signaling (Weitz et al., 2017). However, they may depend on paracrine as well as autocrine signaling via the release of extra-cellular messengers, such as ATP. To test this possibility, we performed FUS stimulation of PC-3 cells in the presence of extracellular apyrase (an ATP degrading enzyme) or in the presence of Suramin or PPADS (2 purinergic receptor blockers). These treatments completely abolished FUS- stimulated Ca2+ responses (Figures 7A–D). These data indicate that extracellular ATP can induce Ca2+ waves, and that FUS stimulation might evoke ATP release into the extracellular space where it activates PM-bound purinergic receptors on the same or nearby cells (e.g., P2X or P2Y). It is unlikely, however that P2×7 or P2Y1 receptors are involved in this process due to pharmacological studies summarized in Table 1 and Supplementary Figure S3.
Figure 7. Effects of intercellular Ca2+ wave inhibitors on Ca2+ response (A–D), and effects of PANX1 modulation on ATP release (E–G). The responses are represented when PC-3 cells were treated with Apyrase, Suramin, PPADS and mock; Mock (A), 20–50 units/ml Apyrase (B), 100 μM Suramin (C), and 100 μM PPADS (D). The percentage of responding cells over time was shown. Experimental results presented are representative and were independently replicates at least two times with three independent biological samples. (E) Quantification of PANX1-mediated ATP release from PC-3 cells pretreated for 15 min with scrambled (SC), 10PX1, or Xestospongin C (XC). n = 4. ANOVA, Dunnet’s correction, exact p-values. (F) Quantification of PANX1-mediated ATP release from PC-3 cells transfected with control si-negative or si-PANX1 RNA. n = 4, p-values by a two-tailed t-test. (G) Quantification of PANX1-mediated ATP release from HEK cells transfected with dsRED, mt PANX1-mRFP or WT PANX1-EGFP, and pretreated for 10 min with CBX (500 μM). n = 3. ANOVA, Dunnet’s correction, exact p-values. Error bars, s.e.m., NS, not significant.
In our previous study we showed that FUS stimulation and subsequent Ca2+ responses did not depend on formation of gap junctions (Weitz et al., 2017). This previous finding supports that PANX1 forms ATP permeant hemichannels which mediate extracellular Ca2+ wave propagation. To evaluate this, we treated PC-3 cells with 10PX1 or si-PANX1 and measured ATP levels. Treated cells have significantly reduced extracellular ATP release (Figures 7E,F), indicating that PC-3 cells mediate substantial ATP release through PANX1 channels.
To determine whether mt PANX1-mRFP or WT PANX1-EGFP alters extracellular ATP release through PANX1 channels, we measured CBX-sensitive extracellular ATP release from HEK cells expressing mt PANX1-mRFP or WT PANX1-EGFP. PANX1-mediated ATP release was quantified by measuring the reduction in ATP release in the presence of CBX (Thompson et al., 2008; Chekeni et al., 2010; Gulbransen et al., 2012). When WT PANX1-EGFP was expressed in HEK cells, CBX-sensitive ATP release was enhanced (Figure 7G). However, CBX-sensitive ATP release was not enhanced when mt PANX1-mRFP was expressed (Figure 7G). This suggests that mt PANX1-mRFP, localized to ER is capable of mediating intracellular Ca2+ release (see Figures 4D,E) but that it may operate differently than PM WT PANX1. Perhaps mt PANX1 lacking the C-terminal amino acids cannot form homo-oligomers which are necessary to form functional PM ATP release channels (Romanov et al., 2012; Wang et al., 2014; Wang and Dahl, 2018). Additional work will be necessary since the construct we used also contains an additional RFP fusion which may interfere with oligomer formation and we also cannot completely rule out the possibility of mt-PANX1 artifacts.
PANX1 is important for inflammasome activation (Silverman et al., 2009). Using a human cytokine array, we examined whether FUS effectively triggers PC-3 cells to secrete specific cytokines and chemokines. The assay was performed on the supernatants of cells grown for 1 day in media supplemented with charcoal-stripped fetal bovine serum after 46-MHz FUS stimulation repeated five times under several conditions (Figure 8A). PC-3 cells exhibited Ca2+ responses over a range of ultrasound intensities (∼300–1,155 mW/cm2) (Figures 1E–G), while non-invasive BPH-1 cells showed no response at this range of intensities (Weitz et al., 2017). Notably, FUS stimulation showed both qualitative and quantitative differences in the levels of cytokine and chemokine secretion from PC-3 cells as the intensity of stimulation was varied (Figure 8B). This suggests that FUS stimulation may be fine-tuned to control release of specific cytokine/chemokine profiles, an exciting possibility with potentially important therapeutic applications.
