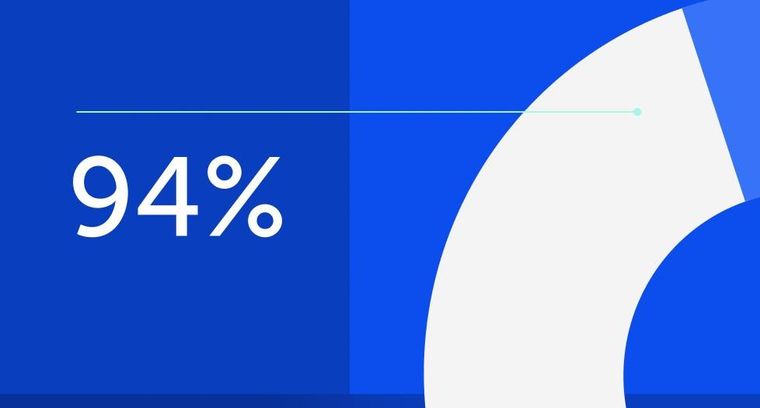
94% of researchers rate our articles as excellent or good
Learn more about the work of our research integrity team to safeguard the quality of each article we publish.
Find out more
ORIGINAL RESEARCH article
Front. Cell Dev. Biol., 02 April 2020
Sec. Epigenomics and Epigenetics
Volume 8 - 2020 | https://doi.org/10.3389/fcell.2020.00206
This article is part of the Research TopicThe Interplay Between Epigenetic Regulation and Other Cellular ProcessesView all 11 articles
Intramuscular fat (IMF), which regulated by genetics, nutrition and environment is an important factor that influencing meat quality. Up to now, the epigenetic regulation mechanism underlying poultry IMF deposition remains poorly understood. Here, we focused on the DNA methylation, which usually regulate genes in transcription level. To look into the essential role of DNA methylation on the IMF deposition, chicken intramuscular preadipocytes were isolated and cultured in vitro, and a model of intramuscular adipocyte differentiation was constructed. Combined the whole genome bisulfite sequencing (WGBS) and RNA-Seq technologies, we identified several methylated genes, which mainly affecting fatty acid metabolism and muscle development. Furthermore, we reported that DNA methylation regulate intramuscular adipogenesis by regulating the genes, such as collagen, type VI, alpha 1 (COL6A1) thus affecting IMF deposition. Overexpression of COL6A1 increases the lipid droplet and inhibits cell proliferation by regulating CHAD and CAMK2 in intramuscular adipocytes, while knockdown of COL6A1 shows the opposite effect. Taken together, our results reveal that DNA methylation plays an important role in poultry IMF deposition.
Intramuscular fat (IMF) is one of the most important factors that affect meat quality (Fanatico et al., 2007; Ros-Freixedes et al., 2014; Li et al., 2019). Previous researches have indicated that IMF improved the quality of meat by improving the flavor, juiciness and tenderness (Gao and Zhao, 2009). IMF deposition is primarily dependent on the differentiation, maturation and proliferation of intramuscular preadipocytes (Cristancho and Lazar, 2011; Zhang et al., 2019). Previous studies have identified about several genes related to chicken IMF, including PPARG, GPAT1, ACC, CD36, AGPAT1, and DGAT2, FABP, LPL, DGAT1, and SCL27A1 (Ye et al., 2010; Serão et al., 2011; Jeong et al., 2012; Li et al., 2013; Qiu et al., 2017). The mechanism that underlies chicken IMF deposition is very complicated obviously, involving many metabolic pathways and genes.
As one of the earliest discovered epigenetic modification, DNA methylation plays an extremely significant role in sustaining cell’s normal function in animals, gene expression regulation (Razin and Cedar, 1984), genetic imprinting (Jaenisch, 1997), embryonic development (Li et al., 2018), and tumor formation (Shivapurkar et al., 1986; Bender et al., 1998). Growing number of studies suggested that DNA methylation played significantly role in adipogenesis (Broholm et al., 2016; Chen et al., 2016; Lim et al., 2016). Previous studies recommended that DNMT3A inhibited porcine intramuscular preadipocytes differentiation by changing the methylation levels of p21 and PPARγ (Abdalla et al., 2018; Qimuge et al., 2019). Zhang et al. (2014) found that MBD4 inhibited porcine preadipocytes differentiation by changing the DNA methylation levels of adipogenic genes. Li et al. suggested that DNA methylation regulated chicken PPARG and CEBPA during the development of chicken adipose tissue (Sun et al., 2014; Gao et al., 2015). Our previous study identified large amount of differentially expressed genes (DEGs) during intramuscular adipogenic differentiation (Zhang et al., 2019). The epigenetic molecular mechanism, especially DNA methylation that underlies IMF deposition remains, however, poorly investigated.
In order to investigate the potential functions of DNA methylation that affected the poultry intramuscular adipogenesis. Whole genome single-base DNA methylation profiles of intramuscular preadipocytes and differentiated adipocytes were generated by whole genome bisulfite sequencing (WGBS). The present study integrated the RNA-Seq and WGBS data, aimed to describe the DNA methylation patterns in chicken intramuscular adipocytes and reveal the novel methylated candidate genes related to intramuscular adipogenesis. Our results offered basic research data about intramuscular adipogenesis and the IMF deposition in poultry.
To investigate the IMF deposition of poultry, chicken intramuscular adipogenic differentiation model in vitro was constructed in the present study. After 80–90% confluence, cells were exposed to MDIO differentiation medium. As shown in Figure 1A, cells were filled with lipid droplets after 10 days’ induction. Furthermore, qRT-PCR results suggested that the adipogenic markers PPARG, FABP4, CEBPA, and FASN significantly increased with adipogenic differentiation (p < 0.01) (Figure 1B).
Figure 1. The identification of chicken intramuscular preadipocytes differentiation model. (A) The shape of chicken intramuscular preadipocytes before (a’) and after (b’) adipogenic differentiation for 10 days. The oil red O staining of chicken intramuscular preadipocytes (c’) and mature adipocytes (d’); (B) qRT-PCR analysis of adipogenic makers PPARG, FABP4, CEBPA, and FASN during chicken intramuscular preadipocyte differentiation. The mRNA levels of adipogenic makers were detected by qRT-PCR at 0, 2, 4, 6, 8, 10 days after induced differentiation. (C) The OD value at 510 nm of Oil Red O staining during intramuscular preadipocyte differentiation. (n = 3, **p < 0.01).
