- 1Biotechnology of Animal and Human Reproduction (TechnoSperm), Institute of Food and Agricultural Technology, University of Girona, Girona, Spain
- 2Unit of Cell Biology, Department of Biology, Faculty of Sciences, University of Girona, Girona, Spain
- 3School of Agriculture and Food Science, University College Dublin, Dublin, Ireland
- 4Animal and Bioscience Research Department, Animal and Grassland Research and Innovation Centre, Teagasc Grange, Dunsany, Ireland
A growing body of evidence suggests that paternal factors have an impact on offspring development. These studies have been mainly carried out in mice, where seminal plasma (SP) has been shown to regulate endometrial gene expression and impact embryo development and subsequent offspring health. In cattle, infusion of SP into the uterus also induces changes in endometrial gene expression, however, evidence for an effect of SP on early embryo development is lacking. In addition, during natural mating, the bull ejaculates in the vagina; hence, it is not clear whether any SP reaches the uterus in this species. Thus, the aim of the present study was to determine whether SP exposure leads to improved early embryo survival and developmental rates in cattle. To this end, Day 7 in vitro produced blastocysts were transferred to heifers (12–15 per heifer) previously mated to vasectomized bulls (n = 13 heifers) or left unmated (n = 12 heifers; control). At Day 14, heifers were slaughtered, and conceptuses were recovered to assess size, morphology and expression of candidate genes involved in different developmental pathways. Additionally, CL volume at Day 7, and weight and volume of CL at Day 14 were recorded. No effect of SP on CL volume and weight not on conceptus recovery rate was observed. However, filamentous conceptuses recovered from SP-exposed heifers were longer in comparison to the control group and differed in expression of CALM1, CITED1, DLD, HNRNPDL, PTGS2, and TGFB3. In conclusion, data indicate that female exposure to SP during natural mating can affect conceptus development in cattle. This is probably achieved through modulation of the female reproductive environment at the time of mating.
Introduction
Despite the molecular complexity underlying the critical processes that take place in the peri-conception and early preimplantation period, the success of in vitro reproductive techniques suggests that the requirements of the embryo can be met by a relative simple set of media, and that embryonic development, at least to the blastocyst stage, occurs independently of interaction with the female reproductive tract. While blastocyst stage embryos can induce changes in the endometrium (Sponchiado et al., 2017; Passaro et al., 2018, 2019) as well as the uterine lumen metabolite composition (Sponchiado et al., 2019), mainly through embryo-derived interferon-tau (Passaro et al., 2019), whether this interaction plays an important role in embryo survival is questionable given the fact that embryos can be transferred to a virgin uterus as late as Day 16 and still establish a pregnancy (Betteridge et al., 1980). Nonetheless, it is becoming apparent that offspring health can be affected by the environmental conditions experienced during gamete maturation, embryo development and fetal growth (Hanson and Gluckman, 2014). While the link between maternal environment and embryo and offspring wellbeing has been investigated in detail, the role of paternal factors in directing offspring development has been somewhat overlooked (Morgan and Watkins, 2019). However, there is growing evidence that paternal nutrition and body mass composition have direct impact on DNA integrity, sperm quality and epigenetic status (Fleming et al., 2018), which has an effect on the metabolic function of the offspring in mice (Bromfield et al., 2014; Watkins and Sinclair, 2014; Watkins et al., 2017, 2018). In addition, seminal plasma (SP) has been shown to modulate gene expression and the immune response of the female reproductive tract in some species such as mice, human and cattle (Fazeli et al., 2004; Sharkey et al., 2007; Chen et al., 2014; Ibrahim et al., 2019).
Seminal plasma, a fluid resulting primarily from the secretions of the male accessory glands, transports, nourishes and protects sperm at the time of ejaculation (Bromfield, 2016). At this time, sperm are coated by SP proteins that are believed to prevent capacitation until they are close to the oocyte, and that modify sperm metabolism and motility (Vicens et al., 2014).
Female exposure to SP has been shown to improve embryo development and survival in mice (Bromfield et al., 2014), humans (Crawford et al., 2015), pigs (O’Leary et al., 2004) and golden hamsters (O et al., 1988). In addition, in llamas and rabbits, species with induced ovulation, SP has been reported to stimulate ovulation through nerve growth factor (Silva et al., 2011; Ratto et al., 2012, 2019; Adams and Ratto, 2013). It is thought that the beneficial effect of SP on the embryo is due in part to the immunoregulatory role. Indeed, leukocyte infiltration in response to SP in mice (Hunt and Robertson, 1996; Tremellen et al., 1998) and pigs (O’Leary et al., 2004) has been observed. Traditionally, this migration of immune cells was thought to solely serve the purpose of clearing microorganisms and excess sperm (O’Leary et al., 2004). However, it has been demonstrated that seminal Transforming Growth Factor Beta (TGFβ) stimulates Granulocyte-Macrophage Colony-Stimulating Factor (GM-CSF) secretion by uterine epithelial cells in vivo and in vitro in mice (Robertson et al., 1997; Tremellen et al., 1998; Moldenhauer et al., 2010). This factor promotes a pro-inflammatory cytokine and chemokine cascade, which recruits immune cells into the endometrial lumen and induces differentiation of tolerogenic dendritic cells and regulatory T cells (Treg cells) (Robertson et al., 2013). This differentiation depends on the micro-environmental cytokine signals which control the transition of naïve Th0 cells into Treg cells. As a result, immune tolerance to paternal antigens is probably established, which improves the ability of the semi-allogenic embryo to implant and develop normally (Robertson et al., 2009, 2018; Guerin et al., 2011). In addition to facilitating embryo implantation, in the mouse, exposure to semen induces the oviductal and uterine synthesis of embryotrophic cytokines such as GM-CSF, Interleukin 6 (IL6) and Leukaemia Inhibitory Factor (LIF) (Robertson, 2007). In this species, these factors have been shown to improve fetal growth and placentation (Sjöblom et al., 2005). Finally, regulation of ovarian function by SP has also been reported. In mice, the macrophage population in the corpus luteum (CL) has been observed to increase one day after mating in response to uterine exposure to SP (Gangnuss et al., 2004). Similarly, an increase in CL size and progesterone (P4) concentration in peripheral blood after SP exposure in pigs has been reported (O’Leary et al., 2006). Elevated P4 during the preimplantation stage has been shown to positively influence embryo growth in mice (Aisemberg et al., 2013), ruminants (Kleemann et al., 1994; Inskeep, 2004; Clemente et al., 2009; O’Hara et al., 2014a), alpacas (Bravo and Diaz, 2010), and pigs (Ashworth, 1991; Jindal et al., 1997). This is, at least in part, due to P4-stimulated endometrial secretions, collectively termed histotroph, which support conceptus development, implantation, and placentation (Simintiras et al., 2019). Thus, the SP-induced increase in P4 likely benefits embryo development and subsequent survival.
Despite the extensive body of evidence in the mouse, in cattle, the effect of SP on embryo development and survival is not clear. Both sperm and SP have been shown to induce expression of pro-inflammatory-related genes in the endometrium after infusion of SP into the bovine uterus (Elweza et al., 2018; Ibrahim et al., 2019; Ortiz et al., 2019). However, using a similar infusion technique, no improvement in the fertility of dairy heifers (Odhiambo et al., 2009) or cows (Ortiz et al., 2019) was observed. These apparent inter-species differences in the role of SP in fertility may be due, in part, to the known variation in composition of this fluid (Rodger, 1976; Druart et al., 2013), owing to differences in accessory gland size, type and level of fluid contribution to the ejaculate (Bedford, 2015). Indeed, caution is needed when interpreting some of the aforementioned bovine studies as some used SP collected by electroejaculation (Ibrahim et al., 2019), others by artificial vagina (Odhiambo et al., 2009) and others do not mention the collection method (Elweza et al., 2018; Ortiz et al., 2019). Collection method significantly influences the composition of SP (Rego et al., 2015) and consequently has a tremendous impact on endometrial response in vitro (Fernandez-Fuertes et al., 2019). As a result of these issues, interpretation of the available data in cattle is challenging.
In addition, the site of ejaculate deposition (i.e., intravaginal or intrauterine) likely determines differences in the response of female reproductive tissues to SP. Due to the characteristics of mating in rodents (Dean et al., 2011) and pigs (Hunter, 1981), SP comes into direct contact with the uterus. In contrast, during natural mating, the bull deposits the ejaculate in the anterior vagina of the cow (Hawk, 1983). Thus, it is not clear if any SP reaches the uterus in this species. However, at ejaculation, SP proteins bind to the sperm membrane (Pini et al., 2016), and can therefore be carried by these cells into more distal regions of the female reproductive tract. In fact, some SP proteins that bind to sperm, such as Binder of Sperm Protein (Suarez and Pacey, 2006) and osteopontin (Souza et al., 2008), have previously been described to influence embryo development in vitro in pigs (Hao et al., 2008) and cattle (Gonçalves et al., 2008; Monaco et al., 2009; Rodríguez-Villamil et al., 2016). However, although bovine sperm can bind to both endometrial and oviductal cells in vitro and stimulate mRNA expression of different cytokines (Yousef et al., 2016; Elweza et al., 2018; Ezz et al., 2019), incubation of endometrial explants with cauda epididymis sperm (which are mature but have had no contact with SP) or Percoll-washed ejaculated sperm did not induce differences in mRNA expression of some of those cytokines (Fernandez-Fuertes et al., 2019). Thus, it is not clear if SP-derived proteins, rather than intrinsic sperm proteins, are responsible for eliciting changes in the endometrium of cattle.
It is also possible that, in species that deposit semen in the vagina, SP elicits a local response that propagates to more distal regions of the female reproductive tract. In humans, unprotected vaginal coitus leads to enhanced cytokine and chemokine expression in the cervix (Sharkey et al., 2012). These factors could travel through local circulation to elicit changes in other reproductive organs, as seems to be the case in pigs, rodents, and cattle. In the bovine, SP infusion into the vagina, but not the uterus, induces an increase in endometrial epidermal growth factor concentrations (Badrakh et al., 2020). Whereas mating (in the mouse) or infusion of SP (in the sow) have been shown to have an effect on ovarian function (Gangnuss et al., 2004; O’Leary et al., 2006).
Taken collectively, the literature suggests that although SP is not essential for pregnancy success, it can improve embryo development and survival through modulation of the maternal environment. However, to the best of our knowledge, there is currently no evidence for a role of SP in early embryo development in cattle in vivo. Thus, the aim of the present study was to assess the effect of SP exposure in cattle through natural mating on pre-implantation embryo survival and conceptus development. To this end, a model in which heifers were mated to vasectomized bulls (which only ejaculate SP) or left unmated (control) was used. In vitro produced embryos were transferred to the heifers at Day 7 post-mating and were recovered after slaughter at Day 14 to assess conceptus size, morphology and gene expression. In addition, CL volume at Day 7, as well as weight and volume at Day 14 were analyzed.
Materials and Methods
Animals
All experimental procedures involving animals were approved by the Animal Research Ethics Committee of University College Dublin, Ireland, and the Universitat de Girona, Spain, and licensed by the Health Products Regulatory Authority (HPRA), Ireland, in accordance with Statutory Instrument No. 543 of 2012 (under Directive 2010/63/EU on the Protection of Animals used for Scientific Purposes). Throughout the course of the experiment, all animals were housed at Teagasc Grange, Animal and Grassland Research Centre, Dunsany, Ireland.