Figure 8. Cytokine and chemokine secretion from PC-3 cells following different intensity of FUS stimulation. (A) Protocol for FUS stimulation and a human cytokine array. (B) Cytokine and chemokine secretion from PC-3 cells following different intensity of FUS stimulation. n = 2. Error bars, s.e.m., NS, not significant, *P < 0.05; **P < 0.01 by a one-tailed t-test.
Our previous work demonstrated that mechanosensory FUS-stimulation generates a robust Ca2+ signaling response which can be used to distinguish invasive from non-invasive cancer cells (Hwang et al., 2013; Weitz et al., 2017). Others have also demonstrated mechanosensory Ca2+ signaling responses using non-focused US-stimulation in other contexts (Wood and Sehgal, 2015; Carina et al., 2018; Pan et al., 2018) as well as more general studies to clarify the physiological, cell biological and molecular mechanisms underlying mechanosensory dependent Ca2+ signaling responses (Tyler et al., 2008; Castellanos et al., 2016; Liu et al., 2017; Maresca et al., 2018). Our results include several new findings that further clarify the mechanically responsive Ca2+ signaling pathways and identify a new role for PANX1 in mediating the FUS-dependent responses. However, further studies would be needed to include more cancer and non-cancer prostate and other types of cancer cell lines to draw the general conclusion.
Removing or lowering external Ca2+ from culture medium did not eliminate FUS dependent Ca2+ signaling (see Figure 1D). This raised the possibility that an internal mechanosensory event is present and coupled to Ca2+ release from an internal storage site. Other work has also identified an ER dependent Ca2+ response mechanism using more conventional US stimulation involving IP3R activation (Burks et al., 2019). This response required an intact cytoskeleton believed to be important for the mechanotransduction of the stimulus to the ER membrane localized IP3R (Kim et al., 2015). However, in our study, FUS-dependent internal Ca2+ release is present even when cytoskeletal integrity has been disrupted (see Figure 6). This raises the interesting possibility that FUS mechanostimulation may be able to directly activate internal Ca2+ release and we identified mechanosensitive PANX1, partially localized to ER, as the potential internal target for this process (see Figures 3–5). However, the Ca2+ dynamics following mechanosensory stimulation is complex and needs further investigation before drawing specific conclusions regarding initiation of FUS-induced calcium signaling in cancer cells.
PANX1 is localized to the PM where it functions as an ATP release channel involved in intercellular signaling events (Wang and Dahl, 2018). We also confirm this for PC-3 cells (see Figures 7E–G). PANX1 channels respond to different types of chemical and mechanical stimuli with distinct channel open conformations (‘large’ and ‘small’) (Dahl, 2018). CBX and PB inhibit both PANX1 conformations (Dahl, 2018). In our study Ca2+ responses are eliminated in PC-3 cells treated with CBX or PB (Table 1 and Supplementary Figure S4) suggesting that these cells contain both PANX1 conformers.
The “small” conformer of PANX1 channel is reported to be impermeant to ATP (Romanov et al., 2012; Wang et al., 2014; Wang and Dahl, 2018). We show that HEK cells transfected with mt PANX1-mRFP also do not release ATP but can confer the internal Ca2+ response (see Figure 4). Perhaps the mt PANX1-mRFP construct we used is functionally similar to the “small” form of PANX1. Interestingly, a mutant truncated PANX1 channel (PANX11–89) has also been associated with highly metastatic breast cancer cells (Furlow et al., 2015). Taken together these results suggest that FUS-induced ER calcium release mediated through mt PANX1 may play a key role in cancer cell invasion and tumor metastasis. Further studies will be required to determine the mechanistic significance of this correlation.
While PANX1 has clearly been localized to ER (Vanden Abeele et al., 2006; Furlow et al., 2015, see also Figure 5) its functional significance is largely unknown. In addition to demonstrating its potential role in Ca2+ release from ER, we show a remarkable similarity with some aspects of previously established IP3R function in this regard (Mery et al., 2005; Sasse et al., 2007; Rizaner et al., 2016) (see Figure 3). Since Xestospongin C also inhibits SERCA Ca2+ pumps (Kume et al., 1997), the partial inhibition of the Ca2+ signal in Figure 3 by using Xestospongin C (Figure 3) might occur. Alternatively, the partial inhibition of the Ca2+ signal might be due to the relatively low concentration of Xestospongin C (2 μM). Effective IP3R inhibition monitoring at higher concentrations of Xestospongin C application, or agonist (IP3)-induced calcium rises, would be needed to validate the experiments. Since the simultaneous inhibition of IP3R and PANX1 exhibit similar patterns of FUS-dependent internal Ca2+ release they may be part of the same Ca2+ signaling pathway and provide a mechanism to transduce various stimuli into similar cellular responses.
