- 1Lazzaro Spallanzani, National Institute for Infectious Diseases – IRCCS, Rome, Italy
- 2Department of Biology, University of Rome Tor Vergata, Rome, Italy
- 3Department of Molecular Medicine, Sapienza University of Rome, Rome, Italy
In the last years, proteomics has represented a valuable approach to elucidate key aspects in the regulation of type I/III interferons (IFNs) and autophagy, two main processes involved in the response to viral infection, to unveil the molecular strategies that viruses have evolved to counteract these processes. Besides their main metabolic roles, mitochondria are well recognized as pivotal organelles in controlling signaling pathways essential to restrain viral infections. In particular, a major role in antiviral defense is played by mitochondrial antiviral signaling (MAVS) protein, an adaptor protein that coordinates the activation of IFN inducing pathways and autophagy at the mitochondrial level. Here, we provide an overview of how mass spectrometry-based studies of protein–protein interactions and post-translational modifications (PTMs) have fostered our understanding of the molecular mechanisms that control the mitochondria-mediated antiviral immunity.
Introduction
Mitochondria are the powerhouse of the cell due to their primary contribution in cell respiration. In addition to their canonical role in cellular metabolism, mitochondria have emerged as a central platform for the regulation of antiviral signaling pathways (Mohanty et al., 2019). Although several pathogen-activated pathways are influenced by mitochondria, this role is to be attributed mainly to the mitochondrial localization of the signaling adaptor mitochondrial antiviral signaling (MAVS) protein, a key mediator of the innate immune response during RNA viral infection (Vazquez and Horner, 2015).
In the last years, remarkable advances have been made in our knowledge about the molecular mechanisms underlying MAVS signaling. The development of innovative technologies in the field of transcriptomics, proteomics, reverse genetics, and structural and cell biology has dramatically improved our understanding of how RNA viruses can be sensed and lead to the expression of hundreds of genes dedicated to infection inhibition. In particular, yeast two-hybrid and mass spectrometry (MS) approaches have provided detailed information on how MAVS-dependent antiviral responses are rapidly activated, and also tightly controlled by protein catabolic processes, i.e. the ubiquitin–proteasome system and autophagy, to avoid excessive inflammation and cell death. Moreover, these approaches have widened our understanding of how viral proteins are able to inhibit MAVS activation to accomplish viral replication and how energy metabolism may influence mitochondrial antiviral pathways.
Here, we review our current view of MAVS signaling, highlighting how proteomics has deepened our understanding of this pathway regulation in terms of protein–protein interactions and post-translational modifications (PTMs).
Innate Immunity and Mitochondria
The innate immune response is the first line of defense against invading pathogens that is activated following the recognition of specific entities, called pathogen-associated molecular patterns (PAMPs), through a series of receptors, termed pattern recognition receptors (PRRs). Upon detection of PAMPs, the transcription of a myriad of antiviral genes is activated, establishing a cellular antiviral state that helps cells to restrict and/or clear infection (Brubaker et al., 2015).
Based on their structures, locations, and functional specificities, PRRs are separated into discrete families, which include the membrane bound Toll-like receptors (TLRs), the cytosolic nucleotide-binding oligomerization domain (NOD)-like receptors (NLRs), cyclic GMP-AMP (cGAMP) synthase (cGAS), and retinoic acid-inducible gene I (RIG-I)-like receptors (RLRs) (Kawai and Akira, 2011; Franchi et al., 2012; Streicher and Jouvenet, 2019). The activity of these PRRs has been described to be influenced at various levels by mitochondria.
The TLRs are a family of transmembrane PRRs that are activated by the binding of ligands to their C-terminal leucine-rich repeats. Ten TLRs have been identified in humans, with TLRs 1, 2, 4, 5, 6, and 10 located at the cell surface and TRLs 3, 7, 8, and 9 spanning the endosomal membrane. Plasma membrane TLRs mainly recognize microbial wall components, such as lipids, polysaccharides, and proteins, while intracellular TLRs sense pathogen nucleic acids (Kawai and Akira, 2011). Stimulation of TLRs results in the activation of the transcription factors NF-κB and IRF3/IRF7 to induce the expression of type I interferon (IFN) genes and other inflammatory cytokines.
Mitochondria are directly involved in the regulation of TLR activity. A relevant example is represented by TLR1, TLR2, and TLR4, which were reported to enhance mitochondrial ROS generation in bacteria-infected macrophages to potentiate the antimicrobial killing (West et al., 2011). This effect is mediated by evolutionarily conserved signaling intermediate in Toll (ECSIT) pathways, a mitochondrial matrix protein required for mitochondrial respiratory complex I activity. Following TLR engagement, the E3 ubiquitin ligase tumor necrosis factor receptor-associated factor 6 (TRAF6) binds to a fraction of the ECSIT protein that becomes accessible on the mitochondrial surface and elicits the pro-oxidant activity of ECSIT via non-degradative ubiquitination (West et al., 2011). Moreover, TLR4 activation drives the formation of a complex including TRAF6, ECSIT, and transforming growth factor-β-activated kinase 1 (TAK1), which promotes TAK1 kinase activity and the activation of the NF-κB signaling (Wi et al., 2014).
The NLR family of PRRs are cytoplasmic proteins that stimulate innate immunity in response to both PAMPs, such as microbial components (e.g. peptidoglycan, viral RNA), and damage-associated molecular patterns (DAMPs), including host components (cholesterol crystals, uric acid), and environmental sources (alum, asbestos, skin irritants) (Franchi et al., 2012). Upon activation, a unique feature of NLRs is the formation of a multiprotein complex termed “inflammasome.” Here, the NLRs bind pro-caspase-1 through the adaptor apoptosis-associated speck-like protein containing a caspase activation and recruitment domain (CARD) (ASC). Inflammasome assembly triggers auto-catalysis of pro-caspase-1, which is able to process the pro-IL (interleukin)-1β and pro-IL-18 into their active forms in order to induce, once secreted, a broad inflammatory response (Sharma and Kanneganti, 2016).
A direct link between mitochondria and NLR activation has been reported for NLRP3. Active NLRP3 translocates from cytosol to mitochondria, where it functions as a scaffold for inflammasome assembly (Swanson et al., 2019). Here, NLRP3 was found to interact with MAVS, with this binding being necessary for optimal inflammasome activity (Subramanian et al., 2013).
Cyclic GMP-AMP synthase is one of the cellular sensors of non-self DNA, together with the endosomal TLR9 and the NLR family protein Absent in Melanoma 2 (AIM2). Cytosolic DNA binds to cGAS and stimulates its enzymatic activity to form the second messenger cGAMP (Wu et al., 2013). cGAMP binds to the endoplasmic reticulum (ER) resident protein STING, promoting a conformational change that allows STING to interact with the protein kinases TBK1 (TANK binding kinase-1) and IKKs (IκB kinases) and to translocate from the ER to the Golgi apparatus. Activated TBK1 and IKKs phosphorylate the transcription factors IRF3 and NF-κB so as to induce the expression of type I IFN and inflammatory cytokines (Motwani et al., 2019).
Although DNA and RNA sensing responses occur through independent receptors, the induction of the antiviral response by one of these agents also relies on the activity of the other pathway. Multiple molecular mechanisms are responsible for this strict interdependence, including the direct interaction of the main regulators of these pathways at the ER–mitochondria contact sites, as well as the fact that many downstream factors are shared between these pathways. For a more exhaustive review of the crosstalk between RNA and DNA sensing pathways, see Zevini et al. (2017).
The RIG-I-Like Receptor Signaling Pathways
Apart from the endosome localized TLRs, the recognition of non-self RNA is mediated by the cytosolic sensors of the RLR pathway (Figure 1).
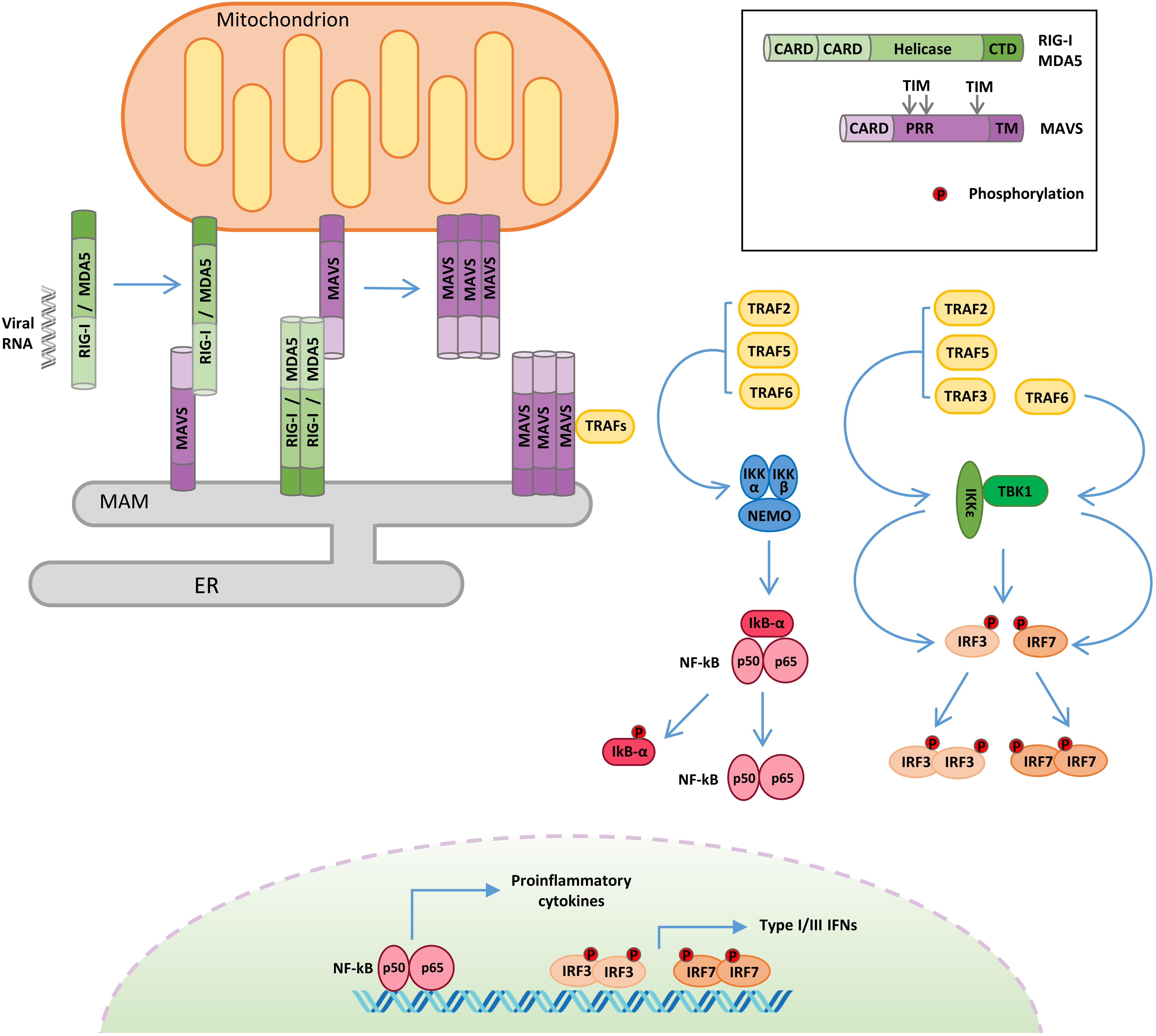
Figure 1. Retinoic acid-inducible gene I-like receptor (RLR)–mitochondrial antiviral signaling (MAVS) protein signaling. Following viral RNA sensing, retinoic acid-inducible gene I (RIG-I)/melanoma differentiation associated gene 5 (MDA5) oligomerize and relocalize from the cytoplasm to outer mitochondrial membrane (OMM) and mitochondria-associated membrane (MAM). RIG-I/MDA5 interaction with MAVS induces MAVS oligomerization and the formation of a signalosome where the tumor necrosis factor receptor-associated factor (TRAF) proteins are recruited. TRAF proteins trigger two molecular cascades leading to: (i) IRF3 and IRF7 phosphorylation by TANK binding kinase-1 (TBK1) and IκB kinase ε (IKKε) to induce the expression of type I and type III interferons (IFNs) and (ii) NF-κB phosphorylation by the IKK α/β/γ complex to upregulate the expression of inflammatory cytokines. TIM: TRAF-interacting motif. PRR: proline-rich region. ER: endoplasmic reticulum.