To explore the role of DNA methylation in intramuscular adipogenic differentiation, 5 mC and 5 hmC levels were detected by immunofluorescence staining. Compared with intramuscular preadipocytes, the 5 mC levels of intramuscular adipocytes were significantly decreased (Figures 2A,B), whereas 5 hmC levels were higher in the intramuscular adipocytes compared to intramuscular preadipocytes (Figures 2A,B). At the same time, the mRNA expression levels of DNA methylation-related enzymes showed that mRNA expression levels of DNA methyltransferases DNMT3A/3B and DNMT1 were significantly decreased after induction of differentiation (p < 0.01, Figure 2C), while the demethylase TET1/2/3 were significantly increased after induction of differentiation (from days 2 to 4) (Figure 2C).
Figure 2. Difference in DNA methylation levels between intramuscular preadipocytes and adipocytes in chickens. (A) Immunofluorescence staining and quantification (B) of 5 mC (green) and 5 hmC (red) abundance in intramuscular preadipocytes and mature adipocytes. (C) Relative mRNA abundance of TET1/2/3, DNMT1, DNMT3A, and DNMT3B during the intramuscular adipogenic differentiation. qRT-PCR analysis of the relative mRNA levels DNA methylasferase DNMT1, DNMT3A/3B, and TET1/2/3 during chicken intramuscular preadipocyte differentiation. (n = 3, **p < 0.01, #p > 0.05).
In the present study, 34.43 and 35.29 G raw data were generated in intramuscular preadipocytes and matured intramuscular adipocytes, respectively. After taking the low quality, N (unknown) and connector contamination reads off, we finally got 212,981,499 and 232,403,717 clean reads in IM_Pre and IM_Ad groups, respectively (Table 1). There were 68.6 and 72% of chicken genome were covered with the uniquely mapped reads in the preadipocytes and adipocytes groups, respectively (Table 1). The unique alignments rate of was more than 80%. The Q30 value was more than 0.9, these results indicated a reliable sequencing outcome. In addition, Circos plot displayed the DNA methylation levels in the various sequence contexts (mCG, mCHG, and mCHH) (where H is A, C, or T) in chicken chromosomes (1–32 and the Z, W, MT chromosome; Figure 3).
Figure 3. Distribution of identified methylation sites on each chromosome. The outer ring represents the chicken genome labeled with chromosome number and position. (A–D) CG Methylation; (E–H) CHG Methylation; (I,J,M,S) CHH Methylation. (C,D,G,H,K,L) IMF_Pre; (A,B,E,F,I,J) IMF_Ad.
Pearson correlation analysis of the CpG base suggested that our samples have good data repeatability (r > 0.87) (Figure 4A). To investigate the differences of global DNA methylation profile between the two groups, DNA methylation levels in three contexts: CG, CHG, and CHH (where H is A, C, or T) were analyzed in the present study. As shown in Figure 4B, most proportion (60%) of cytosines were methylated in CpG context, only small proportion (1.2%) of cytosines were methylated in non-CG context (CHG and CHH context). To explore the patterns of methylated cytosines in chicken intramuscular adipocytes, we analyzed the genome-wide mC sequence preferences in various sequence contexts. Our results showed that the methylated cytosines preference for being located in CG, CHG, and CHH (H = A > T) (Figure 4C). The DMRs of the CGI were mainly located in the openSea (60.4%) and CpG island (CGI) (25.1%) (Figures 4D,E). The DMRs were mainly located in the intergenic region (42.9%), followed by the introns (31.25%) and the TSS region (16.9%) (Figures 4F,G).
Figure 4. The DNA methylation characteristics of intramuscular preadipocytes and adipocytes in chickens. (A) The correlation analysis of the methylation between samples. Heat maps showed the distribution of the methylated CpG sites, the bar plots showed the frequency of methylated CpG sites. (B) Comparison of DNA methylation patterns in different samples. (C) Sequence preferences for methylation in various sequence contexts. 9 bp base information around the position of mCG, mCHG, mCHH at the highest or lowest methylation levels, in which the methylated cytosine is in the fourth position. (D) The frequency distribution histogram of the distance from DMR to CGI. (E) The DMR Annotation in CGI functional elements (Island, Shore, Shelf and OpenSea). (F) The frequency distribution histogram of the distance from DMR to TSS. (G) The DMR Annotation in genome functional regions (5′UTR, 3′UTR, Exon, Intergenic, Intron, Promoter-TSS, and TSS).
In the present study, a total of 7580 DMRs were discovered. The DNA methylation level of adipocytes in the chicken genome showing a “V” trend around the promoter region (Figure 5A), which is consistent with previous studies in chicken breast muscle tissues (Zhang et al., 2017). Furthermore, we found that hypomethylation level in the promoter region and higher genome-wide gene expression level in intramuscular adipocytes groups compared with the preadipocytes group (Figures 5A,B). In addition, a large proportion of DMRs were intron and exon regions (Figure 5C). We noticed that most DMRs were length 100–200 bp and short than 1000 bp (Figure 5D). To look into the DMGs’ potential biological roles, gene ontogeny (GO) analysis and KEGG pathway analysis were performed. Our results showed that the DMGs mainly enriched in the regionalization and skeletal system development terms (Figure 5E), focal adhesion, fatty acid metabolism, ECM-receptor interaction and PPAR signaling pathways (Figure 5F).
Figure 5. The characteristics of DMRs and function analysis. (A) Global DNA methylation levels in different functional regions between different samples. (B) Gene expression levels (FPKM) in intramuscular preadipocytes and adipocytes. (C) The distribution of DMRs in different functional regions (5′UTR, 3′UTR, Exon, Intron, Start-codon, and Stop-codon). (D) The frequency distribution of DMRs count in length. (E) GO terms enriched analysis of DMGs. (F) Scatter plot of the top 30 KEGG enrichments analysis. Tick represents the pathway involved in lipid metabolism.