At high intensities, FUS has been used clinically to thermally ablate tumor cells (Fus and Cancer Immunotherapy Workshop, 2019). Perhaps more importantly at lower intensities FUS has been shown to stimulate an inflammatory response in cancer models which can boost the efficacy of immunotherapy (Curley et al., 2017; Li et al., 2018; Mauri et al., 2018; Bonaventura et al., 2019). Low-intensity FUS, has not yet been used in cancer therapy, partly due to our limited understanding of its effects and mechanism of action. In our study we clearly show the potential to use “tuned” FUS to specifically control release of different cytokine/chemokine profiles from invasive cancer cells since this varies as the amplitude of the stimulation was changed (see Figure 8B). We are now attempting to extend this exciting observation to better understand FUS-induced anti-tumor immune response modulation by linking it to specific signaling pathways/molecules and epigenetic dynamics in simple cellular cancer models. This proof of principle work is critical before proceeding to in vivo experimentation or clinical utility.
For example, FUS applied to tumors could potentially modulate immune responses such as the ability to enhance infiltration of tumor targeting CAR T cells. Immunologically “cold” tumors are cancers that contain few infiltrating T cells thus making them impervious to current immunotherapy treatments (Li et al., 2018; Bonaventura et al., 2019). Classically immunologically “cold” cancers include glioblastomas, ovarian, prostate, pancreatic, and most breast cancers, all extremely resistant to current therapies. FUS can be potentially used as an adjunct therapy to induce secretion of cytokines/chemokines from ‘cold’ cancer cells and mediate conversion into a ‘hot’ tumor responsive to immunotherapy (Curley et al., 2017; Mauri et al., 2018). Of course, more work will be required in a well-controlled cellular model to understand the critical signaling pathways/molecules and mechanisms necessary for successful clinical translation of this technology.
FUS produces a focused beam of acoustic energy that precisely and accurately reaches large targets in the body without damaging surrounding normal cells (Mittelstein et al., 2020). One of the most striking findings of our study is the suggestion that FUS may also directly stimulate intracellular mechanosensory proteins located on particular membrane limited organelles such as ER. This rases the possibility that future studies could be designed to prove this by designing appropriate reporter constructs, i.e., sensors and bioswitches (Kim et al., 2015; Piraner et al., 2017), and optimizing stimulus parameters (e.g., amplitude, frequency, duty factor, and duration) and thus provide a new tool to study mechanosensitive intracellular processes.
In summary, we demonstrate that non-contact mediated FUS stimulates ER localized PANX1 to initiate an intracellular Ca2+ release. This process does not require an intact cytoskeleton and is independent of external Ca2+entry. PM localized PANX1, however, does appear necessary to mediate the intercellular spreading of Ca2+ waves likely through ATP release. In addition, FUS stimulation results in the release of specific chemokine/cytokine profiles from invasive PC-3 cancer cells. The specific cytokine/chemokine profile can be modified by varying FUS stimulus intensity.
Taken together our results suggest a new mechanistic working model for FUS-stimulation dependent Ca2+ signaling in cells which is shown schematically in Figure 9A. The initial cytoplasmic Ca2+ signal subsequently results in extracellular ATP release, possibly mediated by PM-PANX1 action and/or direct FUS stimulation. The ATP acts on purinergic receptors in nearby cells, thus propagating the spread of intercellular Ca2+ waves. Overall, these processes are not dependent on cytoskeletal integrity or other types of Ca2+ channels present in ER. The initial ER Ca2+ release, however, is not strictly related to mechanosensory stimulation of ER localized PANX1 but may also be influenced by ER localized IP3Rs as reported by others (Diver et al., 2001; Bootman et al., 2002; Xu et al., 2005). An additional response of FUS stimulation of PC-3 invasive cancer cells is the coupled release of specific cytokines/chemokines release from PC-3 cells. This new model can be compared to current working model largely derived from conventional US stimulation for comparison (Figure 9B) (Clapham, 2007; Carreras-Sureda et al., 2018; Burks et al., 2019).