The RLR family contains three members: RIG-I, melanoma differentiation associated gene 5 (MDA5), and laboratory of genetics and physiology 2 (LGP2). All RLRs are characterized by a central DEAD box helicase/ATPase domain and a C-terminal regulatory domain (CTD), necessary for RNA binding and to prevent constitutive activation (Tan et al., 2018).
Moreover, RIG-I and MDA5 possess two N-terminal CARDs that mediate their oligomerization following RNA binding and are required for the activation of the downstream adaptor protein MAVS (Brisse and Ly, 2019). RIG-I and MDA5 play non-redundant roles by detecting largely distinct groups of viruses and by recognizing distinct features of viral RNAs. RIG-I preferentially detects 5′-di-/triphosphorylated RNA sequences rich in poly-U or poly-UC tracts, whereas MDA5 binds to high-molecular-weight viral RNAs (Kato et al., 2006). LGP2 exhibits 30–40% amino acid sequence identity to RIG-I and MDA5, but it lacks the CARDs (Yoneyama et al., 2005). The exact role of LGP2 in innate immunity remains not completely understood, since it has been reported to act both as a positive (Venkataraman et al., 2007; Satoh et al., 2010) and as a negative (Komuro and Horvath, 2006; Parisien et al., 2018) regulator of the RLR pathway. To explain these discrepancies, it has been recently proposed that LGP2 may function as a concentration-dependent switch. Early during infection, low levels of LGP2 enhance RLR-mediated antiviral signaling, while, at later stages, IFNs stimulate the expression of LGP2, which, at high concentration, inhibits RLRs, contributing to the termination of the antiviral response (Bruns and Horvath, 2015).
The adaptor protein MAVS (also known as IPS-1/VISA/Cardif) is essential to drive innate immunity in response to RNA virus infection (Figure 1). MAVS is comprised of three functional domains, a CARD at the N terminus, a proline-rich domain, and a C-terminal membrane-targeting transmembrane domain. MAVS contains three TRAF-interacting motifs (TIMs), two in the proline-rich region and one near the transmembrane domain (Seth et al., 2005; Vazquez and Horner, 2015). Through its C-terminal transmembrane domain, MAVS is anchored to different subcellular districts, including the outer mitochondrial membrane (OMM), peroxisomes, and a subdomain of the ER called the mitochondria-associated membrane (MAM) (Seth et al., 2005; Dixit et al., 2010; Horner et al., 2011). It has been proposed that the differential localization of MAVS may drive distinct antiviral signaling responses, with the mitochondrial protein promoting type I IFN expression, while the peroxisomal protein signals for the induction of the type III IFN (Odendall et al., 2014). Other evidence supports the involvement of peroxisomal MAVS in the early induction of IFN-stimulated genes (ISGs), before mitochondrial MAVS establishes a sustained antiviral response (Dixit et al., 2010). Anyhow, these organelles are tightly connected, and mitochondria and peroxisomes interact with each other in signaling “synapses” during activation of the RIG-I pathway (Horner et al., 2011). Moreover, the different localization could also be related to the ability of MAVS to regulate other processes, as in the case of MAM, where MAVS can interact with regulators of cellular metabolism and apoptotic program (Hayashi et al., 2009).
Mitochondrial antiviral signaling-dependent antiviral signaling initiates after RIG-I and MDA5 sense viral RNA in infected cells (Figure 1). In the absence of viral RNA, the CARDs of RIG-I/MDA5 are masked by intramolecular interactions with the helicase domain, but upon viral RNA binding, an ATP-mediated conformational change allows the formation of oligomers, which properly expose their CARDs so as to interact with the equivalent domain of MAVS (Kowalinski et al., 2011). Moreover, RIG-I oligomers form a complex with 14-3-3ε, a mitochondria-targeting chaperone that mediates their translocation from the cytoplasm to OMM and MAM, where MAVS is localized (Liu et al., 2012). The association of RIG-I/MDA5 with MAVS triggers the formation of detergent-insoluble aggregates on the surface of mitochondria (Tang and Wang, 2009). This conformation is the active state of MAVS, necessary for recruiting the downstream effectors TRAF3, TRAF6, TRAF2, and TRAF5 in order to form a signaling supramolecular complex defined as the “MAVS signalosome” (Figure 1) (Hou et al., 2011).
Interestingly, the spontaneous aggregation of MAVS in the absence of viral RNA is prevented by multiple N-terminal truncated proteins that are encoded by the MAVS transcript (Qi et al., 2017). In addition, the inhibition of the short MAVS proteins also results in the autophagic degradation of the full-length protein, highlighting a role of these isoforms in the control of MAVS stability in resting conditions (Brubaker et al., 2014).
Once recruited to the MAVS signalosome, TRAF proteins stimulate two molecular cascades leading to: (a) the phosphorylation of the transcription factors IRF3 and IRF7 by TBK1 and IKKε to induce the expression of type I/III IFNs and (b) the phosphorylation of the transcription factor NF-κB by the IKKα/β/γ complex to upregulate the expression of inflammatory genes (Liu et al., 2013). In turn, type I/III IFNs induce the expression of hundreds of ISGs to establish a protective state, eventually providing viral clearance of the infected cells, while inflammatory cytokines are mainly responsible for regulating the activity of innate and adaptive immune cells (Lazear et al., 2019; Tait Wojno et al., 2019).
Regulation of Mavs Signaling by Post-Translational Modifications
Mass spectrometry-based analyses combined to site-specific mutagenesis approaches have greatly contributed to understand how PTM controls MAVS antiviral response (Figure 2). This has been possible thanks to the improvement of methodologies for PTM enrichment and liquid chromatography-tandem MS (LC-MS/MS)-mediated detection (Yates, 2019). These proteomic studies allowed the identification of hundreds of modification sites in MAVS signaling proteins, whose role in the antiviral response has been extensively investigated, as described hereafter in detail.