To explore whether the candidate DMGs are related to IMF deposition, we integrated the RNA-Seq and WGBS data to reveal methylated candidate genes associated with IMF deposition. Our results showed that there were 324 (hypermethylated and down-regulated) and 338 (hypomethylated and up-regulated) differentially expressed DMGs during adipocytes differentiation process (Figure 6A), several lipid metabolism-related and adipogenic differentiation genes, such as FASN, HADHA, INSIG1, BMP4, and LCLAT1 were found in the present study (Figure 6B). Besides, we observed that several genes were involved in the ECM-receptor interaction, insulin signaling pathway and fatty acid metabolism pathway, such as COL6A1, THBS1, LAMA2, HADHA, ACAA2, ELOVL7, ACADL, LCLAT1, INSIG1, and FOXO3 (Figure 6C). Moreover, the protein-protein interaction (PPI) network analysis illustrated that these DMGs were correlated with each other highly (Figure 6C). The DNA methylation and gene expression levels of three DMGs, INSIG1, BMP4, and COL6A1 were showed in Figure 6D. Furthermore, the correlations between IMF content and gene mRNA levels at different age stages were analyzed. Our results suggested that the expression levels of COL6A1 and ABCA1 were positively correlated with the IMF content (r = 0.980 and 0.994, p < 0.05) (Figure 6E). To study the expression trend of candidate genes in the differentiation of intramuscular adipocytes, the total RNA of intramuscular adipocytes differentiated at different periods was analyzed by qRT-PCR. Our results suggested that the mRNA level of COL6A1 was significantly increased during adipogenic differentiation of intramuscular preadipocytes. The mRNA level of ABCA1 significantly increased in the day 2, while declined slowly from days 4 to 10. And GSTT1L mRNA expression level was downregulated in day 2, while increased slowly after from days 4 to 10 (Figure 6F). Furthermore, our results suggested that the mRNA level of COL6A1 was significantly positive correlative with the TG content of intramuscular adipocytes during differentiation process (r = 0.84, p = 0.03), while ABCA1 and GSTT1L were was not significant correlative with the TG content (r = 0.14, p = 0.78 and r = 0.24, p = 0.65) (Supplementary Figure S1).
Figure 6. Candidate DMGs associated with IMF deposition. (A) The differentially expressed genes (DEGs) overlapped with differentially methylated gene (DMGs) in different groups. (B) Integrated analysis of DNA methylation levels and gene expression levels. (C) KEGG pathways and protein-protein interaction (PPI) network analysis of candidate DMGs associated with IMF deposition. (D) The DNA methylation levels (WGBS) and gene expression levels (RNA-seq) (IGV tracts) of three candidate DMGs (INSIG1, BMP4, and COL6A1). (E) The relative mRNA levels of three genes (COL6A1, ABCA1, and GSTT1L) and IMF content in breast muscle at 6, 14, 22, and 30 weeks old. (r, pearson correlation coefficient). (F) The relative mRNA levels of three genes during intramuscular adipogenic differentiation (n = 3, **p < 0.01, #p > 0.05).
According to the BSP results, there was a hypermethylated (72%) promoter region of COL6A1 in the intramuscular preadipocytes compared with differentiated adipocytes, while a low methylation level (28%) in the matured intramuscular adipocytes (Figures 7A,B). Furthermore, we found that the methylation of COL6A1 promoter were significantly negatively correlated with the mRNA level (r = −0.908, p < 0.05) (Figure 7C). And the DNA methylation levels of ABCA1 and GSTT1L promoter were significantly negatively correlated with their mRNA levels (r = −0.94, p < 0.01, and r = −0.87, p < 0.05) (Supplementary Figures S2, S3).
Figure 7. The DNA Methylation levels of COL6A1 promoter region. (A,B) The DNA Methylation levels of COL6A1 promoter region in intramuscular preadipocytes and adipocytes. BSP analyses of the DNA methylation of COL6A1 promoter during intramuscular adipogenic differentiation. (C) The correlation between the COL6A1 mRNA levels and DNA methylation levels during intramuscular adipogenic differentiation.
To further investigate whether the DNA methylation influence intramuscular adipogenesis, the methylation inhibitor, 5-AZA was used to treat intramuscular preadipocytes. As shown in Figure 8A, the methylation level declined 60% in preadipocytes in the presence of 5-AZA relative to the control cells. Meanwhile, the mRNA levels of COL6A1 and adipogenic makers, PPARG and CEBPA were significantly up-regulated after differentiation induction for 48 h in treating with 5-AZA cells (Figure 8B). In addition, Oil Red O staining showed that 5-AZA promoted the intramuscular adipogenesis (Figures 8C,D).
Figure 8. The effects of 5-AZA-dC treatment on intramuscular adipocytes differentiation. (A) The DNA methylation level (5 mC) in intramuscular preadipocytes treated with or without 5-AZA-dC (5 μM) for 96 h. (B) The relative mRNA levels in intramuscular adipocytes treated with or without 5-AZA-dC (5 μM) for 96 h. (C,D). Oil-red O staining of intramuscular adipocytes treated with or without 5-AZA-dC (5 μM) for 96 h. (n = 3). ∗∗p < 0.01.
To find out the potential role of COL6A1 in chicken intramuscular preadipocyte proliferation and differentiation, COL6A1 overexpression [pcDNA3.1(+)-COL6A1 vs. pcDNA3.1(+)-EGFP] and knockdown (siRNA-NC vs. siRNA-COL6A1) experiments were performed. The mRNA levels of COL6A1 increased over 13-fold in pcDNA3.1(+)-COL6A1-transfected group compared with control pcDNA3.1(+)-EGFP-transfected group (Figure 9A). Overexpressed COL6A1 significantly increased the mRNA expression levels of adipogenic makers PPARG, CEBPA, FABP4, and ECM-related genes CHAD, MMP7, MMP9, and CAMK2 (Figure 9B). In contrast, knockdown the COL6A1 down-regulated their mRNA expression levels (Figures 9C,D). EDU staining suggested that COL6A1 promoted intramuscular preadipocytes proliferation (Figure 9E). BODIPY staining showed that overexpressed COL6A1 significantly promoted the formation of lipid droplet in the intramuscular adipocytes, while decreased lipid droplet formation after RNA interference with COL6A1 (Figure 9F). Wound healing test suggested that COL6A1 promoted intramuscular adipocytes migration (Figure 9G).