Experimental Design
The estrous cycles of crossbred beef heifers (mainly Angus and Holstein-Friesian cross; n = 27) were synchronized using an 8-day intravaginal device (PRID® Delta, 1.55 g progesterone; Ceva Santé Animale; Libourne, France), together with a 2 mL intramuscular injection of a synthetic gonadotrophin releasing hormone (Ovarelin®, equivalent to 100 μg Gonadorelin; Ceva Santé Animale) administered on the day of PRID insertion. One day prior to PRID removal, all heifers received a 5 mL intramuscular injection of PGF2α (Enzaprost®, equivalent to 25 mg of Dinoprost; Ceva Santé Animale) to induce luteolysis. Only heifers observed in standing estrus (Day -1; n = 25) were blocked by weight and randomly allocated to one of two treatments: (1) mated with a vasectomized bull (n = 13), or (2) left unmated (control; n = 12). Each mated heifer was hand-mated once to one of three vasectomized Holstein Friesian bulls within 7 h of the start of standing estrus. Bulls were allowed to mate no more than twice per day and the experiment was carried out over three consecutive days. Seven days after mating, in vitro produced blastocysts were transferred to each heifer (n = 12–15 per heifer). All heifers were slaughtered in a commercial abattoir 7 days after embryo transfer to recover Day 14 conceptuses. In addition, CL volume at Day 7, and CL weight and volume at Day 14 were recorded. The experimental design is summarized in Figure 1.
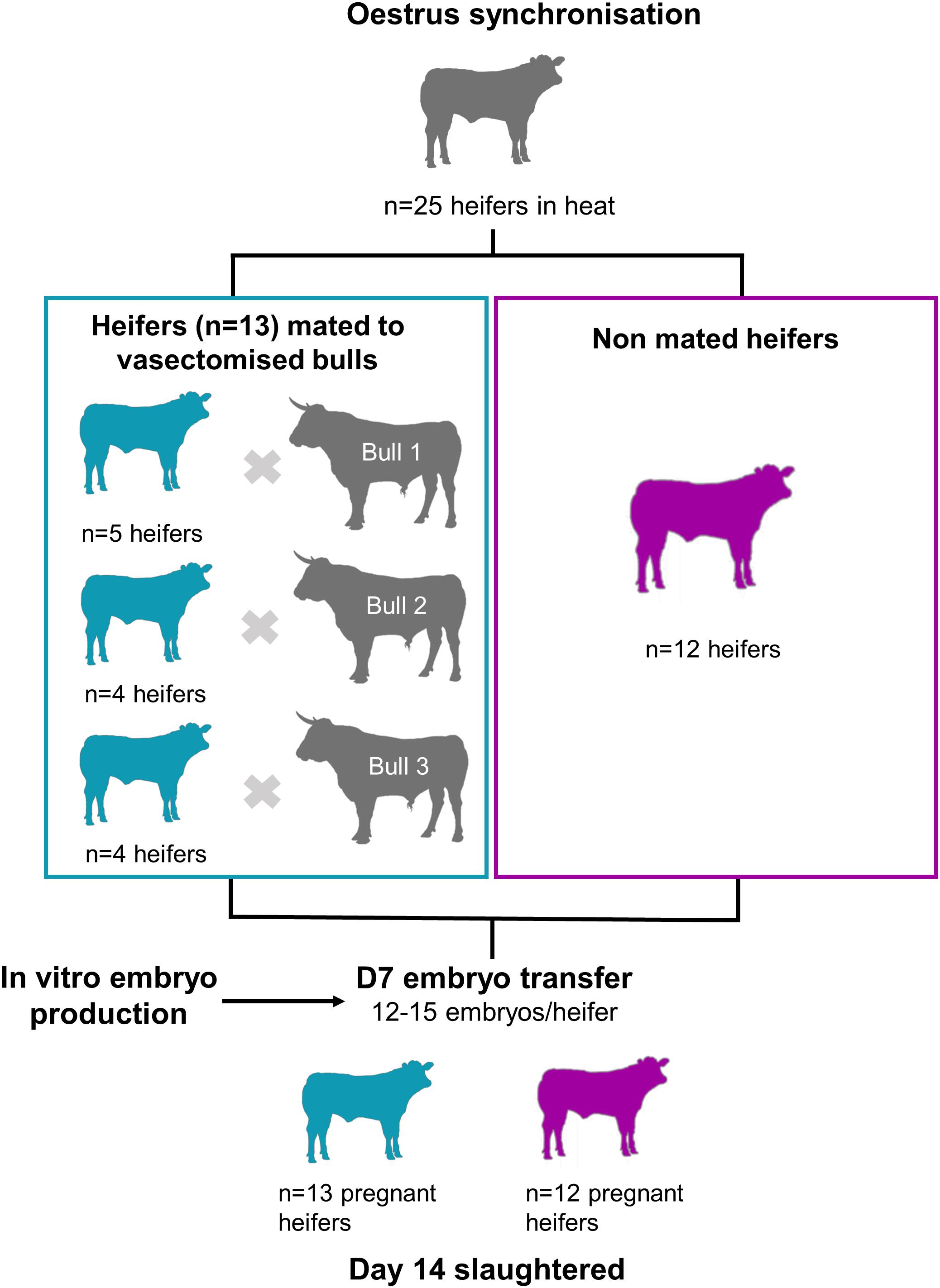
Figure 1. Experimental design. Heifers observed in standing estrus (n = 25) were blocked by weight and randomly allocated to one of two treatments: mated with vasectomised bulls (blue) or left un-mated (purple). Seven days later, 12–15 in vitro produced blastocysts were transferred to all heifers. Heifers were slaughtered at Day 14, and the conceptuses were recovered from the uterine horns.
In vitro Embryo Production
Ovaries from cows and heifers were collected at a commercial abattoir and surface visible follicles (>2 mm) were aspirated to recover cumulus-oocyte complexes (COCs). Good quality COCs were matured in TCM-199 (Sigma Aldrich, Saint Louis, MO, United States) supplemented with 10% (v/v) FCS (Sigma Aldrich) and 10 ng/mL Epidermal Growth Factor (Merck; Darmstadt, Germany) (n = 50 COCs per well) for 24 h at 39°C under an atmosphere of 5% CO2 in air with maximum humidity. Matured COCs were fertilized using sperm from a bull of proven in vitro fertility at a concentration of 1 × 106 sperm/mL. Frozen-thawed semen from the same bull was used throughout. Motile sperm were selected by centrifugation through a 95–45% discontinuous Percoll gradient (Merck) for 10 min at 700 g, followed by a second centrifugation in HEPES-buffered Tyrode medium (Boston BioProducts; MA, United States) at 100 g for 5 min. Gametes were co-incubated at 39°C in an atmosphere of 5% CO2 in air with maximum humidity. Approximately 20 h post-insemination, cumulus cells were removed, and presumptive zygotes were cultured in 25 μL droplets of synthetic oviduct fluid supplemented with 5% FCS (n = 25 per well) under mineral oil (Sigma Aldrich) until Day 7 (Day 0 = day of fertilization). Culture dishes were kept at 39°C under an atmosphere of 5% CO2 and 5% O2 in air with maximum humidity until Day 7. Blastocysts classified as excellent or good (following guidelines of the International Embryo Technology Society, 2009) were pooled, and then randomly loaded into straws (n = 12–15 embryos/straw) for embryo transfer.
Embryo Transfer and Recovery
On Day 7 (Day 0 = day of in vitro fertilization for the embryos, and day after mating for the heifers) the Day 7 in vitro-produced blastocysts were transferred (n = 12–15 blastocysts/heifer) to the horn ipsilateral to the ovary bearing the CL. All heifers were slaughtered on Day 14 (7 days after embryo transfer). Reproductive tracts were recovered, gently dissected, and flushed with PBS containing 5% FCS within 30 min of slaughter. The number and dimensions (length and width) of recovered Day 14 embryos were recorded. Conceptuses were classified based on morphology as ovoid (1–4 mm), tubular (5–19 mm), or filamentous (>20 mm), based on previous studies (Ribeiro et al., 2016). Due to the large range in conceptus length in the filamentous group, the filamentous group was subdivided in short filamentous (20–25 mm) and long filamentous (>25 mm) for gene expression analysis.
Calculation of CL Weight and Volume
Corpus luteum volume was calculated as described previously (Scully et al., 2014; Parr et al., 2017). Briefly, for Day 7 CL, the formula for the volume of a sphere was used (V = 4/3πr3). The radius was calculated as the average of the two cross-sectional ultrasound measurements (the CL diameter) divided by two. For those CL with a cavity, the volume of the cavity was calculated using the same formula and subtracted from the total CL volume.
Because measurements in three axes (a, b, c) could be taken from post-mortem Day 14 CL, the formula for the volume of an ellipsoid (V = 4/3πabc) was used (Grygar et al., 1997). As above, for CL with a cavity, the volume of the cavity was subtracted from the total CL volume. Moreover, the weight of luteal tissue of these CL was also recorded.
Quantitative Real-Time PCR Analysis
Quantitative real-time PCR (RT-qPCR) was used to investigate changes in relative abundance of candidate transcripts in all Day 14 conceptuses due to treatment. A panel of five genes was used to determine conceptus gastrulation stage based on Degrelle et al. (2011): Calmodulin 1 (CALM1), Cbp/P300 interacting transactivator with Glu/Asp rich carboxy-terminal (CITED1), Dihydrolipoamide Dehydrogenase (DLD), Heterogeneous Nuclear Ribonucleoprotein D Like (HNRNPDL), and Transforming Growth Factor Beta 3 (TGFB3). However, under the conditions of the current study, the gene expression patterns that the authors described to classify gastrulation stage were not observed. Because the aforementioned genes are involved in different functional pathways within the conceptus, additional genes that participate in such pathways, and that have been found to be differentially expressed along development (Mamo et al., 2011; Barnwell et al., 2016) were interrogated: Caspase 3 (CASP3), Furin (FURIN), Glutathione S-Transferase Mu 1 (GSTM1), IL6, MHC Class I JSP 1 (JSP1), and Prostaglandin-Endoperoxide Synthase 2 (PTGS2).
Total RNA was extracted from entire conceptuses using Trizol reagent (Invitrogen; Carlsbad, CA, United States) and trimethylene chlorobromide (Sigma Aldrich). On-column DNase digestion and RNA clean-up was performed using the RNeasy Mini Kit (Qiagen; Hilden, Germany) following the manufacturer’s instructions. The quantity and purity of RNA was determined using the Epoch Microplate Spectrophotometer (BioTek; Winooski, VT, United States). For each sample, cDNA was prepared from 14.7 ng of total RNA (based on the sample with lowest RNA concentration) using the High-Capacity cDNA Reverse Transcription Kit (ThermoFisher Scientific; Waltham, MA, United States) according to the manufacturer’s instructions. For the PCR negative control, a retrotranscription mastermix without the enzyme was applied to an RNA pool of a representative sample of conceptuses.