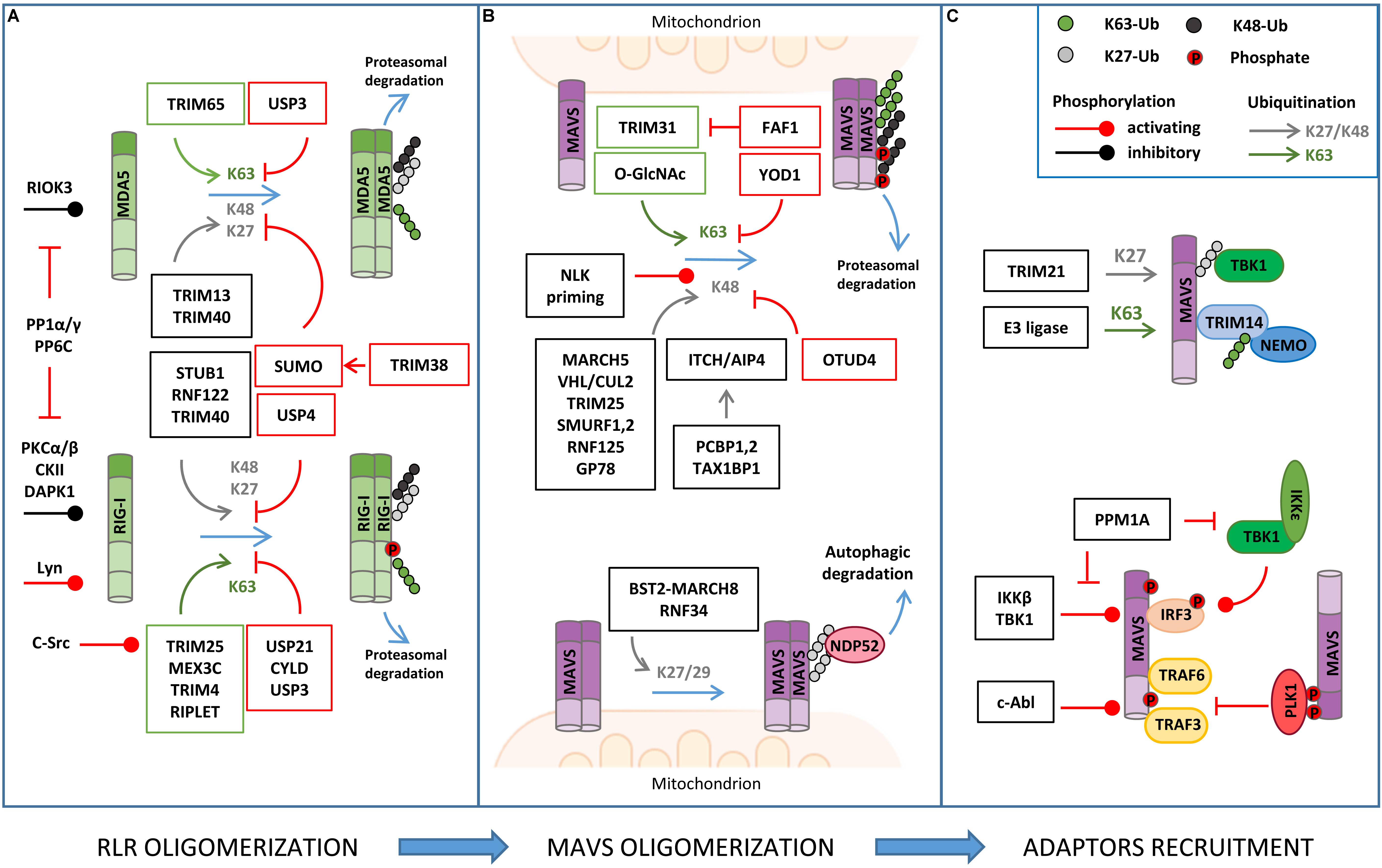
Figure 2. Regulation of RLR–MAVS signaling by post-translational modification (PTM). (A) RLR oligomerization is regulated by different E3 ligases that catalyze lysine 63 (K63)-linked ubiquitination (K63) of both RIG-I and MDA5 to promote oligomerization. K63-linked ubiquitination is countered by different DUBs (USPs, CYLD). K48- or K27-linked ubiquitination directs RIG-I/MDA5 to proteasomal degradation. USP and sumoylation inhibit K48-linked ubiquitination and RLR degradation. Phosphorylation levels regulated by the indicated kinases and phosphatases also regulate RIG/MDA5 oligomerization. (B) MAVS activity is tightly regulated by K63-linked ubiquitination of MAVS, promoting its oligomerization, and K48-linked ubiquitination, which triggers protein degradation. K48-linked ubiquitination is primed by NEMO-like kinase (NLK) and countered by ovarian tumor family deubiquitinase 4 (OTUD4) (upper panel); K27/K29-linked ubiquitination mediates NDP52-dependent autophagic degradation of MAVS (lower panel). (C) MAVS interaction with downstream effectors is regulated by non-degradative ubiquitination (K27- or K63-linked) and phosphorylation, as indicated.
The main PTM responsible for the regulation of RIG-I, MDA5, and MAVS activity is ubiquitination, which acts as either a degradative or regulatory signal, depending on the type of polyubiquitin chain that is formed (Figure 2 and Table 1).
RIG-I/MDA5 Ubiquitination
A series of E3 ubiquitin ligases catalyzing non-degradative lysine 63 (K63)-linked ubiquitination, including TRIM25, RIPLET, TRIM4, and MEX3C, were reported to induce MAVS activity by triggering ubiquitin-dependent RIG-I tetramerization through their CARDs (Okamoto et al., 2017). Interestingly, both covalently linked and unanchored ubiquitin chains were shown to promote RIG-I oligomerization. Unexpectedly, the same E3 ligase was reported to mediate either one or the other type of ubiquitination in independent studies (Oshiumi et al., 2009; Jiang et al., 2012). An explanation for these discrepant results was recently proposed by a co-immunoprecipitation (coIP)/MS-based proteomic study that revealed how the E3 ligase RIPLET can indeed mediate both types of ubiquitination depending on which type of E2 ubiquitin-conjugating enzyme has been bound (i.e. Ube2D3 for covalently linked ubiquitination, Ube2N for unanchored ubiquitination) (Shi et al., 2017). LC-MS/MS analyses have also contributed to identifying lysine residues 48, 96, and 172 in the CARDs of RIG-I that are ubiquitinated by RIPLET and required for MAVS activation (Shi et al., 2017).
K63-linked ubiquitination is also necessary for the oligomerization of MDA5. TRIM65 was identified by an LC-MS/MS-based interactome analysis as the E3 ligase responsible for this regulation by targeting the helicase domain of MDA5 at lysine 743 (Lang et al., 2017). Using a similar coIP/MS approach, TRIM65-mediated MDA5 ubiquitination was found to be stimulated by the interaction with the adaptor protein ARRDC4, whose expression is upregulated in infected cells (Meng et al., 2017).
Retinoic acid-inducible gene I/MDA5-mediated activation of MAVS needs to be tightly regulated in order to ensure the optimal activation and timely termination of innate antiviral response. To this aim, K63-linked ubiquitination of RIG-I and MDA5 is countered by a series of deubiquitinating enzymes (DUBs), such as USP3, USP21, and CYLD (Cui et al., 2014; Fan et al., 2014; Lin et al., 2016). Insights on how DUBs can be turned on during infection came from a study showing that CYLD activity is positively regulated by Syndecan-4 (SDC4), a proteoglycan whose expression is induced by type-I IFN. In a yeast two-hybrid screen, SDC4 was identified as a RIG-I interacting protein that promotes the binding of RIG-I with CYLD in order to decrease K63-linked ubiquitination (Lin et al., 2016).
Degradative ubiquitination also contributes to turning off the mitochondrial antiviral response by regulating the stability of RIG-I and MDA5. The E3 ligases STUB1, RNF122, RNF125, TRIM13, and TRIM40 (the first three identified by proteomic analyses) were shown to mediate lysine 27 (K27)- or lysine 48 (K48)-linked polyubiquitination of RIG-I/MDA5, which results in their proteasomal degradation (Arimoto et al., 2007; Narayan et al., 2014; Wang et al., 2016; Zhao et al., 2016, 2017; Zhou et al., 2018). How degradative ubiquitination is triggered upon RIG-I activation by non-degradative ubiquitination was investigated using an LC-MS/MS approach. This study showed that K63-linked polyubiquitination of RIG-I rapidly enhances its binding to a protein complex containing p97, UFD1, and NPL4, which in turn recruits the E3 ligase RNF125 to trigger K48-linked polyubiquitination of RIG-I and subsequent degradation (Hao et al., 2015).
Retinoic acid-inducible gene I stability is also controlled by autophagy, a catabolic process that plays an important role in the regulation of innate immune response by both degrading invading pathogens and contributing to the termination of the inflammatory response (Tal and Iwasaki, 2009). The autophagic degradation of RIG-I is regulated by leucine-rich repeat containing protein 25 (LRRC25), which binds to RIG-I when it is associated to the IFN-inducible ubiquitin-like protein 15 (ISG15). This protein complex promotes the interaction between RIG-I and the autophagic cargo receptor p62, which is responsible for the selective engulfment of RIG-I by autophagosomes (Du et al., 2018). Interestingly, during infection, this degradative mechanism is transiently counteracted by a different leucine-rich repeat containing protein, LRRC59, which binds to ISG15-associated RIG-I and inhibits the association between LRRC25 and RIG-I (Xian et al., 2019).
Conversely, degradative ubiquitination of MDA5 and RIG-I is negatively regulated by different mechanisms, such as deubiquitination, which is mediated by the DUB USP4 (Wang L. et al., 2013), and sumoylation, which prevents K48-linked ubiquitination of these proteins through the incorporation of the ubiquitin-like (UBL) modifier SUMO, as it was characterized by two-step immunoaffinity purification and LC-MS/MS analyses (Hu et al., 2017).
Recently, NLRP12, a protein of the NLR family, has been reported to negatively control the RLR signaling pathway by modulating both K63- and K48-linked ubiquitination of RIG-I, uncovering an important crosstalk between these innate immune receptor families. In detail, NLRP12 interacts with the ubiquitin ligase TRIM25, preventing RIG-I activation mediated by K63-linked ubiquitination, as well as with RNF125, enhancing K48-linked degradative ubiquitination of RIG-I (Chen et al., 2019). Of note, RIG-I degradation is also a mechanism by which the antiviral response is regulated by members of the lectin family, a different type of PRR that is activated by glycan PAMPs. In particular, the lectin Siglec-G (sialic-acid-binding immunoglobulin-like lectin G) promotes the interaction of the E3 ligase c-Cbl with RIG-I, which mediates its K48-linked ubiquitination in lysine 813 (Chen et al., 2013).
MAVS Ubiquitination
Mitochondrial antiviral signaling is a direct target of both non-degradative and degradative ubiquitination in the course of antiviral response (Figure 2 and Table 1).
The E3 ligase TRIM31 has been identified as a regulator of MAVS aggregation via ubiquitination. During viral infection, TRIM31 is recruited to mitochondria, where it catalyzes K63-linked polyubiquitination of MAVS at lysine residues 10, 311, and 461 to facilitate the formation of prion-like aggregates (Liu et al., 2017). Interestingly, K63-linked ubiquitination of MAVS has been reported to be stimulated by O-GlcNAcylation, highlighting an unappreciated role of glucose metabolism in host innate immune (Li et al., 2018; Song et al., 2019). In detail, LC-MS/MS analysis identified serine 366 as the residue of MAVS where uridine diphosphate N-acetylglucosamine (UDP-GlcNAc) is linked by the O-GlcNAc transferase. Moreover, metabolomic analysis of viral infected macrophages revealed an increase of intermediate metabolites involved in hexosamine biosynthesis, the pathway responsible for the generation of UDP-GlcNAc. The functional relationship between RLRs and glucose metabolism was confirmed by the observation that lactate, the end product of glycolysis in anaerobic conditions, acts a negative regulator of MAVS signaling by preventing RIG-I–MAVS complex formation (Zhang et al., 2019). Interestingly, using a metabolomic approach, it was shown that RLR activation is accompanied by a decrease of glycolysis intermediates, including lactate, which facilitates MAVS-dependent production of type I IFN (Zhang et al., 2019). In line with these findings, inhibition of mitochondrial oxidative phosphorylation results in an impairment of MAVS-mediated induction of IFNs and inflammatory cytokines, further highlighting how antiviral response and mitochondria metabolism are strictly coordinated (Yoshizumi et al., 2017).