Figure 9. (A) Schematic of new working model FUS-dependent response mechanisms in PC-3 invasive cancer cells. (1) FUS stimulation activates ER localized mechanosensitive PANX1 resulting in internal Ca2+ release from ER stores. (2) This cytoplasmic Ca2+ signal stimulates ATP release through PANX1 PM channels. (3) The released ATP acts on purineregic receptors, many in adjacent cells. (4) This results in a propagating extracellular Ca2+ wave which spreads through the cell population possibly via PM PANX1 or opening of PM Ca2+ channels. (5) FUS stimulation also results in secretion of cytokines/chemokines. (B) Schematic of currently accepted working model based largely on conventional US stimulation with no proposed role for internal ER Ca2+ release but rather a link to US energy transduction to ER mediated by IP3R. MB, Microbubbles.
All datasets presented in this study are included in the article/Supplementary Material.
NL conceived and designed experiments. NL and PS wrote the manuscript. CY, QW, SM, and KK executed the Ca2+ imaging. CY and QW analyzed the Ca2+ imaging. NL executed the all molecular and cell biological experiments and assays with CY and QW. HJ (46-MHz) and GL (3-MHz) characterized transducers with a needle hydrophone. RC and LJ fabricated transducers in house. AF and FP performed the TIRF imaging. AW helped develop calcium imaging program. PS, AW, RHC, and KS contributed helpful discussions/advice. CY, PS, AW, FP, and KS edited the manuscript with helpful suggestions. All authors reviewed the manuscript.
This work was supported by the National Institutes of Health grants P41-EB2182 and GM126016, and USC BME Baum chair account (KS), a private Salvaterra family foundation (NL) and the State Scholarship fund CSC (QW).
The authors declare that the research was conducted in the absence of any commercial or financial relationships that could be construed as a potential conflict of interest.
We thank Sohail F. Tavazoie (The Rockefeller University) for providing WT PANX1-EGFP and mt PANX1-mRFP cDNA constructs.
The Supplementary Material for this article can be found online at: https://www.frontiersin.org/articles/10.3389/fcell.2020.00504/full#supplementary-material
FIGURE S1 | Effect of FUS stimulation amplitude on PC-3 cell calcium response using 3-MHz transducer. All stated voltages represent peak-to-peak amplitude (Vp-p). Values in parentheses indicate the mV at each voltage, as measured by a hydrophone. (A) 2-D histograms showing the percentage of responding cells over time. (B) Scatter plots showing the time at which each cell first responded to the stimulus (each dot represents a responding cell). (C) Quantitative percentage of responding cells. n = 3. Error bars, s.e.m., n represents biological replicates.
FIGURE S2 | Effect of FUS stimulation amplitude on HEK cell calcium response using 46-MHz transducer. All stated voltages represent peak-to-peak amplitude (Vp-p). Values in parentheses indicate the mV at each voltage, as measured by a hydrophone. Quantitative percentage of responding cells. n = 3. Error bars, s.e.m., n represents biological replicates. Some spontaneous response background was occasionally shown, so the percentages of responding cells are ∼10%. However, the calcium response in HEK cells was not altered by different FUS stimulation amplitude, which is different from PC-3 cells.
FIGURE S3 | Effect of treatment of inhibitors on PC-3 cell calcium response. 2-D histograms showing the percentage of responding cells over time. (A) Effect of treatments of P2 receptor inhibitors on PC-3 cell calcium response. (B) Effect of treatments of Ca2+ influx inhibitors on PC-3 cell calcium response. These did not change the calcium response.
FIGURE S4 | Effect of both treatment of 10PX1 and XC on PC-3 cell calcium response using 46-MHz transducer. (A) 2-D histograms showing the percentage of responding cells over time. (B) Scatter plots showing the time at which each cell first responded to the stimulus (each dot represents a responding cell). (C) Effect of treatments of CBX, PB and FFA. The histograms showed the percentage of responding cells over time. Treatment of CBX, PB or FFA in PC-3 cells abolished Ca2+ responses.
FIGURE S5 | Quantitative RT-PCR analysis of WT PANX1 transcript expression in PC3 cells transfected two independent siRNAs that specifically target FL PANX1, as described previously; n = 2. Error bars, s.e.m., si-PANX1-N1 (L-018253-00) showed <35% reduction (A) so we did not use it. Another si-PANX1-N2 (D-018253-02) was used in most experiments and called as ‘si-PANX1’ (B). The variations of reduction occurred because of cell heterogeneity.
TABLE S1 | Pharmacological agents used to investigate the mechanism of FUS-induced calcium rise in PC-3 cells.
VIDEO S1 | Calcium responses of strongly invasive PC-3 prostate cancer cells to stimulation with 46-MHz low-intensity FUS. The FUS stimulus onset and offset times are 50 and 200 s, respectively.