All primers were designed using Primer Blast software1 (Table 1). In order to identify the most suitable housekeeping genes, duplicate qPCR assays were performed in a total volume of 20 μL, containing 10 μL Fast SYBR Green Master Mix (ThermoFisher Scientific), 1.2 μL forward and reverse primer mix (5 nM final concentration), 5.1 μL Nuclease-Free Water (ThermoFisher Scientific) and 2.5 ng of a representative sample of embryos. The Applied Biosystems 7500 Real-Time PCR Systems (ThermoFisher Scientific) was used and the thermo-cycling conditions were as follows: 1 cycle of holding stage at 50°C for 2 min and 95°C for 10 min; 40 cycles of cycling stage at 95°C for 15 s and 60°C for 1 min and, finally, 1 cycle of melt curve stage at 95°C for 15 s, 60°C for 1 min, 95°C for 30 s and 60°C for 15 s. The presence of a single sharp peak in the melt curve analysis confirmed the specificity of all targets. A total of eight potential reference genes [Glyceraldehyde 3-Phosphate Dehydrogenase (GAPDH), Actin Cytoplasmic 1 (ACTB), 60S Ribosomal Protein L18 (RPL18), Peptidyl-Prolyl Cis-Trans Isomerase A (PPIA), 14-3-3 Protein Zeta/Delta (YWHAZ), RING Finger Protein 11 (RNF11), Histone H3.3 (H3F3A), Succinate Dehydrogenase Complex Subunit A Flavoprotein Variant (SDHA)] were analyzed using the geNorm function of the Qbase + package (Biogazelle; Zwijnaarde, Belgium) to identify the most appropriate for the study (Vandesompele et al., 2002). Because they were more stably expressed (average GeNorm M ≤ 0.5), the reference genes selected were RPL19 and PPIA.
Primer efficiency was carried out for the genes of interest, and qPCR of 1:4 dilutions of a cDNA mix from a representative pool of conceptuses were analyzed. The presence of a single sharp peak in the melt curve as well as the standard curve was used to confirm primer specificity. Average primer efficiency was 93.0 ± 4.7%. The expression of these genes was individually evaluated in 76 conceptuses (20 ovoid, 20 tubular, 36 filamentous) using 2.5 ng of cDNA, 10 μL of Fast SYBRTM Green Master Mix, 1.2 μL of 5 nM primers and 5.1 μL nuclease-free water, and the thermo-cycling conditions previously detailed.
The comparative Livak Ct method (ΔΔCt method; Livak and Schmittgen, 2001) was used to quantify the relative gene expression levels. First, for each conceptus, the expression of the gene of interest was normalized to the expression of the two housekeeping genes (RPL19 and PPIA), using the following formula: ΔCt = Ctgeneof interest−CtRPL19+PPIA/2. To calculate the ΔΔCt, results were scaled to the average ΔCt across all conceptuses per target. The ΔCt values were used for the subsequent statistical analysis and results are presented as 2(–Δ Δ Ct).
Statistical Analysis
Data relating to conceptus and CL sizes were checked for normality and homogeneity of variance by histograms, Qplots, and formal statistical tests as part of the UNIVARIATE procedure of SAS (version 9.1.3; SAS Institute, Cary, NC, United States). Conceptus size data were not normally distributed and, as such, were transformed by raising the variable to the power of lambda. The appropriate lambda value was obtained by conducting a Box–Cox transformation analysis using the TRANSREG procedure of SAS. The transformed data were used to calculate P values. The corresponding least squares means and standard error of the non-transformed data are presented in the results. Conceptus data and CL data (on Days 7 and 14) were analyzed using a mixed model (PROC MIXED of SAS). The model had experimental treatment (Control or Vasectomized) as a fixed effect, and heifer within treatment was included as a random effect. Differences between treatments were determined by F tests using type III sums of squares. The PDIFF command incorporating the Tukey test was applied to evaluate pairwise comparisons between treatment means. Values were statistically significantly different when P ≤ 0.05 and considered a tendency when P ≤ 0.10.
Gene expression data were analyzed with IBM SPSS 25.0 for Windows (Armonk; New York, NY, United States). First, data were checked for normal distribution (Shapiro-Wilk test) and homogeneity of variance (Levene test), premises for linear models. In those cases in which these premises were not met, data (x) were transformed using the arcsine of the square root (arcsin √x). Later, data (transformed or not depending on the case) were analyzed by an ANOVA of two factors followed by a Sidak post hoc test for pairs comparison. Since even after arcsin-transformation, expression of CALM1, CASP3, CITED1, DLD, and TFGB3 did not match with parametric assumptions, Scheirer-Ray-Hare and Mann-Whitney tests were used as alternatives. In all cases, the significance level was established at P ≤ 0.05.
Results
Effect of SP Exposure on CL Size
Exposure to SP through mating with a vasectomized bull did not elicit differences in the CL volume at Day 7 or at Day 14 (Day 7: 7.1 ± 0.76 cm3 vs. 6.5 ± 0.49 cm3, for mated and unmated heifers, respectively, P > 0.05; Day 14: 3.1 ± 0.39 cm3 vs. 4.5 ± 0.91 cm3, respectively, P > 0.05). Similarly, no difference in CL weight on Day 14 was observed between SP-exposed and control heifers (5.1 ± 0.46 g vs. 6.4 ± 0.79 g, respectively; P > 0.05).
Effect of SP Exposure on Embryo Viability and Morphology
Conceptus recovery rate was similar from mated (exposed to SP) and unmated heifers (86/168: 51 ± 8.4% vs. 78/153: 51 ± 8.1%, respectively, P > 0.05; Figure 2A), indicating a lack of effect of SP-exposure on the survival of the transferred embryos. As is normal in cattle studies in which multiple-embryo transfer is carried out (O’Hara et al., 2014b), considerable variation in conceptus length within heifer was observed in both groups (CV 44–79%). However, conceptuses recovered from heifers mated to vasectomized bulls tended to be longer than those recovered from control heifers (16 ± 1.3 mm vs. 12 ± 1.2 mm, respectively, P = 0.07; Figure 2B).
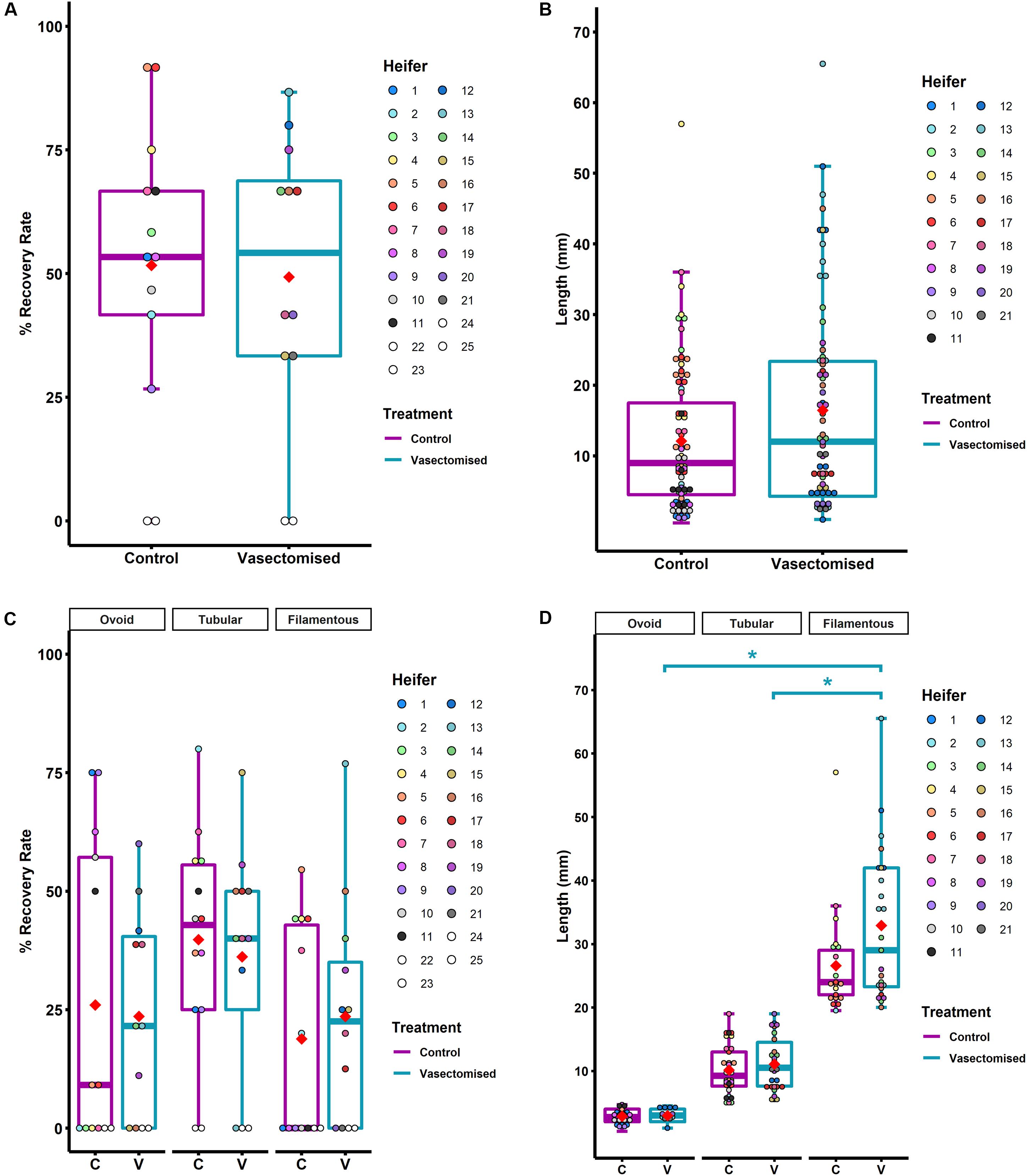
Figure 2. (A) Conceptus recovery rate at Day 14 post-mating (vasectomized; n = 12 heifers) or post-estrus onset (control; n = 13 heifers). Each color represents one heifer. (B) Length of the recovered conceptuses post-mating (vasectomized; n = 78) or post-estrus onset (control; n = 86). Dots represent the conceptus length and their color reflects the heifer from which they were recovered. (C) Conceptus recovery rate at Day 14 depending on morphology (for control (C): ovoid n = 24, tubular n = 40, filamentous n = 22; for vasectomized (V): ovoid n = 21, tubular n = 21, filamentous n = 27). Dots represent the recovery rate of each heifer for each morphology. (D) Length of the recovered conceptuses depending on morphology [for control (C): ovoid n = 24, tubular n = 40, filamentous n = 22; for vasectomized (V): ovoid n = 21, tubular n = 21, filamentous n = 27]. Dots represent the conceptus length and their color depends on the heifer from which they were recovered. In all graphs, means are represented as red rhombus. Control group box-plots are represented in purple and vasectomized group box-plot, in blue. For the dots, light scale colors correspond to the control group and dark scale, to heifers mated to vasectomized bulls. Differences are indicated as *P ≤ 0.05.
Moreover, although no differences were observed in the percentage of ovoid, tubular and filamentous conceptuses recovered between groups (24/86: 26 ± 8.8%, 40/86: 40 ± 6.4%, 22/86: 18 ± 6.2% in control heifers and 21/78: 23 ± 6.2%, 30/78: 36 ± 6.9%, 27/78: 24 ± 6.8% in mated heifers; P > 0.05; Figure 2C), filamentous conceptuses recovered from SP-exposed heifers were longer than those recovered from control heifers (33 ± 2.2 mm vs. 27 ± 1.8 mm, respectively; P < 0.05; Figure 2D). Due to the large range in filamentous conceptus length, this group was subdivided into short filamentous (20–25 mm) and long filamentous (>25 mm) for subsequent gene expression analysis. In the control group, 6/86 conceptuses (7 ± 3.8%) were classified as long filamentous (average size 36 ± 4.7 mm; n = 6). In the SP-exposed group, 14/78 (18 ± 5.6%) conceptuses exhibited this morphology (average size 42 ± 2.4 mm; n = 14).