Non-degradative ubiquitination also favors the interaction of MAVS with downstream effectors. For example, viral infection induces the expression of the E3 ligase TRIM21, which binds MAVS to promote its K27-linked polyubiquitination at lysine 325 and its association with TBK1 (Xue et al., 2018). Moreover, TRIM proteins regulate the recruitment of IKKγ/NEMO to MAVS signalosome through their own ubiquitination. For example, upon viral infection, TRIM14 undergoes K63-linked polyubiquitination, which provides a platform for the binding of IKKγ/NEMO to MAVS on OMM (Zhou et al., 2014). The temporal kinetics of MAVS oligomerization is controlled by either DUBs or proteins containing UBL domains. YOD1, a deubiquitinase of the ovarian tumor family, is an MAVS interactor identified by coIP/MS that translocates to mitochondria upon viral infection to limit its activation through K63-linked deubiquitination (Liu et al., 2019). UBXN1 and FAF1, two proteins that contain ubiquitin-associated (UBA) or UBL domains, act as steric antagonists of MAVS. The UBA domain of UBXN1 competes with the TRAF3/6-binding sites of MAVS and interferes with its oligomerization (Wang P. et al., 2013). FAF1 forms aggregates through its UBL domain that negatively regulates MAVS by disrupting its association with TRIM31 (Dai et al., 2018).
K48-linked polyubiquitination represents a main mechanism to negatively regulate MAVS signaling by promoting its proteasomal degradation. Lysine 7 was identified as a K48-linked polyubiquitination site on MAVS in a high-resolution proteomic study aimed at investigating endogenous ubiquitination sites through the immuno-enrichment of the di-glycine-lysine remnants that are generated following tryptic digestion of ubiquitinated peptides (Wagner et al., 2012). More recently, it was shown that the mitochondrial E3 ligase MARCH5 binds to MAVS aggregates during viral infection and conjugates K48-linked polyubiquitin chains at lysine residues 7 and 500, leading to their proteasomal degradation (Yoo et al., 2015). Proteasomal degradation of MAVS is also triggered by: (i) the tumor suppressor E3 ligase VHL/Cullin 2 (Du et al., 2015), which promotes K48-linked polyubiquitination at lysine 420 residue, (ii) TRIM29, which mediates K11-linked polyubiquitination at lysine residues 371, 420, and 500 (Xing et al., 2018), and (iii) ITCH/AIP4, which targets lysine residues 371 and 420 with K48-linked polyubiquitination (You et al., 2009). In this regard, yeast two-hybrid screens identify poly rC binding protein 1 (PCBP1) and PCBP2 as positive regulators of the interaction between ITCH/AIP4 and MAVS, whose levels are increased upon viral infection (You et al., 2009). A similar role is played by the adaptor protein TAX1BP1, which is also required for promoting ITCH/AIP4-mediated ubiquitination of MAVS (Choi et al., 2017). Interestingly, TRIM25, an E3 ligase responsible for K63 ubiquitination of RIG-I, has also been identified as a MAVS binding partner using GeLC-MS/MS (1D SDS PAGE gel followed by LC-MS/MS). In particular, TRIM25 mediates K48-linked ubiquitination of MAVS at lysine residues 7 and 10, suggesting that the same E3 ligase can initially trigger the antiviral response and, later on, be responsible for its termination by inducing MAVS proteasomal degradation (Castanier et al., 2012). Other E3 ubiquitin ligases that catalyze K48 ubiquitination of MAVS are SMURF1, SMURF2, Ring Finger protein (RNF) 125, and GP78, but the ubiquitination sites have not been determined yet (Arimoto et al., 2007; Wang et al., 2012; Jacobs et al., 2014; Pan et al., 2014). Degradative ubiquitination is counteracted by DUBs, as shown for ovarian tumor family deubiquitinase 4 (OTUD4), whose expression is increased by IRF3/7 in a positive-feedback loop (Liuyu et al., 2019).
Ubiquitination can also trigger the autophagic degradation of MAVS. The E3 ubiquitin RNF34 promotes K27/K29-linked polyubiquitination on MAVS at lysine residues 297, 311, 348, and 362. Ubiquitinated MAVS-enriched mitochondria are then recognized by the autophagy receptor NDP52 and delivered to autophagosomes for degradation (He et al., 2019). Similarly, the IFN-induced Tetherin (also known as BST2) recruits the E3 ligase MARCH8 to mediate K27-linked ubiquitination of MAVS at lysine 7 and subsequent NDP52-dependent autophagic degradation, which is important to prevent excessive activation of RLR signaling (Jin et al., 2017).
RIG-I/MDA5 Phosphorylation
Protein phosphorylation is a second main type of PTM that controls MAVS signaling during viral infection (Figure 2). This regulation is important to modulate both the binding of RIG-I/MDA5 to MAVS and the recruitment of downstream effectors to assemble the MAVS signalosome (Oshiumi et al., 2016).
On one hand, tyrosine kinases of the SRC family have been shown to stimulate RIG-I activity. CoIP/MS analyses identified LYN as a binding partner of both RIG-I and MAVS in macrophages upon treatment with synthetic analogs of double-stranded RNA (Lim et al., 2015). In this condition, LYN phosphorylates RIG-I at tyrosine 396 and promotes its oligomerization. c-SRC was also observed to stimulate RIG-I, but it acts in an indirect manner (Lee et al., 2018). In fact, c-SRC phosphorylates TRIM25 at tyrosine 278, thus stimulating K63-linked ubiquitination and oligomerization of RIG-I.
On the other hand, RIG-I/MDA5 phosphorylation in serine and threonine is mainly associated to the inhibition of their activity in resting conditions. The negative roles of this type of phosphorylation were highlighted by an LC-MS/MS analysis of phosphorylated peptides differentially present in ubiquitinated and non-ubiquitinated RIG-I. This study identified, among others, serine 8 and threonine 170 as phosphorylated sites that negatively regulate the ubiquitination on lysine 172 by preventing the binding of TRIM25 (Gack et al., 2010; Nistal-Villán et al., 2010). These amino acids are phosphorylated by protein kinase C-α (PKC-α) and PKC-β (Maharaj et al., 2012). Inhibitory phosphorylations were also identified at threonine 770, serine 854, and serine 855 at the C-term domain RIG-I, residues that are phosphorylated by casein kinase II (CK2), and at serine 828 of MDA5, which is carried out by RIO kinase 3 (RIOK3) (Sun et al., 2011; Takashima et al., 2015). In both cases, phosphorylation prevents the oligomerization of these sensor proteins.
Early during viral infection, serine/threonine phosphorylation levels of RIG-I/MDA5 are decreased by PP1α and PP1γ, two protein phosphatases that have been identified through a phosphatome RNAi screen (Wies et al., 2013). A combination of RNA interference, yeast two-hybrid, and APEX2 proximity labeling-based MS approaches also identified PPP6C as a phosphatase that controls RIG-I activity. In particular, PPP6C forms a complex with WHIP and TRIM14, which mediate the binding of PPP6C to ubiquitinated RIG-I and MAVS, respectively (Tan et al., 2017). At later stages of infection, the inhibitory phosphorylations are re-established and contribute to turning off MAVS signaling. This event is regulated by death associated protein kinase 1 (DAPK1), whose activation is triggered by RIG-I signaling as a negative-feedback mechanism (Willemsen et al., 2017).
MAVS Phosphorylation
Various phosphorylations of MAVS have also been characterized with either positive or negative effects on its function (Figure 2 and Table 1).
Stimulatory phosphorylations were identified by LC-MS/MS analyses at serine residues 442, 444, and 44 in the C terminus of MAVS, which are phosphorylated by TBK1 and IKKβ upon viral infection. This modification is needed to recruit IRF3 on MAVS signalosome and facilitate its phosphorylation by TBK1 (Liu et al., 2015). Phosphorylation-driven formation of MAVS signaling complex is countered by PPM1A (protein phosphatase magnesium-dependent 1A, also known as PP2Cα), which targets both MAVS and TBK1/IKKε for dephosphorylation (Xiang et al., 2016).
c-ABL has been reported to phosphorylate MAVS at tyrosine 9, promoting the formation of MAVS/TRAF3/TRAF6 complex and the activation of the antiviral response (Song et al., 2010). Recently, the role of c-ABL-mediated phosphorylation of MAVS has been further investigated in the context of microglia inflammation, where this kinase promotes MAVS activity by disrupting its interaction with the autophagosome protein LC3 and preventing autophagy-mediated MAVS degradation (Cheng et al., 2017). Additional activating phosphorylations of MAVS have been identified by proteomic approaches, but the molecular mechanism underlying their effect remains to be elucidated. For example, a quantitative analysis of phospho-peptides using iTRAQ labeling identified serine residues 328 and 330 in mouse MAVS sequence as required for the stimulation of IRF3 and NF-κB activity (Kandasamy et al., 2016).
Conversely, phosphorylation of threonine 234 and serine 233 of MAVS was shown to inhibit the antiviral response by serving as a docking site for the recruitment of mitotic kinase Polo-like kinase 1 (PLK1). The PLK1–MAVS interaction, identified through a yeast two-hybrid screen, interferes with the binding of MAVS with TRAF3 and attenuates IFN signaling during viral infection as well as during the G2/M phase of the cell cycle (Vitour et al., 2009). Recently, an LC-MS/MS study showed that MAVS phosphorylation could also prime MAVS for proteasomal degradation. This is mediated by the NEMO-like kinase (NLK), which targets MAVS at serine residues 121, 212, 258, and 329 (Li et al., 2019).
RIG-I Acetylation
A third type of PTM that has been shown to regulate MAVS signaling is protein acetylation. LC-MS/MS analysis identified lysine residues 858 and 909 as acetylated residues of RIG-I that contribute to preventing its oligomerization in resting conditions. The acetyltransferases responsible for this PTM remain to be characterized, while HDAC6 was identified as the deacetylase required for removal of these acetyl groups during viral infection to allow RIG-I activation (Choi et al., 2016; Liu et al., 2016).
Regulation of MAVS Signaling by Protein–Protein Interaction
Cellular Protein Interactions
In addition to PTMs, proteomic analyses have provided a large set of information on how MAVS signaling is regulated by direct protein–protein interactions with cellular and viral factors (Figure 3). Interactomics studies were carried out using either antibody-based affinity pull-down combined with MS analyses, which allows the characterization of protein complexes in a physiological context but favoring stable associations, or yeast two-hybrid assays, which also detect transient or labile interactions but assessed in non-physiological cell systems (Mehta and Trinkle-Mulcahy, 2016).