Figure 9. The effects of COL6A1 overexpression and knockdown on cell proliferation, differentiation and migration. (A) Overexpressed of COL6A1 promoted the expression of adipogenic differentiation and ECM-related genes (B) of intramuscular adipocytes. The relative mRNA levels of genes were detected by qRT-PCR after transfected with plasmid for 48 h. (C) Knockdown of COL6A1 suppressed the expression of adipogenic differentiation and ECM-related genes (D) of intramuscular adipocytes. The relative mRNA levels of genes were detected by qRT-PCR after transfected with RNA oligos for 24 h. (E) COL6A1 promoted intramuscular preadipocytes proliferation. The percentage of EDU positive cells was quantified after transfected with plasmid or RNA oligos. (F) COL6A1 accelerated intramuscular preadipocytes differentiation. BODIPY (green) and DAPI (blue) staining of intramuscular adipocytes after transfected with plasmid or RNA oligos. (G) COL6A1 promoted intramuscular adipocytes migration. The width of the scratches was measured by microscope after transfected with plasmid or RNA oligos for 72 h (n = 3), *p < 0.05, **p < 0.01.
All animal experiments were conducted with the guidelines of Institutional Animal Care and Use Committee (IACUC) at the Henan Agricultural University (Zhengzhou, Henan, China) (#11-0085).
All of the Gushi chickens were purchased from the Animal Center of Henan Agricultural University (Zhengzhou, Henan, China). Chickens were fed with the same diet ad libitum in the same environment. Tissues used for tissues expression profiles were collected and stored at −80°C until use. The breast muscle tissues were used for the IMF preadipocytes isolation according to our previous methods (Zhang et al., 2019).
Genomic DNA used for WGBS was extracted by an animal genomic DNA kit (Tiangen, China) according to the manufacturer’s instructions. genomic DNA was interrupted into fragments and purified by PCR purification kit. Fragmented DNA was end-repaired, added “A” nucleotide to the 3′end and ligated with methylated adapters. Fragments with adapters were used for bisulfite convertion by a methylation-gold kit (ZYMO, Los Angeles, CA, United States). Furthermore, converted DNA fragments were sequenced by Illumina HiSeq 2500. After removing unknown nucleotides and low-quality reads of raw reads, clean reads were got and used for the downstream analysis.
Produced clean reads were mapped to chicken reference genome (GGA_5.0) using the Bismark software (version: 2.90) (Krueger and Andrews, 2011). Then, a methylkit R package (Akalin et al., 2012) was to estimate methylation status and ratio of the CpG sites, promoter region, CpG island region and gene annotation. To get different methylation status in the chicken different genomic regions, methylation levels at 5′-flanking 2 kb regions and gene sequences in different samples were plotted. The RNA-Seq data used in the present study come from our present study (Zhang et al., 2019). The IMF content data used in the present study came from our previous study (Fu et al., 2018).
The methylation regions with p ≤ 0.05 (chi-square test) and the degree of difference methylation >20% were considered as differentially methylated regions (DMRs). DMRs that overlapping with genes body or up or downstream 2 kb of body regions were considered as differentially methylated genes (DMGs). To investigate the functions of the DMGs, GO, and KEGG pathway analysis were conducted in the present study. Fisher’s Exact Test is p ≤ 0.05 as threshold.
DNA methylation levels in gene promoters were measured by the Bisulfite sequencing PCR (BSP). Briefly, 200 ng of the chicken preadipocytes and adipocytes genomic DNA was treated with bisulfite. The bisulfite-treated DNA was used for touchdown PCR. BSP primers were designed using the MethPrimer software1 (Supplementary Table S1). The PCR products were cloned into the pMD19-T vector (TaKaRa, China) and sequenced by Comate Bioscience Co., Ltd. (Jilin, China). The methylation levels visualizated by MSRcall software2.
To construct the overexpressed plasmid of COL6A1, the CDS sequence of chicken COL6A1 was synthesized and cloned into pcDNA3.1(+)-EGFP vector (Invitrogen, United States). Sanger sequencing was performed to confirm the sequence. The siRNAs for COL6A1 were purchase from GenePharma (Shanghai, China) and transfection with lipofectamine 3000 (Thermo, Shanghai, China). The siRNA-1 of COL6A1 were: 5′-GGAUGAUGCUGCUAAUGAATT-3′, and 5′-UUCAUUAGCA GCAUCAUCCTT-3′. The siRNA-2 of COL6A1 were: 5′-GGUC AUCGCCAAAGCUGUUTT-3′, and 5′-AACAGCUUUGGCGA UGACCTT-3′.
Total RNA was isolated by RNAiso Plus (TaKaRa, Dalian, China) following the instruction of manufacturers. The TAKARA PrimeScriptTM RT reagent kit (TaKaRa) was used for reverse transcription. The qRT-PCR primers were designed by Primer3plus3 (Supplementary Table S1). GAPDH was used as internal control to normalized to the expression level of genes. The analysis of genes relative expression levels was using 2–ΔΔCt method.
For immunofluorescence, intramuscular adipocytes were fixed with 4% PFA (Beyotime) for 40 min, permeabilized 0.5% Triton X-100 for 10 min, and then blocked with 2% bovine serum albumin (BSA) for 2 h. Following incubated overnight at 4°C with anti-5 mC (Active Motif, 1:200) and anti-5 hmC (Active Motif, 1:200), stained at room temperature for 1 h with Alexa Fluor 488 goat anti-mouse or 594 goat anti-rabbit. The DNA were stained with DAPI (10 μg/mL, Beyotime) for 5 min. The images were captured with fluorescence microscopy (Nikon, Tokyo, Japan). The fluorescence intensity was analyzed by ImageJ software.
After reaching 70–80% confluent, intramuscular preadipocytes were treated with demethylation agent 5-aza-dC (Sigma) (dissoloved in DMSO) at 5 μM for 96 h. DMSO treatment was used as a control. Then cells were induced differentiation for 96 h, then for downstream experiment.