Effect of SP on Conceptus Gene Expression
In order to more accurately evaluate the developmental stage of the conceptuses, the relative abundance of transcripts for five candidate genes (CALM1, CITED1, DLD, HNRNPDL, and TFGB3) previously described as gastrulation markers (Degrelle et al., 2011) was assessed. However, the expression profiles described by Degrelle et al. (2011) in association with different developmental stages were not observed. Nevertheless, these genes are involved in important pathways for embryo development, and their expression changes are temporally regulated. In order to better characterize these pathways, an additional set of genes related to metabolism (GSTM1 and PTGS2), apoptosis (CASP3), development (FURIN), and immunology (JSP1 and IL6) (Mamo et al., 2011; Barnwell et al., 2016) was also interrogated. All these genes were analyzed in individual conceptuses exhibiting different morphologies (ovoid, tubular, short filamentous, and long filamentous).
The relative abundance of CITED1, HNRNPDL, IL6, JSP1, and TGFB3 was affected by conceptus morphology in both the control and vasectomized groups. Long and short filamentous conceptuses recovered from control or SP-exposed heifers had lower JSP1 expression (P < 0.05; Figure 3I). On the other hand, in both treatments, IL6 relative expression was higher in short filamentous conceptuses in comparison to ovoid embryos (P < 0.05; Figure 3H); control long and short filamentous embryos also had higher relative abundances of this gene compared to ovoid and tubular conceptuses (P < 0.05; Figure 3H). In control conceptuses, CITED1 and TGFB3 relative expression was highest in long filamentous conceptuses (P < 0.01; Figures 3C,K), while in the vasectomized group such conceptuses exhibited the lowest relative expression of CITED1 (P < 0.05; Figure 3C) and both long and short conceptuses had lower TGFB3 expression compared to ovoid conceptuses (P < 0.01; Figure 3K). Additionally, HNRNPDL relative expression in the control group was highest in short filamentous conceptuses (P < 0.01; Figure 3G), whereas in the vasectomized group, the expression of this gene was lowest in both short and long filamentous conceptuses (P < 0.01; Figure 3G). In addition to these, in the control group, long filamentous conceptuses also exhibited the lowest CALM1 and DLD relative abundance (P < 0.01; Figures 3A,D); while FURIN relative expression in short filamentous conceptuses was lower than ovoid and tubular embryos (P < 0.05; Figure 3E). On the contrary, higher PTGS2 expression was detected in long and short filamentous conceptuses in comparison to ovoid conceptuses (P < 0.05; Figure 3J).
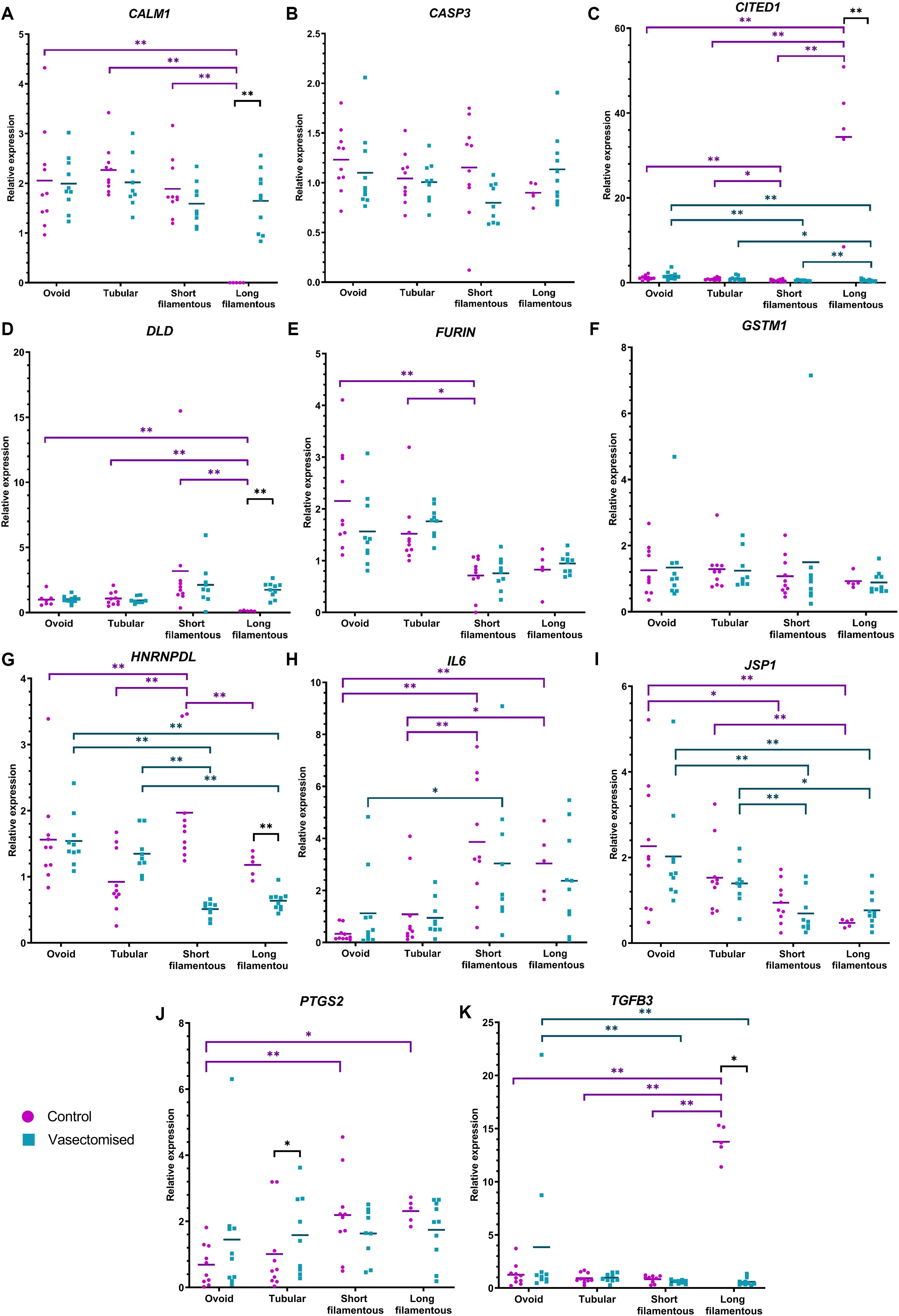
Figure 3. Relative expression of CALM1 (A), CASP3 (B), CITED1 (C), DLD (D), FURIN (E), GSTM1 (F), HNRNPDL (G), IL6 (H), JSP1 (I), PTGS2 (J), and TGFB3 (K) among the different conceptus morphologies (for control: ovoid n = 10, tubular n = 10, short filamentous n = 10 and long filamentous n = 5; for vasectomized: ovoid n = 10, tubular n = 10, short filamentous n = 10 and long filamentous n = 10). Individual expression values (calculated with 2– ΔΔCt method, using the housekeeping genes RPL19 and PPIA) with bar representing the mean are presented. Purple dots correspond to the control group and blue squares correspond to the vasectomized group. Differences are indicated as *P ≤ 0.05 and **P ≤ 0.01.
Relative expression of CALM1, CITED1, DLD, HNRNPDL, PTGS2, and TGFB3 differed between treatments. Long filamentous conceptuses recovered from mated heifers presented lower CITED1, HNRNPDL, and TGFB3 expression, and higher CALM1 and DLD expression levels, compared to morphology-matched conceptuses recovered from control heifers (P < 0.05; Figure 3). In addition, PTGS2 relative expression was higher in tubular conceptuses recovered from conceptuses that developed in a SP-primed environment than in control heifers (P < 0.05; Figure 3J).
Discussion
The main findings of this study are that exposure of heifers to SP through natural mating with vasectomized bulls: (1) does not elicit changes in the size of Day 7 or Day 14 CL; (2) does not improve embryo survival to Day 14, but (3) is associated with an increase in conceptus length and (4) alteration in expression of CALM1, PTGS2, CITED1, DLD, HNRNPDL, and TGFB3.
In recent years, the paternal influence on offspring health has gained increasing interest. This is due to studies showing that paternal health and nutrition can affect offspring development, and such effects can be carried over to the next generation (Morgan et al., 2020). One may immediately assume that the changes elicited in the embryo and subsequent individual are directly linked to abnormalities in the sperm of these males, which manage to fertilize the oocyte and transmit certain epigenetic signatures. However, mating of artificially-inseminated females to vasectomized mice fed different diets also has an effect on offspring outcomes (Watkins et al., 2018; Morgan et al., 2020), indicating that SP-induced changes in the female reproductive tract at the time of mating affect embryo development. Indeed, studies in mice and pigs have demonstrated that SP plays a role in the modulation of the maternal environment and, as a result, improves embryo survival and implantation (Johansson et al., 2004; O’Leary et al., 2004; Bromfield et al., 2014). In cattle, however, while SP infusion into the uterus alters the expression of certain genes (Ibrahim et al., 2019), this is not correlated with improved pregnancy rates (Odhiambo et al., 2009; Ortiz et al., 2019). These differences between species may reflect differences in SP composition (Rodger, 1976; Druart et al., 2013). As SP is a complex secretion produced by different accessory glands, variation in the type, structure and size of these organs can have a major impact on its composition (Bedford, 2015). For example, while the boar has large bulbourethral, prostate and vesicular glands, the latter two are relatively small in the bull (Druart et al., 2013). These two species only share 34% of their SP proteins in common (Druart et al., 2013). Perhaps more interesting to the subject at hand, both rodents and boars have an additional accessory gland that is lacking in the bull: the coagulating gland. This gland contributes to semen coagulation after ejaculation, which has been suggested to make sperm coating by SP proteins highly inefficient (Lefebvre et al., 2007). Thus, direct contact of the endometrium with the ejaculate might be more important in these species than in the bovine, where sperm can act as a vehicle for SP proteins. Indeed, it is important to note that in both mice and pigs, SP reaches the uterus during mating, while in cattle, the ejaculate is deposited in the anterior vagina (Hawk, 1983), and it is not clear whether any reaches the uterus without sperm involvement. Thus, models based on SP infusion directly into the uterus (Odhiambo et al., 2009; Ortiz et al., 2019) might not be representative of the events that take place physiologically. For this reason, in the current study, a model based on mating heifers to vasectomized bulls was used.
In addition to having an effect on the endometrium, studies in both mice and pigs have reported influences of SP on the ovary (Gangnuss et al., 2004; O’Leary et al., 2006). Exposure to SP (through mating in the mouse, or infusion into the uterus in the pig) led to an increase in macrophage recruitment into the ovulatory follicle (Gangnuss et al., 2004; O’Leary et al., 2006). In the pig, this was associated with an increase in CL weight at Days 5–9, which probably explains the increase in P4 secretion that was also observed at this time (O’Leary et al., 2006). Although there is inconsistent evidence on the effect of high P4 on embryo survival in pigs, with some authors reporting a positive effect (Ashworth, 1991; Jindal et al., 1997), others a negative (Mao and Foxcroft, 1998), and others a lack of effect (Muro et al., 2019), P4 prevents embryo resorption (Aisemberg et al., 2013) and is essential for timely progression of early embryogenesis (Zhang and Murphy, 2014) in mice. In cattle, elevated P4 concentrations prior to Day 7 are associated with an altered endometrial transcriptome (Forde et al., 2009) and accelerated conceptus development (Clemente et al., 2009; O’Hara et al., 2014a). Thus, increased P4 output could be one mechanism through which SP induces an increase in embryo survival in this species. However, in the present study, no differences in CL volume at Days 7 and 14, nor in Day 14 CL weight were observed between treatments.