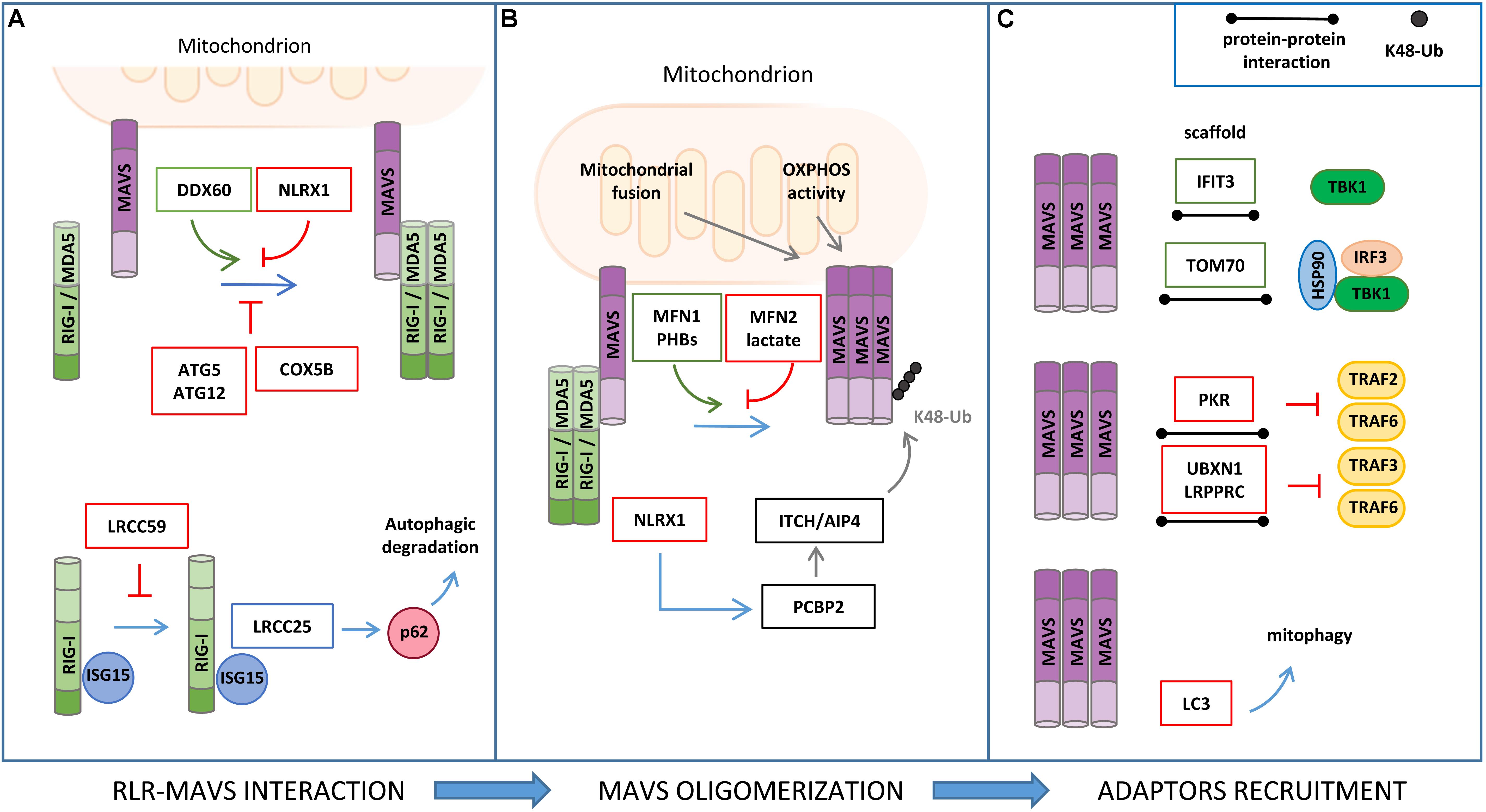
Figure 3. Regulation of RLR–MAVS signaling by protein–protein interaction. (A) RIG-I/MDA5 interaction with MAVS is facilitated by DDX60 and inhibited by NLRX1, ATG5/ATG12 complex, and COX5B (upper panel). ISG15 association to RIG-I promotes leucine-rich repeat containing protein 25 (LRRC25) interaction and p62-mediated autophagic degradation of RIG-I, which is inhibited by LRCC59 (lower panel). (B) Mitochondrial fusion and oxidative phosphorylation (OXPHOS) are required for efficient MAVS signaling. In particular, MFN1 and PHB1 and PHB2 act as positive regulators of MAVS signaling, while MFN2, lactate, and NLRX1 negatively regulate MAVS activity. (C) Interferon Induced Protein With Tetratricopeptide Repeats 3 (IFIT3) and TOM70 interact with MAVS and function as a scaffold for the recruitment of downstream effectors; protein kinase R (PKR), UBXN1, and LRPPRC interact with MAVS, inhibiting TRAF association (upper and middle panels); LC3–MAVS interaction promotes mitochondrial degradation by mitophagy (lower panels).
Various studies have reported that ISGs that are induced upon MAVS activation may establish a positive-feedback loop to potentiate the antiviral signaling at multiple steps (Crosse et al., 2018). For example, the ISG protein DDX60 is an RNA helicase that, upon binding with double-strand RNA, associates with RIG-I, MDA5, and LGP2, and increases MAVS activation (Miyashita et al., 2011). The shorter isoform of zinc-finger antiviral protein (S-ZAP) is an ISG that associates with RIG-I to promote its oligomerization and activity (Hayakawa et al., 2011). Interferon Induced Protein With Tetratricopeptide Repeats 3 (IFIT3) localizes to mitochondria, where it functions as a scaffold to facilitate the interaction of MAVS with TBK1 (Liu et al., 2011). The dsRNA-dependent protein kinase R (PKR) is upregulated by type I and type III IFNs and induces MAVS signaling by interacting with TRAF2 and TRAF6 to promote NF-κB activation (Gil et al., 2004).
The cytoskeletal network is another source of proteins that were found to stimulate the antiviral response by interacting with components of MAVS signaling. Focal adhesion kinase (FAK) is an actin-associated tyrosine kinase that binds to MAVS in a viral infection-dependent manner and potentiates its signaling independently of its kinase activity (Bozym et al., 2012). Two microtubule proteins, Rho guanine nucleotide exchange factor 2 (GEF-H1) and HAUS augmin-like complex subunit 8 (HAUS8), also act as positive regulators of the RLR pathway by stimulating TBK1 activity and MAVS ubiquitination, respectively (Chiang et al., 2014; He et al., 2018).
A recent study in which global RNA interference analyses were combined to experimentally and computationally derive interactome data has provided an in-depth view of the RIG-I protein interaction network and identified new processes that influence the host response to viral RNA, including the unfolded protein response, WNT/β-catenin signaling, and RNA metabolism. The role of K-Homology Splicing Regulatory Protein (KHSRP), one of the best hits obtained from this screening, was further characterized, showing that its interaction with RIG-I is required to maintain the receptor in an inactive state in resting conditions (Soonthornvacharin et al., 2017).
The mitochondrial resident factors NLRX1, LRPPRC, MFN1, MFN2, TOM70, PHB1/2, and LONP1 have been described to interact with MAVS and regulate the antiviral signaling.
NLRX1 (nucleotide-binding domain and leucine-rich repeat containing family member) was the first mitochondrial protein identified as a negative regulator of MAVS (Allen et al., 2011). NLRX1 interacts with MAVS via its CARD and interferes with the binding of MAVS with the upstream partners RIG-I and MDA5. However, the role of NLRX1 in MAVS signaling has long been debated. NLRX1-deficient mice exhibit unaltered antiviral and inflammatory responses to Sendai virus, influenza A virus, or synthetic analogs of double-stranded RNA injection when compared to wild-type mice (Rebsamen et al., 2011; Soares et al., 2013). In addition, NLRX1 may also play a positive role in innate immune signaling mediated by NF-κB through the generation of ROS, which is supported by the observations that NLRX1 is mainly localized to the mitochondrial matrix and interacts with UQCRC2, a protein of the respiratory chain complex III (Arnoult et al., 2009). More recently, the mechanism by which NLRX1 negatively regulates the antiviral response has been refined by studying hepatitis C virus (HCV) infection. In this context, NLRX1 functions as a bridging protein between MAVS and PCBP2, thus promoting the ITCH/AIP4-mediated K48-linked polyubiquitination and degradation of MAVS (Qin et al., 2017).
LRPPRC, a protein known to regulate mitochondrial RNA stability, has been characterized as a negative regulator of the mitochondrial-mediated antiviral immunity during HCV infection. In particular, LRPPRC interacts with MAVS and inhibits its signaling by preventing the association with TRAF3 and TRAF6 (Refolo et al., 2019).
The mitochondrial fusion protein MFN2 has been identified as a negative regulator of MAVS-mediated antiviral signaling through an LC-MS/MS-based interactome analysis. MFN2 binds to MAVS, preventing its oligomerization and the propagation of the downstream antiviral response (Yasukawa et al., 2009). This MFN2 function is distinct from its role in mitochondrial dynamics. In fact, the inhibition of mitochondrial fusion by the concomitant downregulation of MFN1 and MFN2 expression (Koshiba et al., 2011), as well as that of OPA1 (Yoshizumi et al., 2017), leads to the repression of MAVS activity, which is associated to a dissipation of mitochondrial membrane potential. Moreover, MFN1 was shown to physically interact with MAVS and promote RIG-I-mediated MAVS oligomerization, suggesting also a direct involvement of MFN1 in the induction of the mitochondrial antiviral response (Onoguchi et al., 2010).
Analysis of MAVS interacting proteins upon mitochondrial enrichment and GeLC-MS/MS identified the mitochondrial protein TOM70 (translocase of outer membrane 70) as a novel component of the MAVS signalosome. In detail, Tom70 acts as a promoting factor of the antiviral response by functioning as a scaffold for the recruitment of both IRF3 and TBK1, in association with HSP90, to MAVS (Liu et al., 2010).