The genomic DNAs were extracted with TIANamp Genomic DNA Kit (TIANGEN) following the instruction of manufacturers. The methylation analysis was performed by the 5 mC DNA ELISA Kit (Zymo Research, United States) following the manufacturer’s instructions. The microplate reader (Thermo Fisher) was used to detect the absorbance at 405 nm.
After transfection for 48 h, intramuscular adipocytes were incubated at 37°C with 50 μM EdU (RiboBio, China) for 2 h, then cells were fixed with 4% PFA for 30 min and neutralized by 2 mg/mL glycine solution, permeabilized with 0.5% Triton X-100. Then cells were incubated with Apollo Reaction Cocktail (RiboBio, China) for 30 min at room temperature. The DNA was stained with DAPI (Beyotime) for 15 min. The EDU-positive cells were observation with a fluorescence microscope (Nikon, Tokyo, Japan).
After reached 70–80% confluence, intramuscular preadipocytes were transfected with plasmid or RNA oligos. Subsequently, 10 μL pipette tips were used to generated linear wound. The width of the scratches was measured by microscope (Nikon, Japan) at 0 and 72 h.
Oil red O staining was performed following our previously method (Zhang et al., 2018). Cells were fixed with10% PFA for 40 min, and then stained with oil red O for 20 min. The dye was extracted by isopropanol incubation for 15 min at room temperature. Quantitative assessment was obtained by microplate reader (Thermo Scientific) at 510 nm. Where indicated, lipids were co-stained by adding BODIPY 493/503 (1 mg/mL, Molecular Probes #D3922) to secondary antibody solution. Cells were washed three times with PBS prior to imaging.
Statistical analyses were performed using SPSS19 software (SPSS Inc., Chicago, IL, United States). In the present study, the results were presented as mean ± SEM, were subjected to statistical analysis by two-tailed t-test. The level of significance was presented as ∗p < 0.05) and ∗∗p < 0.01.
IMF content contributes to the meat juiciness and tenderness. Our previous study suggested that the breast muscle of later laying-period hens had higher IMF content than that of juvenile hens, while they exhibited higher global DNA methylation levels (Zhang et al., 2017). Growing numbers of studies demonstrated that DNA methylation played important roles in adipogenesis. Therefore, we speculated that DNA methylation might have great influences on adipogenic differentiation of chicken intramuscular adipocytes in vitro.
According to our WGBS data, 60% of mC were found to be existed in the CG context, 0.6% in the CHG context, and 0.7% in the CHH context in the present study. The methylation level at the genome-wide scale was significantly reduced in the mature intramuscular adipocytes. We noticed that the DNA methylation level declined aggressively prior to TSS and gradually rose in the coding region of the chicken genome, which is consistent with previous studies in chicken (Zhang et al., 2017). The exon and intron regions of the chicken genome consisted of a large proportion of the DMRs, a small part of DMRs were belong to the 5′UTR and 3′UTR (Figure 5). The methylation regulation of the intron regions underlying adipocytes differentiation was worth to study in the future.
DNMT1 mainly involved in maintain methylation (Song et al., 2012), while DNMT3A/3B mainly involved in the de novo DNA methylation (Li et al., 2007; Hervouet et al., 2009). Tet methylcytosine dioxygenases (TET1/2/3) play important roles in elimination of methylation (Williams et al., 2011). qPCR results showed that the mRNA levels of DNA methyltransferases DNMT1, DNMT3A/3B, and TET1/2/3 were significantly down-regulated during intramuscular adipocyte differentiation, suggesting that whole-genome DNA demethylation may occur during adipocyte differentiation. The process of adipocyte differentiation requires the initiation of a large number of genes and transcription factors for synergistic expression, which may be related to the differentiation of adipocytes (Mersmann and Ding, 2001). Our previous study found that the hypermethylation in the promoters of ABCA1, COL6A1, and GSTT1L, thus inhibiting their expression in the later laying-period hens (Zhang et al., 2017). Interestingly, we noticed that they were up-regulated after adipocyte differentiation, suggesting that they may play crucial roles in the differentiation of intramuscular preadipocytes.
ABCA1 maintains cholesterol homeostasis, regulates lipid metabolism in adipocytes (Schmitz et al., 1999; Schmitz and Langmann, 2005). The DNA methylation level of ABCA1 affects high density lipoprotein cholesterol (HDLC) levels in patients with familial hypercholesterolemia (Yasuaki et al., 2017). ABCA1 expression influenced triglyceride metabolism in bovine mammary epithelial cells by regulating the expression of related genes in the lipid metabolism pathway (Chen et al., 2019). ABCA1 silencing by siRNA also reduce peroxisome proliferator-activated receptor γ (PPARγ) expression and triglyceride content during 3T3-L1 pre-adipocyte differentiation (Cuffe et al., 2018). ABCA1 is significantly up-regulated after differentiation of 3T3-L1 adipocytes, which is consistent with our study on chicken intramuscular adipocytes (Le et al., 2003). Glutathione S-transferases (GSTT1) Glutathione S-transferases influencing the lipid peroxides metabolism during adipocytes differentiation process (Jowsey et al., 2003; Corton et al., 2008). Wang et al. (2009) found that GSTT1 were upregulated in the adipose tissues of fat line birds compared with lean line birds.
Muscle tenderness is closely related with the content of collagen. The ECM not only affects the development of muscle fibers, but also has an effect on IMF content and tenderness (Cánovas et al., 2010). COL6A1 gene is involved in cell adhesion and extracellular matrix (ECM). Previous studies suggested that the expression of collagen synthesis related-genes is related to the meat quality of beef (Zhang et al., 2011).
To further investigate the effects of DNA methylation on intramuscular preadipocytes differentiation, we focused on collagen type VI alpha 1 chain gene (COL6A1), which is located in the extracellular matrix (ECM) receptor interaction and focal adhesion pathway. With the differentiation of preadipocytes, the lipid droplets gradually fill the cytoplasm, and the cells are easily crushed and ruptured. At this time, the collagen components that act as protective cells in the extracellular matrix are synthesized in large amounts. It is generally believed that DNA methylation of the gene promoter region inhibits gene expression (Lorincz et al., 2004). In our study, we found that the DNA methylation level of COL6A1 promoter was decreased while the mRNA level was increasing after adipogenic differentiation. The methylation inhibitor, 5-AZA-dC promotes intramuscular adipocytes differentiation by increasing the core adipogenic factors, PPARG and CEBPA. Furthermore, function loss and gain of experiment of COL6A1 suggested that DNA methylation can regulate the chicken intramuscular adipocytes differentiation by affecting the expression of ECM-related genes (such as COL6A1 gene).