VIDEO S2 | Calcium responses of non-invasive HEK 293T cells to stimulation with 46-MHz low-intensity FUS. The FUS stimulus onset and offset times are 50 and 200 s, respectively.
VIDEOS S3–S5 | Calcium responses of strongly invasive PC-3 prostate cancer cells after scrambled peptide (SC, S3), 10PX1 peptide (S4) and Xestospongin C (XC, S5) application for 20 min, to stimulation with 46-MHz low-intensity FUS. The FUS stimulus onset and offset times are 50 and 200 s, respectively.
VIDEOS S6, S7 | Calcium responses of strongly invasive PC-3 prostate cancer cells after si-negative (S6) or si-PANX1 (S7) treatments for 2 days, with 46-MHz low-intensity FUS. The FUS stimulus onset and offset times are 50 and 200 s, respectively.
VIDEOS S8–S10 | Calcium responses of non-invasive HEK 293T cells, after transfection of dsRED (S8), mt PANX1-mRFP (S9) or WT PANX1 (S10) constructs, to stimulation with 46-MHz low-intensity FUS. The FUS stimulus onset and offset times are 50 and 200 s, respectively.
Bao, L., Locovei, S., and Dahl, G. (2004). Pannexin membrane channels are mechanosensitive conduits for ATP. FEBS Lett. 572, 65–68. doi: 10.1016/j.febslet.2004.07.009
Bonaventura, P., Shekarian, T., Alcazer, V., Valladeau-Guilemond, J., Valsesia-Wittmann, S., Amigorena, S., et al. (2019). Cold tumors: a therapeutic challenge for immunotherapy. Front. Immunol. 10:168. doi: 10.3389/fimmu.2019.00168
Bootman, M. D., Collins, T. J., Mackenzie, L., Roderick, H. L., Berridge, M. J., and Peppiatt, C. M. (2002). 2-aminoethoxydiphenyl borate (2-APB) is a reliable blocker of store-operated Ca2+ entry but an inconsistent inhibitor of InsP3-induced Ca2+ release. FASEB J. 16, 1145–1150. doi: 10.1096/fj.02-0037rev
Burks, S. R., Lorsung, R. M., Nagle, M. E., Tu, T. W., and Frank, J. A. (2019). Focused ultrasound activates voltage-gated calcium channels through depolarizing TRPC1 sodium currents in kidney and skeletal muscle. Theranostics 9, 5517–5531. doi: 10.7150/thno.33876
Caliendo, F., Dukhinova, M., and Siciliano, V. (2019). Engineered cell-based therapeutics: synthetic biology meets immunology. Front. Bioeng. Biotechnol. 7:43. doi: 10.3389/fbioe.2019.00043
Carina, V., Costa, V., Pagani, S., De Luca, A., Raimondi, L., Bellavia, D., et al. (2018). Inhibitory effects of low intensity pulsed ultrasound on osteoclastogenesis induced in vitro by breast cancer cells. J. Exp. Clin. Cancer Res. 37:197. doi: 10.1186/s13046-018-0868-862
Carpenter, A. E., Jones, T. R., Lamprecht, M. R., Clarke, C., Kang, I. H., Friman, O., et al. (2006). CellProfiler: image analysis software for identifying and quantifying cell phenotypes. Genome Biol. 7:R100. doi: 10.1186/gb-2006-7-10-r100
Carreras-Sureda, A., Pihan, P., and Hetz, C. (2018). Calcium signaling at the endoplasmic reticulum: fine-tuning stress responses. Cell Calcium 70, 24–31. doi: 10.1016/j.ceca.2017.08.004
Castellanos, I., Balteanu, B., Singh, T., and Zderic, V. (2016). Therapeutic modulation of calcium dynamics using ultrasound and other energy-based techniques. IEEE Rev. Biomed. Eng. 9, 177–191. doi: 10.1109/RBME.2016.2555760
Chekeni, F. B., Elliott, M. R., Sandilos, J. K., Walk, S. F., Kinchen, J. M., Lazarowski, E. R., et al. (2010). Pannexin 1 channels mediate “find-me” signal release and membrane permeability during apoptosis. Nature 467, 863–867. doi: 10.1038/nature09413
Cox, C. D., Bae, C., Ziegler, L., Hartley, S., Nikolova-Krstevski, V., Rohde, P. R., et al. (2016). Removal of the mechanoprotective influence of the cytoskeleton reveals PIEZO1 is gated by bilayer tension. Nat. Commun. 7:10366. doi: 10.1038/ncomms10366