A positive role of the inner mitochondrial membrane (IMM) proteins PROHIBITIN 1 (PHB1) and PHB2 in MAVS signaling has recently emerged in a proteomic study aimed at characterizing the function of the coiled coil domains of these mitochondrial scaffold proteins. Upon viral infection, PHBs were shown to form a complex with MAVS oligomers, which also includes the AAA(+) ATPase proteins Caseinolytic Peptidase B (CLPB) Homolog and ATPase Family AAA Domain Containing 3A (ATAD3A), thus establishing a bridge between the OMM and IMM that is required for the efficient activation of the RIG-I signaling pathway (Yoshinaka et al., 2019).
The mitochondrial protease LONP1 has been identified as a positive regulator of MAVS in a coIP/MS-based analysis from purified MAM, a study that was performed using surfactants hydrolyzable at low pH to reduce detergent interference in MS (Horner et al., 2015). However, the molecular mechanism underlying this regulation remains to be characterized.
Mitochondrial antiviral signaling activity is also regulated by autophagy through protein–protein interaction. MAVS directly binds to the autophagosome protein LC3 through an LC3-interaction region (LIR) at the MAVS N-terminal region. Through this interaction, MAVS was reported to function as a mitophagy receptor for the engulfment of mitochondria that are damaged upon excessive activation of antiviral signaling (Sun et al., 2016). Moreover, the autophagy proteins ATG5–ATG12 were found to downregulate RIG-I signaling by interacting with the CARDs of both RIG-I and MAVS (Jounai et al., 2007). Interestingly, the mitochondrial protein COX5B has been shown to act in concert with ATG5 in the suppression of MAVS activity both through direct protein interaction and, indirectly, by decreasing ROS levels (Zhao et al., 2012).
The identification of gC1qR (receptor for globular head domain of complement component C1q) and Sorting nexin 8 (SNX8) as MAVS interacting proteins that regulate the antiviral response suggests a role of other signaling pathways to MAVS activity that remain to be contextualized (Xu et al., 2009; Guo et al., 2019).
Viral Protein Interactions
Viruses have evolved different mechanisms to inhibit MAVS activity as part of their immune evasion strategies. Viral proteins are able to interfere with the binding of MAVS to the upstream RLR sensors. The non-structural protein NS4A of Dengue and Zika viruses binds to the CARD of MAVS, preventing RIG-I/MDA5 accessibility and hampering RLR signal transduction (He et al., 2016; Ma et al., 2018). Zika NS3 also contributes to the inhibition of MAVS activation, as demonstrated in an interactome study where NS3 was found to bind and inhibit the function of 14-3-3ε, a docking protein required for the translocation of RIG-I/MDA5 to mitochondria (Riedl et al., 2019).
We have recently characterized the interactome of HCV NS5A using an HCV replicon cell system in which a double tag was inserted in the viral genome in a region of NS5A that did not alter its ability to support viral replication. NS5A coIP was carried out using n-dodecyl β-D-maltoside as a detergent to preserve the integrity of mitochondrial membrane complexes. We found that HCV NS5A interacts with the mitochondrial protein LRPPRC and represses the antiviral response by promoting LRPPRC association with MAVS, which results in a reduced association of MAVS with TRAF proteins (Refolo et al., 2019).
A peculiar mechanism to elude MAVS activity has been described for human immunodeficiency virus 1 (HIV-1) in dendritic cells. In these cells, the RNA helicase DDX3 acts as an intracellular sensor of abortive HIV-1 RNAs that stimulates MAVS activity by promoting its interaction with TRAF3. HIV-1 is able to block this pathway through the HIV-1 envelope protein GP120, which, by binding to the C-type lectin receptor DC-SIGN, activates PLK1 to interfere with the formation of MAVS–TRAF3 complex (Gringhuis et al., 2017).
Finally, a large series of viral proteases induces the proteolytic cleavage of MAVS to release it from the outer membrane of mitochondria. These include: HCV NS3/4A viral protease, which cleaves MAVS at cysteine 508 (Li et al., 2005); hepatitis A virus (HAV) 3ABC precursor of 3Cpro cysteine protease (Yang et al., 2007); 3Cpro cysteine protease of coxsackievirus B3 (CVB3), which cleaves MAVS at Q148 within the proline-rich region (Mukherjee et al., 2011); and enterovirus 71 (EV71) 2A protease (2Apro), which cleaves MAVS on multiple residues, at Gly209, Gly251, and Gly265 (Wang B. et al., 2013). A different inhibitory mechanism has been described for the influenza A virus PB1-F2 protein and the human cytomegalovirus (HCMV) glycoprotein US9, which interact with MAVS and inhibit its activity by dissipating the mitochondrial membrane potential (Varga et al., 2012; Choi et al., 2018).
Concluding Remarks
The mitochondrial proteome is a highly dynamic entity that undergoes profound changes in response to a variety of stress conditions in the attempt to mount an adaptive response or, when not feasible, to trigger programmed cell death. In this review, we discussed recent findings describing how the host cell response to RNA virus infection is centered on the mitochondrial outer membrane. In this subcellular compartment, MAVS signalosome is rapidly assembled to recruit key components of IRFs and NF-κB signaling pathways and induces the expression of hundreds of genes with antiviral properties. In particular, we highlighted how proteomic approaches have contributed to the characterization of protein networks and their PTMs that regulate MAVS signaling. In addition, for its unbiased characteristics, proteomics has provided several new insights on the crosstalk of the mitochondrial antiviral response with other PPRs, such as NLR and lectins, or other cellular processes, such as glucose metabolism. The key contribution of proteomic studies to unraveling the complexity of the antiviral response is expected to have an important impact on the development of novel therapeutic strategies aimed at suppressing viral infection and enhancing immune responses.
Author Contributions
GR, GF, and FC wrote the text and prepared the figures. TV and MP contributed to literature search and revised the manuscript and discussion.
Funding
This work was supported in part by grants from AIRC (IG2015 n. 17404 to GF and IG2014 n. 15244 to MP), the Italian Ministry of University and Research (FIRB Accordi di Programma 2011 to MP and PRIN 2015 20152CB22L to GF), the Italian Ministry of Health (Ricerca Corrente to MP and GF, Ricerca Finalizzata GR-2013-02359524 to TV), Fondazione Fibrosi Cistica (Progetto FFC#8/2018 to MP), Regione Lazio (Progetto “Gruppi di Ricerca” 2018 to MP), and Transautophagy COST action CA15138 to GF.
Conflict of Interest
The authors declare that the research was conducted in the absence of any commercial or financial relationships that could be construed as a potential conflict of interest.
References
Allen, I. C., Moore, C. B., Schneider, M., Lei, Y., Davis, B. K., Scull, M. A., et al. (2011). NLRX1 protein attenuates inflammatory responses to infection by interfering with the RIG-I-MAVS and TRAF6-NF-κB signaling pathways. Immunity 34, 854–865. doi: 10.1016/j.immuni.2011.03.026
Arimoto, K., Takahashi, H., Hishiki, T., Konishi, H., Fujita, T., and Shimotohno, K. (2007). Negative regulation of the RIG-I signaling by the ubiquitin ligase RNF125. Proc. Natl. Acad. Sci. U.S.A. 104, 7500–7505. doi: 10.1073/pnas.0611551104
Arnoult, D., Soares, F., Tattoli, I., Castanier, C., Philpott, D. J., and Girardin, S. E. (2009). An N-terminal addressing sequence targets NLRX1 to the mitochondrial matrix. J. Cell Sci. 122, 3161–3168. doi: 10.1242/jcs.051193
Bozym, R. A., Delorme-Axford, E., Harris, K., Morosky, S., Ikizler, M., Dermody, T. S., et al. (2012). Focal adhesion kinase is a component of antiviral RIG-I-like receptor signaling. Cell Host Microbe 11, 153–166. doi: 10.1016/j.chom.2012.01.008
Brisse, M., and Ly, H. (2019). Comparative structure and function analysis of the RIG-I-Like receptors: RIG-I and MDA5. Front. Immunol. 10:01586. doi: 10.3389/fimmu.2019.01586
Brubaker, S. W., Bonham, K. S., Zanoni, I., and Kagan, J. C. (2015). Innate immune pattern recognition: a cell biological perspective. Annu. Rev. Immunol. 33, 257–290. doi: 10.1146/annurev-immunol-032414-112240
Brubaker, S. W., Gauthier, A. E., Mills, E. W., Ingolia, N. T., and Kagan, J. C. (2014). A bicistronic MAVS transcript highlights a class of truncated variants in antiviral immunity. Cell 156, 800–811. doi: 10.1016/j.cell.2014.01.021