In conclusion, our study firstly supplies comprehensive DNA methylation atlas in chicken adipocytes. Integrated DNA methylation with transcriptome, the present study revealed several potential genes (such as COL6A1, FASN, and INSIG, etc.) and pathways related to lipid metabolism and adipocytes differentiation regulated by DNA methylation (Figure 10). Our study will accelerate the study of genome epigenetic mechanism in adipocytes differentiation and IMF deposition in poultry.
Figure 10. Schema of the epigenetic regulatory mechanism of DNA methylation intramuscular adipogenesis in chicken. The global DNA methylation level decreased with the expression levels of DNA methylasferase (DNMTs, TETs) during intramuscular adipogenic differentiation. Thus, increasing large amount of lipid metabolism and adipocyte differentiation-related genes (such as COL6A1, FASN, and INSIG1, etc.) expression.
The datasets generated for this study can be found in the PRJNA429489 and PRJNA428933.
The animal study was reviewed and approved by Institutional Animal Care and Use Committee (IACUC).
MZ, GS, and XK conceived of and designed the experiments. MZ, DL, YZ, and ZW performed the experiments. MZ, DL, and YZ analyzed the data. ZL, GL, XM, DZ, RH, and RJ contributed reagents, materials, and analysis tools. MZ wrote the manuscript. ZL reviewed the manuscript. All authors approved the final manuscript.
This research was supported by the China Agriculture Research System (CARS-40-K04), National Natural Science Foundation of China (31572356 and 31501948), Research on the Foundation and Frontier Technology of Henan Science and Technology Department (162300410162), Program for Innovation Research Team of Ministry of Education (IRT16R23) and Key Science and Technology Research Project of Henan Province (151100110800).
The authors declare that the research was conducted in the absence of any commercial or financial relationships that could be construed as a potential conflict of interest.
The Supplementary Material for this article can be found online at: https://www.frontiersin.org/articles/10.3389/fcell.2020.00206/full#supplementary-material
FIGURE S1 | The correlation between the mRNA levels of COL6A1, ABCA1, and GSTT1L and the TG content of intramuscular adipocytes during differentiation process.
FIGURE S2 | The DNA Methylation levels of ABCA1 promoter region. (A,B) The DNA Methylation levels of ABCA1 promoter region in intramuscular preadipocytes and adipocytes. BSP analyses of the DNA methylation of ABCA1 promoter during intramuscular adipogenic differentiation. (C) The correlation between the ABCA1 mRNA levels and DNA methylation levels during intramuscular adipogenic differentiation.
FIGURE S3 | The DNA Methylation levels of GSTT1L promoter region. (A,B) The DNA Methylation levels of GSTT1L promoter region in intramuscular preadipocytes and adipocytes. BSP analyses of the DNA methylation of GSTT1L promoter during intramuscular adipogenic differentiation. (C) The correlation between the GSTT1L mRNA levels and DNA methylation levels during intramuscular adipogenic differentiation.
TABLE S1 | Primer sequences for BSP and qRT-PCR.
Abdalla, B. A., Li, Z., Ouyang, H., Jebessa, E., Sun, T., Yu, J. A., et al. (2018). A novel Dnmt3a1 transcript inhibits adipogenesis. Front. Physiol. 9:1270. doi: 10.3389/fphys.2018.01270
Akalin, A., Kormaksson, M., Li, S., Garrett-Bakelman, F. E., Figueroa, M. E., Melnick, A., et al. (2012). methylKit: a comprehensive R package for the analysis of genome-wide DNA methylation profiles. Genome Biol. 13:R87. doi: 10.1186/gb-2012-13-10-r87
Bender, C. M., Pao, M. M., and Jones, P. A. (1998). Inhibition of DNA methylation by 5-Aza-2’-deoxycytidine suppresses the growth of human tumor cell lines. Cancer Res. 58, 95–101.
Broholm, C., Olsson, A. H., Perfilyev, A., Gillberg, L., Hansen, N. S., Ali, A., et al. (2016). Human adipogenesis is associated with genome-wide DNA methylation and gene expression changes. Epigenomics 8, 1601–1617. doi: 10.2217/epi-2016-0077
Cánovas, A., Quintanilla, R., Amills, M., and Pena, R. N. (2010). Muscle transcriptomic profiles in pigs with divergent phenotypes for fatness traits. BMC Genomics 11:372. doi: 10.1186/1471-2164-11-372
Chen, Y. S., Wu, R., Yang, X., Kou, S., MacDougald, O. A., Yu, L., et al. (2016). Inhibiting DNA methylation switches adipogenesis to osteoblastogenesis by activating Wnt10a. Sci. Rep. 6:25283. doi: 10.1038/srep25283
Chen, Z., Chu, S. F., Wang, X. L., Fan, Y., Zhan, T., Arbab, A. A. I., et al. (2019). microRNA-106b regulates milk fat metabolism via ATP binding cassette subfamily A member 1 (ABCA1) in bovine mammary epithelial cells. J. Agric. Food Chem. 67, 3981–3990. doi: 10.1021/acs.jafc.9b00622
Corton, M., Botella-Carretero, M., Lopez, J. A., Camafeita, E., San Millan, J. L., Escobar-Morreale, H. F., et al. (2008). Proteomic analysis of human omental adipose tissue in the polycystic ovary syndrome using two-dimensional difference gel electrophoresis and mass spectrometry. Hum. Reprod. 23, 651–661. doi: 10.1093/humrep/dem380
Cristancho, A. G., and Lazar, M. A. (2011). Forming functional fat: a growing understanding of adipocyte differentiation. Nat. Rev. Mol. Cell Biol. 12, 722–734. doi: 10.1038/nrm3198
Cuffe, H., Liu, M. X., Key, C.-C. C., Boudyguina, E., Sawyer, J. K., Weckerle, A., et al. (2018). Targeted deletion of adipocyte Abca1 (ATP-binding cassette transporter A1) impairs diet-induced obesity. Arterioscher. Thromb. Vasc. Biol. 38, 733–743. doi: 10.1161/ATVBAHA.117.309880
Fanatico, A. C., Pillai, P. B., Emmert, J. L., and Owens, C. M. (2007). Meat quality of slow- and fast-growing chicken genotypes fed low-nutrient or standard diets and raised indoors or with outdoor access. Poult. Sci. 86, 2245–2255. doi: 10.1093/ps/86.10.2245