Curley, C. T., Sheybani, N. D., Bullock, T. N., and Price, R. J. (2017). Focused ultrasound immunotherapy for central nervous system pathologies: challenges and opportunities. Theranostics 7, 3608–3623. doi: 10.7150/thno.21225
Dahl, G. (2018). The Pannexin1 membrane channel: distinct conformations and functions. FEBS Lett. 592, 3201–3209. doi: 10.1002/1873-3468.13115
De Cock, I., Zagato, E., Braeckmans, K., Luan, Y., de Jong, N., De Smedt, S. C., et al. (2015). Ultrasound and microbubble mediated drug delivery: acoustic pressure as determinant for uptake via membrane pores or endocytosis. J. Control Release 197, 20–28. doi: 10.1016/j.jconrel.2014.10.031
Diver, J. M., Sage, S. O., and Rosado, J. A. (2001). The inositol trisphosphate receptor antagonist 2-aminoethoxydiphenylborate (2-APB) blocks Ca2+ entry channels in human platelets: cautions for its use in studying Ca2+ influx. Cell Calcium 30, 323–329. doi: 10.1054/ceca.2001.0239
Fletcher, D. A., and Mullins, R. D. (2010). Cell mechanics and the cytoskeleton. Nature 463, 485–492. doi: 10.1038/nature08908
Furlow, P. W., Zhang, S., Soong, T. D., Halberg, N., Goodarzi, H., Mangrum, C., et al. (2015). Mechanosensitive pannexin-1 channels mediate microvascular metastatic cell survival. Nat. Cell Biol. 17, 943–952. doi: 10.1038/ncb3194
Fus and Cancer Immunotherapy Workshop (2019). UVA Darden Sands Family Grounds, Arlington. Available Online at: Focused_ Ultrasound_and_Cancer_Immunotherapy_Workshop_White_Paper_2019.pdf (accessed July 18-19, 2019).
Gulbransen, B. D., Bashashati, M., Hirota, S. A., Gui, X., Roberts, J. A., MacDonald, J. A., et al. (2012). Activation of neuronal P2X7 receptor-pannexin-1 mediates death of enteric neurons during colitis. Nat. Med. 18, 600–604. doi: 10.1038/nm.2679
Hersh, D. S., Kim, A. J., Winkles, J. A., Eisenberg, H. M., Woodworth, G. F., and Frenkel, V. (2016). Emerging applications of therapeutic ultrasound in neuro-oncology: moving beyond tumor ablation. Neurosurgery 79, 643–654. doi: 10.1227/NEU.0000000000001399
Hwang, J. Y., Lee, N. S., Lee, C., Lam, K. H., Kim, H. H., Woo, J., et al. (2013). Investigating Contactless high frequency ultrasound microbeam stimulation for determination of invasion potential of breast cancer cells. Biotechnol. Bioeng. 110, 2697–2705. doi: 10.1002/bit.24923
Kim, T. J., Joo, C., Seong, J., Vafabakhsh, R., Botvinick, E. L., Berns, M. W., et al. (2015). Distinct mechanisms regulating mechanical force-induced Ca(2)(+) signals at the plasma membrane and the ER in human MSCs. eLife 4:e04876. doi: 10.7554/eLife.04876
Kume, S., Muto, A., Inoue, T., Suga, K., Okano, H., and Mikoshiba, K. (1997). Role of inositol 1,4,5-trisphosphate receptor in ventral signaling in Xenopus embryos. Science 278, 1940–1943. doi: 10.1126/science.278.5345.1940
Lam, K. H., Hsu, H. S., Li, Y., Lee, C., Lin, A., Zhou, Q., et al. (2013). Ultrahigh frequency lensless ultrasonic transducers for acoustic tweezers application. Biotechnol. Bioeng. 110, 881–886. doi: 10.1002/bit.24735
Lee, N. S., Evgrafov, O. V., Souaiaia, T., Bonyad, A., Herstein, J., Lee, J. Y., et al. (2015). Non-coding RNAs derived from an alternatively spliced REST transcript (REST-003) regulate breast cancer invasiveness. Sci. Rep. 5:11207. doi: 10.1038/srep11207
Li, J., Byrne, K. T., Yan, F., Yamazoe, T., Chen, Z., Baslan, T., et al. (2018). Tumor cell-intrinsic factors underlie heterogeneity of immune cell infiltration and response to immunotherapy. Immunity 49:e7. doi: 10.1016/j.immuni.2018.06.006
Liu, S. H., Lai, Y. L., Chen, B. L., and Yang, F. Y. (2017). Ultrasound enhances the expression of brain-derived neurotrophic factor in astrocyte through activation of TrkB-Akt and calcium-CaMK signaling pathways. Cereb. Cortex 27, 3152–3160. doi: 10.1093/cercor/bhw169