Bruns, A. M., and Horvath, C. M. (2015). LGP2 synergy with MDA5 in RLR-mediated RNA recognition and antiviral signaling. Cytokine 74, 198–206. doi: 10.1016/j.cyto.2015.02.010
Castanier, C., Zemirli, N., Portier, A., Garcin, D., Bidère, N., Vazquez, A., et al. (2012). MAVS ubiquitination by the E3 ligase TRIM25 and degradation by the proteasome is involved in type I interferon production after activation of the antiviral RIG-I-like receptors. BMC Biol. 10:44. doi: 10.1186/1741-7007-10-44
Chen, W., Han, C., Xie, B., Hu, X., Yu, Q., Shi, L., et al. (2013). Induction of Siglec-G by RNA viruses inhibits the innate immune response by promoting RIG-I degradation. Cell 152, 467–478. doi: 10.1016/j.cell.2013.01.011
Chen, S.-T., Chen, L., Lin, D. S.-C., Chen, S.-Y., Tsao, Y.-P., Guo, H., et al. (2019). NLRP12 regulates anti-viral RIG-I activation via interaction with TRIM25. Cell Host Microbe 25, 602.e7–616.e7. doi: 10.1016/j.chom.2019.02.013
Cheng, J., Liao, Y., Xiao, L., Wu, R., Zhao, S., Chen, H., et al. (2017). Autophagy regulates MAVS signaling activation in a phosphorylation-dependent manner in microglia. Cell Death Differ. 24, 276–287. doi: 10.1038/cdd.2016.121
Chiang, H.-S., Zhao, Y., Song, J.-H., Liu, S., Wang, N., Terhorst, C., et al. (2014). GEF-H1 controls microtubule-dependent sensing of nucleic acids for antiviral host defenses. Nat. Immunol. 15, 63–71. doi: 10.1038/ni.2766
Choi, H. J., Park, A., Kang, S., Lee, E., Lee, T. A., Ra, E. A., et al. (2018). Human cytomegalovirus-encoded US9 targets MAVS and STING signaling to evade type I interferon immune responses. Nat. Commun. 9:125. doi: 10.1038/s41467-017-02624-8
Choi, S. J., Lee, H.-C., Kim, J.-H., Park, S. Y., Kim, T.-H., Lee, W.-K., et al. (2016). HDAC6 regulates cellular viral RNA sensing by deacetylation of RIG-I. EMBO J. 35, 429–442. doi: 10.15252/embj.201592586
Choi, Y. B., Shembade, N., Parvatiyar, K., Balachandran, S., and Harhaj, E. W. (2017). TAX1BP1 restrains virus-induced apoptosis by facilitating itch-mediated degradation of the mitochondrial adaptor MAVS. Mol. Cell. Biol. 37:e00422-16. doi: 10.1128/mcb.00422-16
Crosse, K. M., Monson, E. A., Beard, M. R., and Helbig, K. J. (2018). Interferon-stimulated genes as enhancers of antiviral innate immune signaling. J. Innate Immun. 10, 85–93. doi: 10.1159/000484258
Cui, J., Song, Y., Li, Y., Zhu, Q., Tan, P., Qin, Y., et al. (2014). USP3 inhibits type I interferon signaling by deubiquitinating RIG-I-like receptors. Cell Res. 24, 400–416. doi: 10.1038/cr.2013.170
Dai, T., Wu, L., Wang, S., Wang, J., Xie, F., Zhang, Z., et al. (2018). FAF1 regulates antiviral immunity by inhibiting MAVS but is antagonized by phosphorylation upon viral infection. Cell Host Microbe 24, 776.e5–790.e5. doi: 10.1016/j.chom.2018.10.006
Dixit, E., Boulant, S., Zhang, Y., Lee, A. S. Y., Odendall, C., Shum, B., et al. (2010). Peroxisomes are signaling platforms for antiviral innate immunity. Cell 141, 668–681. doi: 10.1016/j.cell.2010.04.018
Du, J., Zhang, D., Zhang, W., Ouyang, G., Wang, J., Liu, X., et al. (2015). pVHL negatively regulates antiviral signaling by targeting MAVS for proteasomal degradation. J. Immunol. 195, 1782–1790. doi: 10.4049/jimmunol.1500588
Du, Y., Duan, T., Feng, Y., Liu, Q., Lin, M., Cui, J., et al. (2018). LRRC25 inhibits type I IFN signaling by targeting ISG15-associated RIG-I for autophagic degradation. EMBO J. 37, 351–366. doi: 10.15252/embj.201796781
Fan, Y., Mao, R., Yu, Y., Liu, S., Shi, Z., Cheng, J., et al. (2014). USP21 negatively regulates antiviral response by acting as a RIG-I deubiquitinase. J. Exp. Med. 211, 313–328. doi: 10.1084/jem.20122844
Franchi, L., Muñoz-Planillo, R., and Núñez, G. (2012). Sensing and reacting to microbes through the inflammasomes. Nat. Immunol. 13, 325–332. doi: 10.1038/ni.2231
Gack, M. U., Nistal-Villan, E., Inn, K.-S., Garcia-Sastre, A., and Jung, J. U. (2010). Phosphorylation-mediated negative regulation of RIG-I antiviral activity. J. Virol. 84, 3220–3229. doi: 10.1128/jvi.02241-09
Gil, J., Garcia, M. A., Gomez-Puertas, P., Guerra, S., Rullas, J., Nakano, H., et al. (2004). TRAF family proteins link PKR with NF- B activation. Mol. Cell. Biol. 24, 4502–4512. doi: 10.1128/mcb.24.10.4502-4512.2004
Gringhuis, S. I., Hertoghs, N., Kaptein, T. M., Zijlstra-Willems, E. M., Sarrami-Fooroshani, R., Sprokholt, J. K., et al. (2017). HIV-1 blocks the signaling adaptor MAVS to evade antiviral host defense after sensing of abortive HIV-1 RNA by the host helicase DDX3. Nat. Immunol. 18, 225–235. doi: 10.1038/ni.3647
Guo, W., Wei, J., Zhong, X., Zang, R., Lian, H., Hu, M.-M., et al. (2019). SNX8 modulates the innate immune response to RNA viruses by regulating the aggregation of VISA. Cell. Mol. Immunol. [Epub ahead of print].
Hao, Q., Jiao, S., Shi, Z., Li, C., Meng, X., Zhang, Z., et al. (2015). A non-canonical role of the p97 complex in RIG-I antiviral signaling. EMBO J. 34, 2903–2920. doi: 10.15252/embj.201591888
Hayakawa, S., Shiratori, S., Yamato, H., Kameyama, T., Kitatsuji, C., Kashigi, F., et al. (2011). ZAPS is a potent stimulator of signaling mediated by the RNA helicase RIG-I during antiviral responses. Nat. Immunol. 12, 37–44. doi: 10.1038/ni.1963
Hayashi, T., Rizzuto, R., Hajnoczky, G., and Su, T.-P. (2009). MAM: more than just a housekeeper. Trends Cell Biol. 19, 81–88. doi: 10.1016/j.tcb.2008.12.002
He, T.-S., Chen, T., Wang, D.-D., and Xu, L.-G. (2018). HAUS8 regulates RLR-VISA antiviral signaling positively by targeting VISA. Mol. Med. Rep. 18, 2458–2466. doi: 10.3892/mmr.2018.9171
He, X., Zhu, Y., Zhang, Y., Geng, Y., Gong, J., Geng, J., et al. (2019). RNF34 functions in immunity and selective mitophagy by targeting MAVS for autophagic degradation. EMBO J. 38, e100978. doi: 10.15252/embj.2018100978
He, Z., Zhu, X., Wen, W., Yuan, J., Hu, Y., Chen, J., et al. (2016). Dengue virus subverts host innate immunity by targeting adaptor protein MAVS. J. Virol. 90, 7219–7230. doi: 10.1128/jvi.0022116
Horner, S. M., Liu, H. M., Park, H. S., Briley, J., and Gale, M. (2011). Mitochondrial-associated endoplasmic reticulum membranes (MAM) form innate immune synapses and are targeted by hepatitis C virus. Proc. Natl. Acad. Sci. U.S.A. 108, 14590–14595. doi: 10.1073/pnas.1110133108
Horner, S. M., Wilkins, C., Badil, S., Iskarpatyoti, J., and Gale, M. (2015). Proteomic analysis of mitochondrial-associated ER membranes (MAM) during RNA virus infection reveals dynamic changes in protein and organelle trafficking. PLoS One 10:e0117963. doi: 10.1371/journal.pone.0117963
Hou, F., Sun, L., Zheng, H., Skaug, B., Jiang, Q.-X., and Chen, Z. J. (2011). MAVS forms functional prion-like aggregates to activate and propagate antiviral innate immune response. Cell 146, 448–461. doi: 10.1016/j.cell.2011.06.041
Hu, M.-M., Liao, C.-Y., Yang, Q., Xie, X.-Q., and Shu, H.-B. (2017). Innate immunity to RNA virus is regulated by temporal and reversible sumoylation of RIG-I and MDA5. J. Exp. Med. 214, 973–989. doi: 10.1084/jem.20161015
Jacobs, J. L., Zhu, J., Sarkar, S. N., and Coyne, C. B. (2014). Regulation of mitochondrial antiviral signaling (MAVS) expression and signaling by the mitochondria-associated endoplasmic reticulum membrane (MAM) protein Gp78. J. Biol. Chem. 289, 1604–1616. doi: 10.1074/jbc.M113.520254
Jiang, X., Kinch, L. N., Brautigam, C. A., Chen, X., Du, F., Grishin, N. V., et al. (2012). Ubiquitin-induced oligomerization of the RNA sensors RIG-I and MDA5 activates antiviral innate immune response. Immunity 36, 959–973. doi: 10.1016/j.immuni.2012.03.022
Jin, S., Tian, S., Luo, M., Xie, W., Liu, T., Duan, T., et al. (2017). Tetherin suppresses type I interferon signaling by Targeting MAVS for NDP52-mediated selective autophagic degradation in human cells. Mol. Cell 68, 308.e4–322.e4. doi: 10.1016/j.molcel.2017.09.005
Jounai, N., Takeshita, F., Kobiyama, K., Sawano, A., Miyawaki, A., Xin, K.-Q., et al. (2007). The Atg5 Atg12 conjugate associates with innate antiviral immune responses. Proc. Natl. Acad. Sci. U.S.A. 104, 14050–14055. doi: 10.1073/pnas.0704014104
Kandasamy, R. K., Vladimer, G. I., Snijder, B., Müller, A. C., Rebsamen, M., Bigenzahn, J. W., et al. (2016). A time-resolved molecular map of the macrophage response to VSV infection. NPJ Syst. Biol. Appl. 2, 16027. doi: 10.1038/npjsba.2016.27
Kato, H., Takeuchi, O., Sato, S., Yoneyama, M., Yamamoto, M., Matsui, K., et al. (2006). Differential roles of MDA5 and RIG-I helicases in the recognition of RNA viruses. Nature 441, 101–105. doi: 10.1038/nature04734
Kawai, T., and Akira, S. (2011). Toll-like Receptors and Their Crosstalk with Other Innate Receptors in Infection and Immunity. Immunity 34, 637–650. doi: 10.1016/j.immuni.2011.05.006