Fu, S., Zhao, Y. L., Li, Y. F., Li, G., Xi, L., Chen, Y., et al. (2018). Characterization of miRNA transcriptome profiles related to breast muscle development and intramuscular fat deposition in chickens. J. Cell Biochem. 119, 7063–7079. doi: 10.1002/jcb.27024
Gao, S. Z., and Zhao, S. M. (2009). Physiology, affecting factors and strategies for control of pig meat intramuscular fat. Recent Pat. Food Nutr. Agric. 1, 59–74. doi: 10.2174/2212798410901010059
Gao, Y., Sun, Y., Duan, K., Shi, H., Wang, S., Li, H., et al. (2015). CpG site DNA methylation of the CCAAT/enhancer-binding protein, alpha promoter in chicken lines divergently selected for fatness. Anim. Genet. 46, 410–417. doi: 10.1111/age.12326
Hervouet, E., Vallette, F. M., and Pierre-Fancois, C. (2009). Dnmt3/transcription factor interactions as crucial players in targeted DNA methylation. Epigenetics 4, 487–499. doi: 10.4161/epi.4.7.9883
Jaenisch, R. (1997). DNA methylation and imprinting: why bother? Trends Genet. 13, 323–329. doi: 10.1016/s0168-9525(97)01180-3
Jeong, J., Kwon, E. G., Im, S. K., Seo, K. S., and Baik, M. (2012). Expression of fat deposition and fat removal genes is associated with intramuscular fat content in longissimus dorsi muscle of Korean cattle steers1. J. Anim. Sci. 90, 2044–2053. doi: 10.2527/jas.2011-4753
Jowsey, I. R., Smith, S. A., and Hayes, J. D. (2003). Expression of the murine glutathione S-transferase α3 (GSTA3) subunit is markedly induced during adipocyte differentiation: activation of the GSTA3 gene promoter by the pro-adipogenic eicosanoid 15-deoxy-Δ12,14-prostaglandin J2. Biochem. Biophys. Res. Commun. 312, 1226–1235. doi: 10.1016/j.bbrc.2003.11.068
Krueger, F., and Andrews, S. R. (2011). Bismark: a flexible aligner and methylation caller for bisulfite-Seq applications. Bioinformatics 27, 1571–1572. doi: 10.1093/bioinformatics/btr167
Le, Lay S, Robichon, C., Le, Liepvre X, Dagher, G., Ferre, P., and Dugail, I. (2003). Regulation of ABCA1 expression and cholesterol efflux during adipose differentiation of 3T3-L1 cells. J. Lipid Res. 44, 1499–1507. doi: 10.1194/jlr.M200466-JLR200
Li, C., Fan, Y., Li, G., Xu, X., Duan, J., Li, R., et al. (2018). DNA methylation reprogramming of functional elements during mammalian embryonic development. Cell Discov. 4:41. doi: 10.1038/s41421-018-0039-9
Li, F., Li, D. H., Zhang, M., Sun, J. W., Li, W. T., Jiang, R. R., et al. (2019). miRNA-223 targets the GPAM gene and regulates the differentiation of intramuscular adipocytes. Gene 15, 106–113. doi: 10.1016/j.gene.2018.10.054
Li, T., Xu, D., Zuo, B., Lei, M., Xiong, Y., Chen, H., et al. (2013). Ectopic overexpression of porcine DGAT1 increases intramuscular fat content in mouse skeletal muscle. Transgenic Res. 22, 187–194. doi: 10.1007/s11248-012-9633-z
Li, Y. Q., Zhou, P. Z., Zheng, X. D., Walsh, C. P., and Xu, G. L. (2007). Association of Dnmt3a and thymine DNA glycosylase links DNA methylation with base-excision repair. Nucleic Acids Res. 35, 390–400. doi: 10.1093/nar/gkl1052
Lim, Y. C., Chia, S. Y., Jin, S., Han, W., Ding, C., and Sun, L. (2016). Dynamic DNA methylation landscape defines brown and white cell specificity during adipogenesis. Mol. Metab. 5, 1033–1041. doi: 10.1016/j.molmet.2016.08.006
Lorincz, M. C., Dickerson, D. R., Schmitt, M., and Groudine, M. (2004). Intragenic DNA methylation alters chromatin structure and elongation efficiency in mammalian cells. Nat. Struct. Mol. Biol. 11, 1068–1075. doi: 10.1038/nsmb840
Mersmann, H. J., and Ding, S. T. (2001). Fatty acids modulate porcine adipocyte differentiation and transcripts for transcription factors and adipocyte-characteristic proteins? J. Nutr. Biochem. 12, 101–108. doi: 10.1016/S0955-2863(00)00136-4
Qimuge, N., He, Z., Qin, J., Sun, Y., Wang, X., Yu, T., et al. (2019). Overexpression of DNMT3A promotes proliferation and inhibits differentiation of porcine intramuscular preadipocytes by methylating p21 and PPARg promoters. Gene 696, 54–62. doi: 10.1016/j.gene.2019.02.029
Qiu, F., Liang, X., Jing-e, M., Wen, L., Li, Z., Zhe, C., et al. (2017). Lower expression of SLC27A1 enhances intramuscular fat deposition in chicken via down-regulated fatty acid oxidation mediated by CPT1A. Front. Physiol. 8:449. doi: 10.3389/fphys.2017.00449
Razin, A., and Cedar, H. (1984). DNA methylation and gene expression. Microbiol. Rev. 55, 451–458. doi: 10.1007/978-1-4613-8519-6-8
Ros-Freixedes, R., Reixach, J., Bosch, L., Tor, M., and Estany, J. (2014). Genetic correlations of intramuscular fat content and fatty acid composition among muscles and with subcutaneous fat in Duroc pigs. J. Anim. Sci. 92, 5417–5425. doi: 10.2527/jas.2014-8202
Schmitz, G., Kaminski, W. E., Porsch-Ozcürümez, M., Klucken, J., Orsó, E., Bodzioch, M., et al. (1999). ATP-binding cassette transporter A1 (ABCA1) in macrophages: a dual function in inflammation and lipid metabolism? Pathobiology 67, 236–240. doi: 10.1159/000028100
Schmitz, G., and Langmann, T. (2005). Transcriptional regulatory networks in lipid metabolism control ABCA1 expression. Biochim. Biophys. Acta. 1735, 1–19. doi: 10.1016/j.bbalip.2005.04.004
Serão, N. V., Veroneze, R., Ribeiro, A. M., Verardo, L. L., Braccini Neto, J., Gasparino, E., et al. (2011). Candidate gene expression and intramuscular fat content in pigs. J. Anim. Breed. Genet. 128, 28–34. doi: 10.1111/j.1439-0388.2010.00887.x
Shivapurkar, N., Wilson, M. J., Hoover, K. L., Mikol, Y. B., Creasia, D., and Poirier, L. A. (1986). Hepatic DNA methylation and liver tumor formation in male C3H mice fed methionine- and choline-deficient diets. J. Natl. Cancer Inst. 77, 213–217.