Lu, P., Zhu, X. Q., Xu, Z. L., Zhou, Q., Zhang, J., and Wu, F. (2009). Increased infiltration of activated tumor-infiltrating lymphocytes after high intensity focused ultrasound ablation of human breast cancer. Surgery 145, 286–293. doi: 10.1016/j.surg.2008.10.010
Maresca, D., Lakshmanan, A., Abedi, M., Bar-Zion, A., Farhadi, A., Lu, G. J., et al. (2018). Biomolecular ultrasound and sonogenetics. Annu. Rev. Chem. Biomol. Eng. 9, 229–252. doi: 10.1146/annurev-chembioeng-060817-084034
Mauri, G., Nicosia, L., Xu, Z., Di Pietro, S., Monfardini, L., Bonomo, G., et al. (2018). Focused ultrasound: tumour ablation and its potential to enhance immunological therapy to cancer. Br. J. Radiol. 91:20170641. doi: 10.1259/bjr.20170641
Mery, A., Aimond, F., Menard, C., Mikoshiba, K., Michalak, M., and Puceat, M. (2005). Initiation of embryonic cardiac pacemaker activity by inositol 1,4,5-trisphosphate-dependent calcium signaling. Mol. Biol. Cell 16, 2414–2423. doi: 10.1091/mbc.e04-10-0883
Mittelstein, D. R., Ye, J., Schibber, E. F., Roychoudhury, A., Martinez, L. T., Fekrazad, M. H., et al. (2020). Selective ablation of cancer cells with low intensity pulsed ultrasound. Appl. Phys. Lett. 116, 013701. doi: 10.1063/1.5128627
Nakayama, Y., Yoshimura, K., and Iida, H. (2012). Organellar mechanosensitive channels in fission yeast regulate the hypo-osmotic shock response. Nat. Commun. 3:1020. doi: 10.1038/ncomms2014
Pan, Y., Yoon, S., Sun, J., Huang, Z., Lee, C., Allen, M., et al. (2018). Mechanogenetics for the remote and noninvasive control of cancer immunotherapy. Proc. Natl. Acad. Sci. U.S.A. 115, 992–997. doi: 10.1073/pnas.1714900115
Piraner, D. I., Abedi, M. H., Moser, B. A., Lee-Gosselin, A., and Shapiro, M. G. (2017). Tunable thermal bioswitches for in vivo control of microbial therapeutics. Nat. Chem. Biol. 13, 75–80. doi: 10.1038/nchembio.2233
Rizaner, N., Onkal, R., Fraser, S. P., Pristera, A., Okuse, K., and Djamgoz, M. B. (2016). Intracellular calcium oscillations in strongly metastatic human breast and prostate cancer cells: control by voltage-gated sodium channel activity. Eur. Biophys. J. 45, 735–748. doi: 10.1007/s00249-016-1170-x
Romanov, R. A., Bystrova, M. F., Rogachevskaya, O. A., Sadovnikov, V. B., Shestopalov, V. I., and Kolesnikov, S. S. (2012). The ATP permeability of pannexin 1 channels in a heterologous system and in mammalian taste cells is dispensable. J. Cell Sci. 125, 5514–5523. doi: 10.1242/jcs.111062
Sasse, P., Zhang, J., Cleemann, L., Morad, M., Hescheler, J., and Fleischmann, B. K. (2007). Intracellular Ca2+ oscillations, a potential pacemaking mechanism in early embryonic heart cells. J. Gen. Physiol. 130, 133–144. doi: 10.1085/jgp.200609575
Silverman, W. R., de Rivero Vaccari, J. P., Locovei, S., Qiu, F., Carlsson, S. K., Scemes, E., et al. (2009). The pannexin 1 channel activates the inflammasome in neurons and astrocytes. J. Biol. Chem. 284, 18143–18151. doi: 10.1074/jbc.M109.004804
Thompson, R. J., Jackson, M. F., Olah, M. E., Rungta, R. L., Hines, D. J., Beazely, M. A., et al. (2008). Activation of pannexin-1 hemichannels augments aberrant bursting in the hippocampus. Science 322, 1555–1559. doi: 10.1126/science.1165209
Tokarew, N., Ogonek, J., Endres, S., von Bergwelt-Baildon, M., and Kobold, S. (2019). Teaching an old dog new tricks: next-generation CAR T cells. Br. J. Cancer 120, 26–37. doi: 10.1038/s41416-018-0325-321
Tyler, W. J., Tufail, Y., Finsterwald, M., Tauchmann, M. L., Olson, E. J., and Majestic, C. (2008). Remote excitation of neuronal circuits using low-intensity, low-frequency ultrasound. PLoS One 3:e3511. doi: 10.1371/journal.pone.0003511