Exposure to SP at the time of mating had no effect on embryo survival to Day 14 following transfer on Day 7. Recovery rate is related to conceptus survival, as those who die degenerate and are not recovered on Day 14. This is consistent with the studies that indicate that SP infusion into the uterus does not lead to improved pregnancy rates in cattle (Odhiambo et al., 2009; Pfeiffer et al., 2012; Ortiz et al., 2019), and contrasts with some literature available in other species where exposure to SP leads to improved embryo survival and early embryo development (Johansson et al., 2004; O’Leary et al., 2004; Bromfield et al., 2014). Despite the lack of differences regarding embryo survival, filamentous conceptuses recovered from heifers that had been mated were longer than those recovered from control heifers. As already mentioned, large variation in conceptus size recovered from the same recipient is typically observed when multiple embryo transfer is carried out (O’Hara et al., 2014b; Barnwell et al., 2016) and is also seen after insemination in single ovulating cows (Ribeiro et al., 2016). Nevertheless, when conceptuses were grouped according to morphology (thus, reducing variation), filamentous conceptuses recovered from mated heifers were longer than their control counterparts. It is not clear whether an increase in conceptus length on as given day is a positive or negative phenomenon. On the one hand, a higher number of trophectoderm cells will ultimately lead to an increase in the secretion of IFNT, the maternal recognition signal in cattle. Maternal recognition of pregnancy in this species takes place around Day 16 (Sánchez et al., 2018). At this time, conceptuses that are not able to produce sufficient amounts of IFNT will be lost due to their inability to prevent luteolysis. On the other hand, asynchronous transfer of embryos, in which a Day 7 embryo is transferred to a Day 9, results in higher conceptus length but this does not translate into higher pregnancy rates (Randi et al., 2016).
The success of embryo transfer (in the absence of exposure of the reproductive tract of the recipient to either sperm or SP) in many livestock species, where pregnancy rates are comparable to those achieved with artificial insemination (Drost et al., 1999; Sartori et al., 2006), indicates that exposure to SP is not essential for pregnancy. However, as mentioned above, this factor does seem to have an impact on embryo and offspring metabolism and overall health. Having observed differences in conceptus length between embryos developing in an environment that had been exposed to SP or not, the next aim was to determine whether this difference in size was accompanied by a difference in development stage. Because morphology and size might not be representative of the developmental stage of the conceptus, a panel of genes previously reported to be markers of gastrulation stage (Degrelle et al., 2011) was evaluated. However, in the present study, the expression profiles described by Degrelle et al. (2011) were not observed, but we did detect differences in the expression of these genes between morphologies. In both groups, filamentous conceptuses exhibited the highest expression of IL6, and the lowest expression of JSP1. This expression pattern is consistent with prior studies, who detected upregulation of IL6 and downregulation of JSP1 in Day 15 bovine long conceptuses (measuring 24.7 ± 1.9) in comparison to short conceptuses (measuring 4.2 ± 0.1; Barnwell et al., 2016). On the other hand, in the present study, control filamentous conceptuses exhibited the highest CITED1 relative expression, while the opposite was true in conceptuses recovered from mated heifers. In a study by Mamo et al. (2011), Days 16 and 19 bovine conceptuses had higher expression of CITED2 (an important paralog of CITED1) than Days 7, 10 and 13 conceptuses, seemingly agreeing with our control group. HNRNPDL and TGFB3 relative abundance also presented different pattern between conceptuses recovered from mated or unmated animals. While control filamentous embryos had the highest relative expression of both genes, the morphology-matched conceptuses in the vasectomized group has the lowest expression.
In addition, CALM1, DLD, and FURIN relative expression was lowest in filamentous conceptuses, whereas PTGS2 was highest, only in the control group. In accordance with our results, PTGS2 expression has previously been shown to be upregulated in Day 15 long conceptuses in comparison to age-matched short conceptuses (Barnwell et al., 2016), and in Days 16 and 19 in comparison to Days 7, 10, and 13 conceptuses (Mamo et al., 2011). However, FURIN expression was reported to be also upregulated in Days 16 and 19 in comparison to Days 7, 10, and 13 conceptuses (Mamo et al., 2011), in disagreement to our findings.
Although the pattern described by Degrelle et al. (2011) did not allow us to determine the gastrulation stage of our conceptuses, these marker genes are involved in different pathways important to embryo survival and development. Thus, the panel of genes was supplemented with additional ones in order to describe the effect of the SP-exposed environment on conceptus apoptosis, metabolism, development, and immunology. Most of the differences between treatments detected in gene expression were observed when comparing long filamentous conceptuses. Indeed, conceptuses exhibiting this morphology had different CALM1, CITED1, DLD, HNRNPDL, PTGS2, and TGFB3 expression levels depending on whether they developed in a mated or unmated heifer. Interestingly, in the control group, expression levels of these genes differed between short and long conceptuses, whereas this behavior was not detected in the conceptuses from the vasectomized group. This hints at changes in the regulation of different pathways occurring in very large embryos, which is altered by a different uterine environment elicited by SP exposure.
The apoptosis process was evaluated by analyzing HNRNPDL and CASP3 relative expression. Lower levels of HNRNPDL relative expression were observed in long filamentous embryos from the SP-exposed group than in the control. HNRNPDL encodes for the heterogeneous nuclear ribonucleoprotein D Like (hnRNPDL), a RNA-binding protein which binds heterogeneous nuclear RNA (hnRNA) to regulate pre-mRNA in the nucleus (Geuens et al., 2016). When hnRNPDL binds the specific mRNA, it induces the decay of the molecule (Fialcowitz et al., 2005) and, for this reason, it is considered to repress the gene expression of its targets. One of its potential targets is the Cell Division Cycle and Apoptosis Regulator 1 (CCAR1) (Li et al., 2019), which acts as a key intracellular transducer for apoptosis (Rishi et al., 2003). In addition, hnRNPDL also regulates the expression of cytochrome C oxidase subunit 5B (COX5B), a mitochondrial energy-generating enzyme critical for the proper functioning of cells. The disruption of its expression by hnRNPDL may result in the cease of ATP generation and, therefore, the induction of apoptosis (Safavizadeh et al., 2012). Thus, a lower expression of HNRNPDL in the embryos could indicate lower levels of apoptosis. However, CASP3, a gene that codes for one of the proteases that initiates the execution pathway of apoptosis (intrinsic and the extrinsic apoptotic pathways diverge), did not differ between treatments. This suggests that SP-induced changes of HNRNPDL do not relate to apoptotic pathways in the conceptus and, therefore, its biological meaning remains unclear.
Conceptus metabolism was assessed by evaluating the relative expression of DLD, GSTM1, and PTGS2. DLD and PTGS2 relative expression was higher in long filamentous or tubular embryos recovered from mated heifers than unmated heifers. DLD encodes for the mitochondrial dihydrolipoamide dehydrogenase, a member of the class-I pyridine nucleotide-disulfide oxidoreductase family crucial for embryo energy production (Leese, 1991; Johnson et al., 2009). Moreover, this enzyme seems to be essential for preimplantation embryos as DLD knockout mice embryos are unable to undergo gastrulation (Johnson et al., 1997). On the other hand, PTGS2 is the key enzyme in prostaglandin biosynthesis, which may mediate the effects of progesterone and IFNT in the endometrium and is highly expressed in the trophectoderm of ovine (Charpigny et al., 1997), bovine (Barnwell et al., 2016), porcine (Blomberg et al., 2006), and murine (Lim et al., 1999) embryos. The importance of this enzyme in embryo development is highlighted by the fact that PTGS2 is downregulated in both in vivo- and in vitro-produced embryos that result in no pregnancy (El-Sayed et al., 2006; Ghanem et al., 2011). Considering both genes, it seems that SP may have an impact on the development in critical embryo stages such as tubular and filamentous embryos.
The relative expression of CALM1, CITED1, FURIN, and TGFB3, genes related to embryo development, were also evaluated. An effect of SP treatment in the long filamentous conceptuses for CALM1, CITED1, and TGFB3 was observed. CALM1 was the only one of these gene in which the relative expression increased in the long filamentous conceptuses recovered from mated heifers compared to control. On the other hand, both CITED1 and TGFB3 exhibited lower relative expression in conceptuses developing in a SP-primed environment in comparison to the control. CALM1 encodes calmodulin 1, a calcium binding protein which represents the major calcium sensor in eukaryotes. CALM1 has been associated to the morphogenesis process for the development of the body plan during gastrulation in response to global calcium waves (Slusarski and Pelegri, 2007), the early development of the neural system (Seto-Ohshima et al., 1987) and hematopoiesis (Kitsos et al., 2005). The participation of TFGB3 in embryogenesis can be related to its role in the epithelial-mesenchymal transitions (EMT), which enables cell movement and morphogenesis (Zavadil and Böttinger, 2005). During gastrulation, EMT is observed in the generation of the primitive mesoderm, the cell migration into the primitive node and the establishment of the three embryonic layers (Blomberg et al., 2008; Dimitrova et al., 2017). CITED1 (or MSG1), which encodes for the transcriptional factor Cbp/p300-interacting transactivator 1, has been described to be involved during embryogenesis (Dunwoodie et al., 1998; Gerstner and Landry, 2007) and placentation (Rodriguez et al., 2004; Sparrow et al., 2009) in mice. In summary, the lower expression of CITED1 and TGFB3, together with higher levels of CALM1, suggest that long filamentous conceptus in the mated group may be at a later stage of gastrulation than morphology-matched control conceptuses. Finally, the relative expression of IL6 and MHC-I (or JSP1), two immune system related genes, was also evaluated, but no differences between treatments were observed.
To the best of our knowledge, this is the first study describing the effects of SP (as assessed by comparing unmated controls with heifers mated to vasectomized bulls) on the CL and early embryo development in cattle. The weight of evidence suggests that SP does not play a crucial role in embryo development in cattle as: (1) it is not clear whether SP reaches the uterus in bovine; (2) SP has been described to have a negative effect on endometrial RNA integrity in vitro (Fernandez-Fuertes et al., 2019); and (3) there is no evidence of an effect of SP exposure on pregnancy rates (Odhiambo et al., 2009; Pfeiffer et al., 2012; Ortiz et al., 2019). On the other hand, the embryo-related changes reported in the present work suggest that exposure to SP during natural mating changes the environment in which embryos develop from Day 7 onward. However, it is not clear whether these changes may be driven directly by the female reproductive tract or by an earlier CL maturation. Thus, further research should be conducted to elucidate the exact mechanism by which SP may improve embryo development.
Data Availability Statement
The datasets generated for this study are available on request to the corresponding author.
Ethics Statement
All experimental procedures involving animals were approved by the Animal Research Ethics Committee of University College Dublin, Ireland, and the Universitat de Girona, Spain, and licensed by the Health Products Regulatory Authority (HPRA), Ireland, in accordance with Statutory Instrument No. 543 of 2012 (under Directive 2010/63/EU on the Protection of Animals used for Scientific Purposes).
Author Contributions
YM-O carried out the laboratory work, analyzed results and wrote the draft. JS, SR, SB-A, MM, and DK carried out the animal work, including handling of bulls and heifers, estrus detection, embryo transfer and recovery. MY performed statistical analysis of the data. MY, PL, JS, and BF-F contributed to the critical revision of the manuscript.
Funding
This work was supported by the European Union’s Horizon 2020 Research and Innovation Program (Grant Agreement No. 792212) and Science Foundation Ireland (Grant No. 16/IA/4474).
Conflict of Interest
The authors declare that the research was conducted in the absence of any commercial or financial relationships that could be construed as a potential conflict of interest.
Acknowledgments
We would like to thank Mary Wade and Clio Maicas for their help in producing the in vitro embryos used in this study. In addition, we would also like to thank Pol Fernández-López for his input in the statistical analysis and elaboration of charts.