Song, J. K., Teplova, M., Ishibe-Murakami, S., and Patel, D. J. (2012). Structure-based mechanistic insights into DNMT1-mediated maintenance DNA Methylation. Science 335, 709–712. doi: 10.1126/science.1214453
Sun, Y. N., Gao, Y., Qiao, S. P., Wang, S. Z., Duan, K., Wang, Y. X., et al. (2014). Epigenetic DNA methylation in the promoters of peroxisome proliferator-activated receptor γ in chicken lines divergently selected for fatness. J. Anim. Sci. 92, 48–53. doi: 10.2527/jas.2013-6962
Wang, D., Wang, N., Li, N., and Li, H. (2009). Identification of differentially expressed proteins in adipose tissue of divergently selected broilers. Poult. Sci. 88, 2285–2292. doi: 10.3382/ps.2009-00190
Williams, K., Christensen, J., and Helin, K. (2011). DNA methylation: TET proteins-guardians of CpG islands? EMBO Rep. 13, 28–35. doi: 10.1038/embor.2011.233
Yasuaki, T., Yuko, U., Akira, A., Daisuke, M., Keiji, Y., Takata, K., et al. (2017). Increased hepatic ABCA1 transporter is associated with hypercholesterolemia in a cholestatic rat model and primary biliary cholangitis patients. Med. Mod. Morphol. 50, 227–237. doi: 10.1007/s00795-017-0166-7
Ye, M. H., Chen, J. L., Zhao, G. P., Zheng, M. Q., and Wen, J. (2010). Associations of A-FABP and H-FABP markers with the content of intramuscular fat in Beijing-You chicken. Anim. Biotechnol. 21, 14–24. doi: 10.1080/10495390903328116
Zhang, L. J., Zhu, Y. N., Gao, Y., Liu, S. Y., Zhai, B., Li, C. H., et al. (2014). The MBD4 gene plays an important role in porcine adipocyte differentiation. Cell Physiol. Biochem. 34, 1216–1226. doi: 10.1159/000366333
Zhang, M., Li, D. H., Li, F., Sun, J. W., Jiang, R. R., Li, Z. J., et al. (2018). Integrated analysis of MiRNA and genes associated with meat quality reveals that Gga-MiR-140-5p affects intramuscular fat deposition in chickens. Cell Physiol. Biochem. 46, 2421–2433. doi: 10.1159/000489649
Zhang, M., Li, F., Ma, X. F., Li, W. T., Jiang, R. R., Han, R. L., et al. (2019). Identification of differentially expressed genes and pathways between intramuscular and abdominal fat-derived preadipocyte differentiation of chickens in vitro. BMC Genomics 20:743. doi: 10.1186/s12864-019-6116-0
Zhang, M., Yan, F. B., Li, F., Jiang, K. R., Li, D. H., Han, R. L., et al. (2017). Genome-wide DNA methylation profiles reveal novel candidate genes associated with meat quality at different age stages in hens. Sci. Rep. 7:45564. doi: 10.1038/srep45564
Keywords: DNA methylation, transcriptome, intramuscular adipocytes differentiation, COL6A1, IMF deposition
Citation: Zhang M, Li D, Zhai Y, Wang Z, Ma X, Zhang D, Li G, Han R, Jiang R, Li Z, Kang X and Sun G (2020) The Landscape of DNA Methylation Associated With the Transcriptomic Network of Intramuscular Adipocytes Generates Insight Into Intramuscular Fat Deposition in Chicken. Front. Cell Dev. Biol. 8:206. doi: 10.3389/fcell.2020.00206
Received: 12 November 2019; Accepted: 10 March 2020;
Published: 02 April 2020.
Edited by:
Kai Tang, Purdue University, United StatesReviewed by:
Guiping Zhao, Chinese Academy of Agricultural Sciences, ChinaCopyright © 2020 Zhang, Li, Zhai, Wang, Ma, Zhang, Li, Han, Jiang, Li, Kang and Sun. This is an open-access article distributed under the terms of the Creative Commons Attribution License (CC BY). The use, distribution or reproduction in other forums is permitted, provided the original author(s) and the copyright owner(s) are credited and that the original publication in this journal is cited, in accordance with accepted academic practice. No use, distribution or reproduction is permitted which does not comply with these terms.
*Correspondence: Guirong Sun, Z3JzdW4yMDAwQDEyNi5jb20=
Disclaimer: All claims expressed in this article are solely those of the authors and do not necessarily represent those of their affiliated organizations, or those of the publisher, the editors and the reviewers. Any product that may be evaluated in this article or claim that may be made by its manufacturer is not guaranteed or endorsed by the publisher.
Research integrity at Frontiers
Learn more about the work of our research integrity team to safeguard the quality of each article we publish.