Vanden Abeele, F., Bidaux, G., Gordienko, D., Beck, B., Panchin, Y. V., Baranova, A. V., et al. (2006). Functional implications of calcium permeability of the channel formed by pannexin 1. J. Cell Biol. 174, 535–546. doi: 10.1083/jcb.200601115
Wang, J., Ambrosi, C., Qiu, F., Jackson, D. G., Sosinsky, G., and Dahl, G. (2014). The membrane protein Pannexin1 forms two open-channel conformations depending on the mode of activation. Sci. Signal. 7:ra69. doi: 10.1126/scisignal.2005431
Wang, J., and Dahl, G. (2018). Pannexin1: a multifunction and multiconductance and/or permeability membrane channel. Am. J. Physiol. Physiol. 315, C290–C299. doi: 10.1152/ajpcell.00302.2017
Weitz, A. C., Lee, N. S., Yoon, C. W., Bonyad, A., Goo, K. S., Kim, S., et al. (2017). Functional assay of cancer cell invasion potential based on mechanotransduction of focused ultrasound. Front. Oncol. 7:161. doi: 10.3389/fonc.2017.00161
Wood, A. K., and Sehgal, C. M. (2015). A review of low-intensity ultrasound for cancer therapy. Ultrasound Med. Biol. 41, 905–928. doi: 10.1016/j.ultrasmedbio.2014.11.019
Xia, J., Lim, J. C., Lu, W., Beckel, J. M., Macarak, E. J., Laties, A. M., et al. (2012). Neurons respond directly to mechanical deformation with pannexin-mediated ATP release and autostimulation of P2X7 receptors. J. Physiol. 590, 2285–2304. doi: 10.1113/jphysiol.2012.227983
Xu, S. Z., Zeng, F. N., Boulay, G., Grimm, C., Harteneck, C., and Beech, D. J. (2005). Block of TRPC5 channels by 2-aminoethoxydiphenyl borate: a differential, extracellular and voltage-dependent effect. Br. J. Pharmacol. 145, 405–414. doi: 10.1038/sj.bjp.0706197
Keywords: mechanotranduction, focused ultrasound (FUS), calcium signaling, pannexin 1 (Panx1), ATP, invasive cancer cells
Citation: Lee NS, Yoon CW, Wang Q, Moon S, Koo KM, Jung H, Chen R, Jiang L, Lu G, Fernandez A, Chow RH, Weitz AC, Salvaterra PM, Pinaud F and Shung KK (2020) Focused Ultrasound Stimulates ER Localized Mechanosensitive PANNEXIN-1 to Mediate Intracellular Calcium Release in Invasive Cancer Cells. Front. Cell Dev. Biol. 8:504. doi: 10.3389/fcell.2020.00504
Received: 02 April 2020; Accepted: 27 May 2020;
Published: 23 June 2020.
Edited by:
Geert Bultynck, KU Leuven, BelgiumReviewed by:
Raphael Carmo Valente, Rio de Janeiro State University, BrazilCopyright © 2020 Lee, Yoon, Wang, Moon, Koo, Jung, Chen, Jiang, Lu, Fernandez, Chow, Weitz, Salvaterra, Pinaud and Shung. This is an open-access article distributed under the terms of the Creative Commons Attribution License (CC BY). The use, distribution or reproduction in other forums is permitted, provided the original author(s) and the copyright owner(s) are credited and that the original publication in this journal is cited, in accordance with accepted academic practice. No use, distribution or reproduction is permitted which does not comply with these terms.
*Correspondence: Nan Sook Lee, bmFubGVlQHVzYy5lZHU=; K. Kirk Shung, a2tzaHVuZ0B1c2MuZWR1
†Present address: Chi Woo Yoon, Institute of Engineering in Medicine, University of California, San Diego, La Jolla, CA, United States; Sunho Moon, Department of Aerospace and Mechanical Engineering, University of Notre Dame, Notre Dame, IN, United States
Disclaimer: All claims expressed in this article are solely those of the authors and do not necessarily represent those of their affiliated organizations, or those of the publisher, the editors and the reviewers. Any product that may be evaluated in this article or claim that may be made by its manufacturer is not guaranteed or endorsed by the publisher.
Research integrity at Frontiers
Learn more about the work of our research integrity team to safeguard the quality of each article we publish.