Footnotes
References
Adams, G. P., and Ratto, M. H. (2013). Ovulation-inducing factor in seminal plasma: a review. Anim. Reprod. Sci. 136, 148–156. doi: 10.1016/j.anireprosci.2012.10.004
Aisemberg, J., Vercelli, C. A., Bariani, M. V., Billi, S. C., Wolfson, M. L., and Franchi, A. M. (2013). Progesterone is essential for protecting against LPS-induced pregnancy loss. LIF as a potential mediator of the anti-inflammatory effect of progesterone. PLoS One 8:56161. doi: 10.1371/journal.pone.0056161
Ashworth, C. J. (1991). Effect of pre-mating nutritional status and post-mating progesterone supplementation on embryo survival and conceptus growth in gilts. Anim. Reprod. Sci. 26, 311–321. doi: 10.1016/0378-4320(91)90056-6
Badrakh, Y., Yanagawa, Y., Nagano, M., and Katagiri, S. (2020). Effect of seminal plasma infusion into the vagina on the normalization of endometrial epidermal growth factor concentrations and fertility in repeat breeder dairy cows. J. Reprod. Dev. 66, 149–154. doi: 10.1262/jrd.2019-148
Barnwell, C. V., Farin, P. W., Ashwell, C. M., Farmer, W. T., Galphin, S. P., and Farin, C. E. (2016). Differences in mRNA populations of short and long bovine conceptuses on Day 15 of gestation. Mol. Reprod. Dev. 83, 424–441. doi: 10.1002/mrd.22640
Bedford, J. M. (2015). The functions - or not - of seminal plasma? Biol. Reprod. 92:18. doi: 10.1095/biolreprod.114.126045
Betteridge, K. J., Eaglesome, M. D., Randall, G. C. B., and Mitchell, D. (1980). Collection, description and transfer of embryos from cattle 10–16 days after oestrus. Reproduction 59, 205–216. doi: 10.1530/jrf.0.0590205
Blomberg, L. A., Garrett, W. M., Guillomot, M., Miles, J. R., Sonstegard, T. S., Van Tassell, C. P., et al. (2006). Transcriptome profiling of the tubular porcine conceptus identifies the differential regulation of growth and developmentally associated genes. Mol. Reprod. Dev. 1491–1502. doi: 10.1002/mrd
Blomberg, L. A., Hashizume, K., and Viebahn, C. (2008). Blastocyst elongation, trophoblastic differentiation, and embryonic pattern formation. Reproduction 135, 181–195. doi: 10.1530/REP-07-0355
Bravo, P. W., and Diaz, D. (2010). Effect of the reproductive state of female alpacas on embryonic mortality rate. Am. J. Vet. Res. 71, 1096–1099. doi: 10.2460/ajvr.71.9.1096
Bromfield, J. J. (2016). A role for seminal plasma in modulating pregnancy outcomes in domestic species. Reproduction 152, R223–R232. doi: 10.1530/REP-16-0313
Bromfield, J. J., Schjenken, J. E., Chin, P. Y., Care, A. S., Jasper, M. J., and Robertson, S. A. (2014). Maternal tract factors contribute to paternal seminal fluid impact on metabolic phenotype in offspring. Proc. Natl. Acad. Sci. U.S.A. 111, 2200–2205. doi: 10.1073/pnas.1305609111
Charpigny, G., Reinaud, P., Tamby, J.-P., Créminon, C., and Guillomot, M. (1997). Cyclooxygenase-2 unlike cyclooxygenase-1 is highly expressed in ovine embryos during the implantation period1. Biol. Reprod. 57, 1032–1040. doi: 10.1095/biolreprod57.5.1032
Chen, J. C., Johnson, B. A., Erikson, D. W., Piltonen, T. T., Barragan, F., Chu, S., et al. (2014). Seminal plasma induces global transcriptomic changes associated with cell migration, proliferation and viability in endometrial epithelial cells and stromal fibroblasts. Hum. Reprod. 29, 1255–1270. doi: 10.1093/humrep/deu047
Clemente, M., De La Fuente, J., Fair, T., Al Naib, A., Gutierrez-Adan, A., Roche, J. F., et al. (2009). Progesterone and conceptus elongation in cattle: a direct effect on the embryo or an indirect effect via the endometrium? Reproduction 138, 507–517. doi: 10.1530/REP-09-0152
Crawford, G., Ray, A., Gudi, A., Shah, A., and Homburg, R. (2015). The role of seminal plasma for improved outcomes during in vitro fertilization treatment: review of the literature and meta-analysis. Hum. Reprod. Update 21, 275–284. doi: 10.1093/humupd/dmu052
Dean, M. D., Findlay, G. D., Hoopmann, M. R., Wu, C. C., MacCoss, M. J., Swanson, W. J., et al. (2011). Identification of ejaculated proteins in the house mouse (Mus domesticus) via isotopic labeling. BMC Genomics 12:306. doi: 10.1186/1471-2164-12-306
Degrelle, S. A., LêCao, K. A., Heyman, Y., Everts, R. E., Campion, E., Richard, C., et al. (2011). A small set of extra-embryonic genes defines a new landmark for bovine embryo staging. Reproduction 141, 79–89. doi: 10.1530/REP-10-0174
Dimitrova, Y., Gruber, A. J., Mittal, N., Ghosh, S., Dimitriades, B., Mathow, D., et al. (2017). TFAP2A is a component of the ZEB1/2 network that regulates TGFB1-induced epithelial to mesenchymal transition. Biol. Direct 12:8. doi: 10.1186/s13062-017-0180-7
Drost, M., Ambrose, J. D., Thatcher, M.-J., Cantrell, C. K., Wolfsdorf, K. E., Hasler, J. F., et al. (1999). Conception rates after artificial insemination or embryo transfer in lactating dairy cows during summer in Florida. Theriogenology 52, 1161–1167. doi: 10.1016/S0093-691X(99)00208-3
Druart, X., Rickard, J. P., Mactier, S., Kohnke, P. L., Kershaw-Young, C. M., Bathgate, R., et al. (2013). Proteomic characterization and cross species comparison of mammalian seminal plasma. J. Proteomics 91, 13–22. doi: 10.1016/j.jprot.2013.05.029
Dunwoodie, S. L., Rodriguez, T. A., and Beddington, R. S. P. (1998). Msg1 and mrg1, founding members of a gene family, show distinct patterns of gene expression during mouse embryogenesis. Mech. Dev. 72, 27–40. doi: 10.1016/S0925-4773(98)00011-2
El-Sayed, A., Hoelker, M., Rings, F., Salilew, D., Jennen, D., Tholen, E., et al. (2006). Large-scale transcriptional analysis of bovine embryo biopsies in relation to pregnancy success after transfer to recipients. Physiol. Genomics 28, 84–96. doi: 10.1152/physiolgenomics.00111.2006
Elweza, A. E., Ezz, M. A., Acosta, T. J., Talukder, A. K., Shimizu, T., Hayakawa, H., et al. (2018). A proinflammatory response of bovine endometrial epithelial cells to active sperm in vitro. Mol. Reprod. Dev. 85, 215–226. doi: 10.1002/mrd.22955
Ezz, M. A., Marey, M. A., Elweza, A. E., Kawai, T., Heppelmann, M., Pfarrer, C., et al. (2019). TLR2/4 signaling pathway mediates sperm-induced inflammation in bovine endometrial epithelial cells in vitro. PLoS One 14:e0214516. doi: 10.1371/journal.pone.0214516
Fazeli, A., Affara, N. A., Hubank, M., and Holt, W. V. (2004). Sperm-induced modification of the oviductal gene expression profile after natural insemination in mice. Biol. Reprod. 71, 60–65. doi: 10.1095/biolreprod.103.026815
Fernandez-Fuertes, B., Sánchez, J. M., Bagés-Arnal, S., McDonald, M., Yeste, M., and Lonergan, P. (2019). Species-specific and collection method-dependent differences in endometrial susceptibility to seminal plasma-induced RNA degradation. Sci. Rep. 9:15072. doi: 10.1038/s41598-019-51413-4
Fialcowitz, E. J., Brewer, B. Y., Keenan, B. P., and Wilson, G. M. (2005). A hairpin-like structure within an AU-rich mRNA-destabilizing element regulates trans-factor binding selectivity and mRNA decay kinetics. J. Biol. Chem. 280, 22406–22417. doi: 10.1074/jbc.M500618200
Fleming, T. P., Watkins, A. J., Velazquez, M. A., Mathers, J. C., Prentice, A. M., Stephenson, J., et al. (2018). Origins of lifetime health around the time of conception: causes and consequences. Lancet 391, 1842–1852. doi: 10.1016/S0140-6736(18)30312-X
Forde, N., Carter, F., Fair, T., Crowe, M. A., Evans, A. C. O., Spencer, T. E., et al. (2009). Progesterone-regulated changes in endometrial gene expression contribute to advanced conceptus development in cattle. Biol. Reprod. 81, 784–794. doi: 10.1095/biolreprod.108.074336
Gangnuss, S., Sutton-McDowall, M. L., Robertson, S. A., and Armstrong, D. T. (2004). Seminal plasma regulates corpora Lutea macrophage populations during early pregnancy in mice1. Biol. Reprod. 71, 1135–1141. doi: 10.1095/biolreprod.104.027425
Gerstner, J. R., and Landry, C. F. (2007). Expression of the transcriptional coactivator CITED1 in the adult and developing murine brain. Dev. Neurosci. 29, 203–212. doi: 10.1159/000096389
Geuens, T., Bouhy, D., and Timmerman, V. (2016). The hnRNP family: insights into their role in health and disease. Hum. Genet. 135, 851–867. doi: 10.1007/s00439-016-1683-5
Ghanem, N., Salilew-Wondim, D., Gad, A., Tesfaye, D., Phatsara, C., Tholen, E., et al. (2011). Bovine blastocysts with developmental competence to term share similar expression of developmentally important genes although derived from different culture environments. Reproduction 142, 551–564. doi: 10.1530/REP-10-0476
Gonçalves, R. F., Chapman, D. A., Bertolla, R. P., Eder, I., and Killian, G. J. (2008). Pre-treatment of cattle semen or oocytes with purified milk osteopontin affects in vitro fertilization and embryo development. Anim. Reprod. Sci. 108, 375–383. doi: 10.1016/j.anireprosci.2007.09.006
Grygar, I., Kudláč, E., Doležel, R., and Nedbálková, J. (1997). Volume of luteal tissue and concentration of serum progesterone in cows bearing homogeneous corpus luteum or corpus luteum with cavity. Anim. Reprod. Sci. 49, 77–82. doi: 10.1016/S0378-4320(97)00027-4
Guerin, L. R., Moldenhauer, L. M., Prins, J. R., Bromfield, J. J., Hayball, J. D., and Robertson, S. A. (2011). Seminal fluid regulates accumulation of FOXP3+ regulatory T cells in the preimplantation mouse uterus through expanding the FOXP3+ cell pool and CCL19-mediated recruitment1. Biol. Reprod. 85, 397–408. doi: 10.1095/biolreprod.110.088591
Hanson, M. A., and Gluckman, P. D. (2014). Early developmental conditioning of later health and disease: physiology or pathophysiology? Physiol. Rev. 94, 1027–1076. doi: 10.1152/physrev.00029.2013
Hao, Y., Murphy, C., Spate, L., Wax, D., Zhong, Z., Samuel, M., et al. (2008). Osteopontin improves in vitro development of Porcina embryos and decreases apoptosis. Mol. Reprod. Dev. 75, 291–298. doi: 10.1002/mrd.20794
Hawk, H. W. (1983). Sperm survival and transport in the female reproductive tract. J. Dairy Sci. 66, 2645–2660. doi: 10.3168/jds.S0022-0302(83)82138-9
Hunt, J., and Robertson, S. A. (1996). Uterine macrophages and environmental programming for pregnancy success. J. Reprod. Immunol. 32, 1–25. doi: 10.1016/S0165-0378(96)88352-5
Hunter, R. H. F. (1981). Sperm transport and reservoirs in the pig oviduct in relation to the time of ovulation. J. Reprod. Fertil. 63, 109–117. doi: 10.1530/jrf.0.0630109
Ibrahim, L. A., Rizo, J. A., Fontes, P. L. P., Lamb, G. C., and Bromfield, J. J. (2019). Seminal plasma modulates expression of endometrial inflammatory meditators in the bovine. Biol. Reprod. 100, 660–671. doi: 10.1093/biolre/ioy226
Inskeep, E. K. (2004). Preovulatory, postovulatory, and postmaternal recognition effects of concentrations of progesterone on embryonic survival in the cow. J. Anim. Sci. 82(E–Suppl.), 24–39. doi: 10.2527/2004.8213_supple24x
International Embryo Technology Society (2009). Manual of the International Embryo Technology Society? A Procedural Guide and General Information for the Use of Embryo Transfer Technology Emphasizing Sanitary Procedures. Available online at: https://www.iets.org/pub_manual.asp doi: 10.2527/2004.8213_supplE24x (accessed February 02, 2019).
Jindal, R., Cosgrove, J. R., and Foxcroft, G. R. (1997). Progesterone mediates nutritionally induced effects on embryonic survival in gilts. J. Anim. Sci. 75, 1063–1070. doi: 10.2527/1997.7541063x
Johansson, M., Bromfield, J. J., Jasper, M. J., and Robertson, S. A. (2004). Semen activates the female immune response during early pregnancy in mice. Immunology 112, 290–300. doi: 10.1111/j.1365-2567.2004.01876.x
Johnson, M. T., Vang, P., Filipovits, J., and Gardner, D. K. (2009). Maternal enzyme masks the phenotype of mouse embryos lacking A dehydrogenase. Reprod. Biomed. Online 19, 79–88. doi: 10.1016/S1472-6483(10)60050-8
Johnson, M. T., Yang, H.-S., Magnuson, T., and Patel, M. S. (1997). Targeted disruption of the murine dihydrolipoamide dehydrogenase gene (Dld) results in perigastrulation lethality. Proc. Natl. Acad. Sci. U.S.A. 94, 14512–14517. doi: 10.1073/pnas.94.26.14512
Kitsos, C. M., Sankar, U., Illario, M., Colomer-Font, J. M., Duncan, A. W., Ribar, T. J., et al. (2005). Calmodulin-dependent protein kinase IV regulates hematopoietic stem cell maintenance. J. Biol. Chem. 280, 33101–33108. doi: 10.1074/jbc.M505208200
Kleemann, D. O., Walker, S. K., and Seamark, R. F. (1994). Enhanced fetal growth in sheep administered progesterone during the first three days of pregnancy. J. Reprod. Fertil. 102, 411–417. doi: 10.1530/jrf.0.1020411
Leese, H. J. (1991). Metabolism of the preimplantation mammalian embryo. Oxf. Rev. Reprod. Biol. 13, 35–72.
Lefebvre, J., Fan, J., Chevalier, S., Sullivan, R., Carmona, E., and Manjunath, P. (2007). Genomic structure and tissue-specific expression of human and mouse genes encoding homologues of the major bovine seminal plasma proteins. Mol. Hum. Reprod. 13, 45–53. doi: 10.1093/molehr/gal098
Li, R. Z., Hou, J., Wei, Y., Luo, X., Ye, Y., and Zhang, Y. (2019). hnRNPDL extensively regulates transcription and alternative splicing. Gene 687, 125–134. doi: 10.1016/j.gene.2018.11.026
Lim, H., Gupta, R. A., Ma, W. G., Paria, B. C., Moller, D. E., Morrow, J. D., et al. (1999). Cyclo-oxygenase-2-derived prostacyclin mediates embryo implantation in the mouse via PPARδ. Genes Dev. 13, 1561–1574. doi: 10.1101/gad.13.12.1561
Livak, K. J., and Schmittgen, T. D. (2001). Analysis of relative gene expression data using real-time quantitative PCR and the 2-ΔΔCT method. Methods 25, 402–408. doi: 10.1006/meth.2001.1262
Mamo, S., Mehta, J. P., McGettigan, P., Fair, T., Spencer, T. E., Bazer, F. W., et al. (2011). RNA sequencing reveals novel gene clusters in bovine conceptuses associated with maternal recognition of pregnancy and implantation1. Biol. Reprod. 85, 1143–1151. doi: 10.1095/biolreprod.111.092643
Mao, J., and Foxcroft, G. R. (1998). Progesterone therapy during early pregnancy and embryonal survival in primiparous weaned sows. J. Anim. Sci. 76, 1922–1928. doi: 10.2527/1998.7671922x
Moldenhauer, L. M., Keenihan, S. N., Hayball, J. D., and Robertson, S. A. (2010). GM-CSF is an essential regulator of t cell activation competence in uterine dendritic cells during early pregnancy in mice. J. Immunol. 185, 7085–7096. doi: 10.4049/jimmunol.1001374
Monaco, E., Gasparrini, B., Boccia, L., De Rosa, A., Attanasio, L., Zicarelli, L., et al. (2009). Effect of osteopontin (OPN) on in vitro embryo development in cattle. Theriogenology 71, 450–457. doi: 10.1016/j.theriogenology.2008.08.012
Morgan, H. L., Paganopoulou, P., Akhtar, S., Urquhart, N., Philomin, R., Dickinson, Y., et al. (2020). Paternal diet impairs F1 and F2 offspring vascular function through sperm and seminal plasma specific mechanisms in mice. J. Physiol. 598, 699–715. doi: 10.1113/JP278270
Morgan, H. L., and Watkins, A. J. (2019). The influence of seminal plasma on offspring development and health. Semin. Cell Dev. Biol. 97, 131–137. doi: 10.1016/j.semcdb.2019.06.008
Muro, B. B. D., Carnevale, R. F., Leal, D. F., Torres, M. A., Mendonça, M. V., Nakasone, D. H., et al. (2019). Supplemental progesterone during early pregnancy exerts divergent responses on embryonic characteristics in sows and gilts. Animal 1–7. doi: 10.1017/S1751731119002982 [Epub ahead of print].
O, W. S., Chen, H. Q., and Chow, P. H. (1988). Effects of male accessory sex gland secretions on early embryonic development in the golden hamster. J. Reprod. Fertil. 84, 341–344. doi: 10.1530/jrf.0.0840341
Odhiambo, J. F., Poole, D. H., Hughes, L., DeJarnette, J. M., Inskeep, E. K., and Dailey, R. A. (2009). Pregnancy outcome in dairy and beef cattle after artificial insemination and treatment with seminal plasma or transforming growth factor beta-1. Theriogenology 72, 566–571. doi: 10.1016/j.theriogenology.2009.04.013
O’Hara, L., Forde, N., Carter, F., Rizos, D., Maillo, V., Ealy, A. D., et al. (2014a). Paradoxical effect of supplementary progesterone between day 3 and day 7 on corpus luteum function and conceptus development in cattle. Reprod. Fertil. Dev. 26, 328–336. doi: 10.1071/RD12370
O’Hara, L., Forde, N., Kelly, A. K., and Lonergan, P. (2014b). Effect of bovine blastocyst size at embryo transfer on day 7 on conceptus length on day 14: Can supplementary progesterone rescue small embryos? Theriogenology 81, 1123–1128. doi: 10.1016/j.theriogenology.2014.01.041
O’Leary, S., Jasper, M. J., Robertson, S. A., and Armstrong, D. T. (2006). Seminal plasma regulates ovarian progesterone production, leukocyte recruitment and follicular cell responses in the pig. Reproduction 132, 147–158. doi: 10.1530/rep.1.01119
O’Leary, S., Jasper, M. J., Warnes, G. M., Armstrong, D. T., and Robertson, S. A. (2004). Seminal plasma regulates endometrial cytokine expression, leukocyte recruitment and embryo development in the pig. Reproduction 128, 237–247. doi: 10.1530/rep.1.00160
Ortiz, W. G., Rizo, J. A., Carvalheira, L. R., Ahmed, B. M. S., Estrada-Cortes, E., Harstine, B. R., et al. (2019). Effects of intrauterine infusion of seminal plasma at artificial insemination on fertility of lactating Holstein cows. J. Dairy Sci. 102, 6587–6594. doi: 10.3168/jds.2019-16251
Parr, M. H., Scully, S., Lonergan, P., Evans, A. C. O., Crowe, M. A., and Diskin, M. G. (2017). Establishment of critical timing of progesterone supplementation on corpus luteum and embryo development in beef heifers. Anim. Reprod. Sci. 180, 1–9. doi: 10.1016/j.anireprosci.2017.02.005
Passaro, C., Tutt, D., Bagés-Arnal, S., Maicas, C., Laguna-Barraza, R., Gutierrez-Adán, A., et al. (2019). Global transcriptomic response of bovine endometrium to blastocyst-stage embryos. Reproduction 158, 223–235. doi: 10.1530/REP-19-0064
Passaro, C., Tutt, D., Mathew, D. J., Sanchez, J. M., Browne, J. A., Boe-Hansen, G. B., et al. (2018). Blastocyst-induced changes in the bovine endometrial transcriptome. Reproduction 156, 219–229. doi: 10.1530/REP-18-0188
Pfeiffer, K. E., Binversie, J. A., Rhinehart, J. D., and Larson, J. E. (2012). Exposure of beef females to the biostimulatory effects of bulls with or without deposition of seminal plasma prior to AI. Anim. Reprod. Sci. 133, 27–34. doi: 10.1016/j.anireprosci.2012.06.011
Pini, T., Leahy, T., Soleilhavoup, C., Tsikis, G., Labas, V., Combes-Soia, L., et al. (2016). Proteomic investigation of ram spermatozoa and the proteins conferred by seminal plasma. J. Proteome Res. 15, 3700–3711. doi: 10.1021/acs.jproteome.6b00530
Randi, F., Fernandez-Fuertes, B., Mcdonald, M., Forde, N., Kelly, A. K., Bastos Amorin, H., et al. (2016). Asynchronous embryo transfer as a tool to understand embryo-uterine interaction in cattle: Is a large conceptus a good thing? Reprod. Fertil. Dev. 28, 1999–2006. doi: 10.1071/RD15195
Ratto, M. H., Berland, M., Silva, M. E., and Adams, G. P. (2019). New insights of the role of b-NGF in the ovulation mechanism of induced ovulating species. Reprodution 157, 199–207. doi: 10.1530/REP-18-0305
Ratto, M. H., Leduc, Y. A., Valderrama, X. P., Van Straaten, K. E., Delbaere, L. T. J., Pierson, R. A., et al. (2012). The nerve of ovulation-inducing factor in semen. Proc. Natl. Acad. Sci. U.S.A. 109, 15042–15047. doi: 10.1073/pnas.1206273109
Rego, J. P. A., Moura, A. A., Nouwens, A. S., McGowan, M. R., and Boe-Hansen, G. B. (2015). Seminal plasma protein profiles of ejaculates obtained by internal artificial vagina and electroejaculation in Brahman bulls. Anim. Reprod. Sci. 160, 126–137. doi: 10.1016/j.anireprosci.2015.07.015
Ribeiro, E. S., Greco, L. F., Bisinotto, R. S., Lima, F. S., Thatcher, W. W., and Santos, J. E. (2016). Biology of preimplantation conceptus at the onset of elongation in dairy cows. Biol. Reprod. 94, 1–18. doi: 10.1095/biolreprod.115.134908
Rishi, A. K., Zhang, L., Boyanapalli, M., Wali, A., Mohammad, R. M., Yu, Y., et al. (2003). Identification and characterization of a cell cycle and apoptosis regulatory protein-1 as a novel mediator of apoptosis signaling by retinoid CD437. J. Biol. Chem. 278, 33422–33435. doi: 10.1074/jbc.M303173200
Robertson, S. A. (2007). Seminal fluid signaling in the female reproductive tract: lessons from rodents and pigs. J. Anim. Sci. 85, 36–44. doi: 10.2527/jas.2006-578
Robertson, S. A., Care, A. S., and Moldenhauer, L. M. (2018). Regulatory T cells in embryo implantation and the immune response to pregnancy. J. Clin. Invest. 128, 4224–4235. doi: 10.1172/JCI122182
Robertson, S. A., Guerin, L. R., Bromfield, J. J., Branson, K. M., Ahlström, A. C., and Care, A. S. (2009). Seminal fluid drives expansion of the CD4+CD25+ T regulatory cell pool and induces tolerance to paternal alloantigens in mice1. Biol. Reprod. 80, 1036–1045. doi: 10.1095/biolreprod.108.074658
Robertson, S. A., Mau, V. J., Hudson, S. N., and Tremellen, K. P. (1997). Cytokine-leukocyte networks and the establishment of pregnancy. Am. J. Reprod. Immunol. 37, 438–442. doi: 10.1111/j.1600-0897.1997.tb00257.x
Robertson, S. A., Prins, J. R., Sharkey, D. J., and Moldenhauer, L. M. (2013). Seminal fluid and the generation of regulatory T cells for embryo implantation. Am. J. Reprod. Immunol. 69, 315–330. doi: 10.1111/aji.12107
Rodger, J. C. (1976). Comparative aspects of the accessory sex glands and seminal biochemistry of mammals. Comp. Biochem. Physiol. Part B Biochem. 55, 1–8. doi: 10.1016/0305-0491(76)90164-4
Rodriguez, T. A., Sparrow, D. B., Scott, A. N., Withington, S. L., Preis, J. I., Michalicek, J., et al. (2004). Cited1 is required in trophoblasts for placental development and for embryo growth and survival. Mol. Cell. Biol. 24, 228–244. doi: 10.1128/mcb.24.1.228-244.2004
Rodríguez-Villamil, P., Hoyos-Marulanda, V., Martins, J. A. M., Oliveira, A. N., Aguiar, L. H., Moreno, F. B., et al. (2016). Purification of binder of sperm protein 1 (BSP1) and its effects on bovine in vitro embryo development after fertilization with ejaculated and epididymal sperm. Theriogenology 85, 540–554. doi: 10.1016/j.theriogenology.2015.09.044
Safavizadeh, N., Rahmani, S. A., and Zaefizadeh, M. (2012). COX5B and COX2 gene expressions in multiple sclerosis. J. Cell Mol. Biol. 10, 21–30. doi: 10.4103/0971-6866.112879
Sánchez, J. M., Mathew, D. J., Passaro, C., Fair, T., and Lonergan, P. (2018). Embryonic maternal interaction in cattle and its relationship with fertility. Reprod. Domest. Anim. 53, 20–27. doi: 10.1111/rda.13297
Sartori, R., Gümen, A., Guenther, J. N., Souza, A. H., Caraviello, D. Z., and Wiltbank, M. C. (2006). Comparison of artificial insemination versus embryo transfer in lactating dairy cows. Theriogenology 65, 1311–1321. doi: 10.1016/j.theriogenology.2005.05.055
Scully, S., Evans, A. C. O., Carter, F., Duffy, P., Lonergan, P., and Crowe, M. A. (2014). Ultrasound monitoring of blood flow and echotexture of the corpus luteum and uterus during early pregnancy of beef heifers. Theriogenology 83, 449–458. doi: 10.1016/j.theriogenology.2014.10.009
Seto-Ohshima, A., Yamazaki, Y., Karamura, N., Kitajima, S., Sano, M., and Mizutani, A. (1987). The early expression of immunoreactivity for calmodulin in the nervous system of mouse embryos. Histochemistry 86, 337–343. doi: 10.1007/bf00494990
Sharkey, D. J., Macpherson, A. M., Tremellen, K. P., and Robertson, S. A. (2007). Seminal plasma differentially regulates inflammatory cytokine gene expression in human cervical and vaginal epithelial cells. Mol. Hum. Reprod. 13, 491–501. doi: 10.1093/molehr/gam028
Sharkey, D. J., Tremellen, K. P., Jasper, M. J., Gemzell-Danielsson, K., and Robertson, S. A. (2012). Seminal fluid induces leukocyte recruitment and cytokine and chemokine mRNA expression in the human cervix after coitus. J. Immunol. 188, 2445–2454. doi: 10.4049/jimmunol.1102736
Silva, M., Niño, A., Guerra, M., Letelier, C., Valderrama, X. P., Adams, G. P., et al. (2011). Is an ovulation-inducing factor (OIF) present in the seminal plasma of rabbits? Anim. Reprod. Sci. 127, 213–221. doi: 10.1016/j.anireprosci.2011.08.004
Simintiras, C. A., Sanchez, J. M., McDonald, M., Martins, T., Binelli, M., and Lonergan, P. (2019). Biochemical characterization of progesterone-induced alterations in bovine uterine fluid: amino acid and carbohydrate composition. Biol. Reprod. 100, 672–685. doi: 10.1093/biolre/ioy234/5154910
Sjöblom, C., Roberts, C. T., Wikland, M., and Robertson, S. A. (2005). Granulocyte-macrophage colony-stimulating factor alleviates adverse consequences of embryo culture on fetal growth trajectory and placental morphogenesis. Endocrinology 146, 2142–2153. doi: 10.1210/en.2004-1260
Slusarski, D. C., and Pelegri, F. (2007). Calcium signaling in vertebrate embryonic patterning and morphogenesis. Dev. Biol. 307, 1–13. doi: 10.1038/jid.2014.371
Souza, C. E. A., Moura, A. A., Monaco, E., and Killian, G. J. (2008). Binding patterns of bovine seminal plasma proteins A1/A2, 30 kDa and osteopontin on ejaculated sperm before and after incubation with isthmic and ampullary oviductal fluid. Anim. Reprod. Sci. 105, 72–89. doi: 10.1016/j.anireprosci.2007.11.027
Sparrow, D. B., Boyle, S. C., Sams, R. S., Mazuruk, B., Zhang, L., Moeckel, G. W., et al. (2009). Placental insufficiency associated with loss of cited1 causes renal medullary dysplasia. J. Am. Soc. Nephrol. 20, 777–786. doi: 10.1681/asn.2008050547
Sponchiado, M., Gomes, N. S., Fontes, P. K., Martins, T., Del Collado, M., Pastore, A. D. A., et al. (2017). Pre-hatching embryo-dependent and -independent programming of endometrial function in cattle. PLoS One 12:e0175954. doi: 10.1371/journal.pone.0175954
Sponchiado, M., Gonella-Diaza, A. M., Rocha, C. C., Turco, E. G. L., Pugliesi, G., Leroy, J. L. M. R., et al. (2019). The pre-hatching bovine embryo transforms the uterine luminal metabolite composition in vivo. Sci. Rep. 9:8354. doi: 10.1038/s41598-019-44590-9
Suarez, S. S., and Pacey, A. A. (2006). Sperm transport in the female reproductive tract. Hum. Reprod. Update 12, 23–37. doi: 10.1093/humupd/dmi047
Tremellen, K. P., Seamark, R. F., and Robertson, S. A. (1998). Seminal transforming growth factor beta1 stimulates granulocyte-macrophage colony-stimulating factor production and inflammatory cell recruitment in the murine uterus. Biol. Reprod. 58, 1217–1225. doi: 10.1095/biolreprod58.5.1217
Vandesompele, J., De Preter, K., Pattyn, F., Poppe, B., Van Roy, N., De Paepe, A., et al. (2002). Accurate normalisation of real-time quantitative RT-PCR data by geometric averaging of multiple internal control genes. Genome Biol. 3, 503–513. doi: 10.3109/14397595.2014.1001475
Vicens, A., Lüke, L., and Roldan, E. R. S. (2014). Proteins involved in motility and sperm-egg interaction evolve more rapidly in mouse spermatozoa. PLoS One 9:e91302. doi: 10.1371/journal.pone.0091302
Watkins, A. J., Dias, I., Tsuro, H., Allen, D., Emes, R. D., Moreton, J., et al. (2018). Paternal diet programs offspring health through sperm- and seminal plasma-specific pathways in mice. Proc. Natl. Acad. Sci. U.S.A. 115, 10064–10069. doi: 10.1073/pnas.1806333115
Watkins, A. J., and Sinclair, K. D. (2014). Paternal low protein diet affects adult offspring cardiovascular and metabolic function in mice. Am. J. Physiol. Hear. Circ. Physiol. 306, 1444–1452. doi: 10.1152/ajpheart.00981.2013
Watkins, A. J., Sirovica, S., Stokes, B., Isaacs, M., Addison, O., and Martin, R. A. (2017). Paternal low protein diet programs preimplantation embryo gene expression, fetal growth and skeletal development in mice. Biochim. Biophys. Acta 1863, 1371–1381. doi: 10.1016/j.bbadis.2017.02.009
Yousef, M. S., Marey, M. A., Hambruch, N., Hayakawa, H., Shimizu, T., Hussien, H. A., et al. (2016). Sperm binding to oviduct epithelial cells enhances TGFB1 and IL10 expressions in epithelial cells aswell as neutrophils in vitro: prostaglandin E2 as a main regulator of anti-inflammatory response in the bovine oviduct. PLoS One 11:e0162309. doi: 10.1371/journal.pone.0162309
Zavadil, J., and Böttinger, E. P. (2005). TGF-β and epithelial-to-mesenchymal transitions. Oncogene 24, 5764–5774. doi: 10.1038/sj.onc.1208927
Keywords: seminal plasma, embryo development, corpus luteum, cattle, gene expression
Citation: Mateo-Otero Y, Sánchez JM, Recuero S, Bagés-Arnal S, McDonald M, Kenny DA, Yeste M, Lonergan P and Fernandez-Fuertes B (2020) Effect of Exposure to Seminal Plasma Through Natural Mating in Cattle on Conceptus Length and Gene Expression. Front. Cell Dev. Biol. 8:341. doi: 10.3389/fcell.2020.00341
Received: 09 January 2020; Accepted: 20 April 2020;
Published: 12 May 2020.
Edited by:
Tomer Avidor-Reiss, The University of Toledo, United StatesReviewed by:
Arlindo Moura, Federal University of Ceará, BrazilMats H. T. Troedsson, University of Kentucky, United States
Michael Robert McGowan, The University of Queensland, Australia
Copyright © 2020 Mateo-Otero, Sánchez, Recuero, Bagés-Arnal, McDonald, Kenny, Yeste, Lonergan and Fernandez-Fuertes. This is an open-access article distributed under the terms of the Creative Commons Attribution License (CC BY). The use, distribution or reproduction in other forums is permitted, provided the original author(s) and the copyright owner(s) are credited and that the original publication in this journal is cited, in accordance with accepted academic practice. No use, distribution or reproduction is permitted which does not comply with these terms.
*Correspondence: Beatriz Fernandez-Fuertes, YmVhdHJpei5mZXJuYW5kZXpAdWRnLmVkdQ==