Komuro, A., and Horvath, C. M. (2006). RNA- and Virus-Independent Inhibition of Antiviral Signaling by RNA Helicase LGP2. J. Virol. 80, 12332–12342. doi: 10.1128/jvi.01325-06
Koshiba, T., Yasukawa, K., Yanagi, Y., and Kawabata, S. I. (2011). Mitochondrial membrane potential is required for MAVS-mediated antiviral signaling. Sci. Signal 4, ra7. doi: 10.1126/scisignal.2001147
Kowalinski, E., Lunardi, T., McCarthy, A. A., Louber, J., Brunel, J., Grigorov, B., et al. (2011). Structural basis for the activation of innate immune pattern-recognition receptor RIG-I by viral RNA. Cell 147, 423–435. doi: 10.1016/j.cell.2011.09.039
Lang, X., Tang, T., Jin, T., Ding, C., Zhou, R., and Jiang, W. (2017). TRIM65-catalized ubiquitination is essential for MDA5-mediated antiviral innate immunity. J. Exp. Med. 214, 459–473. doi: 10.1084/jem.20160592
Lazear, H. M., Schoggins, J. W., and Diamond, M. S. (2019). Shared and distinct functions of type I and type III interferons. Immunity 50, 907–923. doi: 10.1016/j.immuni.2019.03.025
Lee, N.-R., Choi, J.-Y., Yoon, I.-H., Lee, J. K., and Inn, K.-S. (2018). Positive regulatory role of c-Src-mediated TRIM25 tyrosine phosphorylation on RIG-I ubiquitination and RIG-I-mediated antiviral signaling pathway. Cell. Immunol. 332, 94–100. doi: 10.1016/j.cellimm.2018.08.004
Li, S.-Z., Shu, Q.-P., Song, Y., Zhang, H.-H., Liu, Y., Jin, B.-X., et al. (2019). Phosphorylation of MAVS/VISA by Nemo-like kinase (NLK) for degradation regulates the antiviral innate immune response. Nat. Commun. 10:3233. doi: 10.1038/s41467-019-11258-x
Li, X.-D., Sun, L., Seth, R. B., Pineda, G., and Chen, Z. J. (2005). Hepatitis C virus protease NS3/4A cleaves mitochondrial antiviral signaling protein off the mitochondria to evade innate immunity. Proc. Natl. Acad. Sci. U.S.A. 102, 17717–17722. doi: 10.1073/pnas.0508531102
Li, T., Li, X., Attri, K. S., Liu, C., Li, L., Herring, L. E., et al. (2018). O-GlcNAc transferase links glucose metabolism to MAVS-mediated antiviral innate immunity. Cell Host Microbe 24, 791.e6–803.e6. doi: 10.1016/j.chom.2018.11.001
Lim, Y. J., Koo, J. E., Hong, E.-H., Park, Z.-Y., Lim, K.-M., Bae, O.-N., et al. (2015). A Src-family-tyrosine kinase, Lyn, is required for efficient IFN-β expression in pattern recognition receptor, RIG-I, signal pathway by interacting with IPS-1. Cytokine 72, 63–70. doi: 10.1016/j.cyto.2014.12.008
Lin, W., Zhang, J., Lin, H., Li, Z., Sun, X., Xin, D., et al. (2016). Syndecan-4 negatively regulates antiviral signalling by mediating RIG-I deubiquitination via CYLD. Nat. Commun. 7:11848. doi: 10.1038/ncomms11848
Liu, B., Zhang, M., Chu, H., Zhang, H., Wu, H., Song, G., et al. (2017). The ubiquitin E3 ligase TRIM31 promotes aggregation and activation of the signaling adaptor MAVS through Lys63-linked polyubiquitination. Nat. Immunol. 18, 214–224. doi: 10.1038/ni.3641
Liu, C., Huang, S., Wang, X., Wen, M., Zheng, J., Wang, W., et al. (2019). The otubain YOD1 suppresses aggregation and activation of the signaling adaptor MAVS through Lys63-linked deubiquitination. J. Immunol. 202, 2957–2970. doi: 10.4049/jimmunol.1800656
Liu, H. M., Jiang, F., Loo, Y. M., Hsu, S., Hsiang, T.-Y., Marcotrigiano, J., et al. (2016). Regulation of retinoic acid inducible gene-I (RIG-I) activation by the histone deacetylase 6. EBioMedicine 9, 195–206. doi: 10.1016/j.ebiom.2016.06.015
Liu, H. M., Loo, Y.-M., Horner, S. M., Zornetzer, G. A., Katze, M. G., and Gale, M. (2012). The mitochondrial targeting chaperone 14-3-3ε regulates a RIG-I translocon that mediates membrane association and innate antiviral immunity. Cell Host Microbe 11, 528–537. doi: 10.1016/j.chom.2012.04.006
Liu, S., Cai, X., Wu, J., Cong, Q., Chen, X., Li, T., et al. (2015). Phosphorylation of innate immune adaptor proteins MAVS, STING, and TRIF induces IRF3 activation. Science 347:aaa2630. doi: 10.1126/science.aaa2630
Liu, S., Chen, J., Cai, X., Wu, J., Chen, X., Wu, Y.-T., et al. (2013). MAVS recruits multiple ubiquitin E3 ligases to activate antiviral signaling cascades. Elife 2:e00785. doi: 10.7554/eLife.00785
Liu, X.-Y., Chen, W., Wei, B., Shan, Y.-F., and Wang, C. (2011). IFN-induced TPR protein IFIT3 potentiates antiviral signaling by bridging MAVS and TBK1. J. Immunol. 187, 2559–2568. doi: 10.4049/jimmunol.1100963
Liu, X.-Y., Wei, B., Shi, H.-X., Shan, Y.-F., and Wang, C. (2010). Tom70 mediates activation of interferon regulatory factor 3 on mitochondria. Cell Res. 20, 994–1011. doi: 10.1038/cr.2010.103
Liuyu, T., Yu, K., Ye, L., Zhang, Z., Zhang, M., Ren, Y., et al. (2019). Induction of OTUD4 by viral infection promotes antiviral responses through deubiquitinating and stabilizing MAVS. Cell Res. 29, 67–79. doi: 10.1038/s41422-018-0107-6
Ma, J., Ketkar, H., Geng, T., Lo, E., Wang, L., Xi, J., et al. (2018). Zika virus non-structural protein 4A blocks the RLR-MAVS Signaling. Front. Microbiol. 9:1350. doi: 10.3389/fmicb.2018.01350
Maharaj, N. P., Wies, E., Stoll, A., and Gack, M. U. (2012). Conventional protein kinase C- (PKC-) and PKC- negatively regulate RIG-I antiviral signal transduction. J. Virol. 86, 1358–1371. doi: 10.1128/jvi.06543-11
Mehta, V., and Trinkle-Mulcahy, L. (2016). Recent advances in large-scale protein interactome mapping. F1000Res. 5:F1000FacultyRev–782. doi: 10.12688/F1000RESEARCH.7629.1
Meng, J., Yao, Z., He, Y., Zhang, R., Zhang, Y., Yao, X., et al. (2017). ARRDC4 regulates enterovirus 71-induced innate immune response by promoting K63 polyubiquitination of MDA5 through TRIM65. Cell Death Dis. 8:e2866. doi: 10.1038/cddis.2017.257
Miyashita, M., Oshiumi, H., Matsumoto, M., and Seya, T. (2011). DDX60, a DEXD/H Box helicase, is a novel antiviral factor promoting RIG-I-Like receptor-mediated signaling. Mol. Cell. Biol. 31, 3802–3819. doi: 10.1128/mcb.01368-10
Mohanty, A., Tiwari-Pandey, R., and Pandey, N. R. (2019). Mitochondria: the indispensable players in innate immunity and guardians of the inflammatory response. J. Cell Commun. Signal. 13, 303–318. doi: 10.1007/s12079-019-00507-9
Motwani, M., Pesiridis, S., and Fitzgerald, K. A. (2019). DNA sensing by the cGAS–STING pathway in health and disease. Nat. Rev. Genet. 20, 657–674. doi: 10.1038/s41576-019-0151-1
Mukherjee, A., Morosky, S. A., Delorme-Axford, E., Dybdahl-Sissoko, N., Oberste, M. S., Wang, T., et al. (2011). The coxsackievirus B 3C protease cleaves MAVS and TRIF to attenuate host type I interferon and apoptotic signaling. PLoS Pathog. 7:e1001311. doi: 10.1371/journal.ppat.1001311
Narayan, K., Waggoner, L., Pham, S. T., Hendricks, G. L., Waggoner, S. N., Conlon, J., et al. (2014). TRIM13 Is a negative regulator of MDA5-mediated type I interferon production. J. Virol. 88, 10748–10757. doi: 10.1128/jvi.02593-13
Nistal-Villán, E., Gack, M. U., Martínez-Delgado, G., Maharaj, N. P., Inn, K.-S., Yang, H., et al. (2010). Negative role of RIG-I serine 8 phosphorylation in the regulation of interferon-beta production. J. Biol. Chem. 285, 20252–20261. doi: 10.1074/jbc.M109.089912
Odendall, C., Dixit, E., Stavru, F., Bierne, H., Franz, K. M., Durbin, A. F., et al. (2014). Diverse intracellular pathogens activate type III interferon expression from peroxisomes. Nat. Immunol. 15, 717–726. doi: 10.1038/ni.2915
Okamoto, M., Kouwaki, T., Fukushima, Y., and Oshiumi, H. (2017). Regulation of RIG-I activation by K63-Linked polyubiquitination. Front. Immunol. 8:1942. doi: 10.3389/fimmu.2017.01942
Onoguchi, K., Onomoto, K., Takamatsu, S., Jogi, M., Takemura, A., Morimoto, S., et al. (2010). Virus-infection or 5′ppp-RNA activates antiviral signal through redistribution of IPS-1 mediated by MFN1. PLoS Pathog. 6:e1001012. doi: 10.1371/journal.ppat.1001012
Oshiumi, H., Kouwaki, T., and Seya, T. (2016). Accessory factors of cytoplasmic viral RNA sensors required for antiviral innate immune response. Front. Immunol. 7:200. doi: 10.3389/fimmu.2016.00200
Oshiumi, H., Matsumoto, M., Hatakeyama, S., and Seya, T. (2009). Riplet/RNF135, a RING finger protein, ubiquitinates RIG-I to promote interferon-beta induction during the early phase of viral infection. J. Biol. Chem. 284, 807–817. doi: 10.1074/jbc.M804259200
Pan, Y., Li, R., Meng, J.-L., Mao, H.-T., Zhang, Y., and Zhang, J. (2014). Smurf2 negatively modulates RIG-I-dependent antiviral response by targeting VISA/MAVS for ubiquitination and degradation. J. Immunol. 192, 4758–4764. doi: 10.4049/jimmunol.1302632
Parisien, J.-P., Lenoir, J. J., Mandhana, R., Rodriguez, K. R., Qian, K., Bruns, A. M., et al. (2018). RNA sensor LGP2 inhibits TRAF ubiquitin ligase to negatively regulate innate immune signaling. EMBO Rep. 19:e45176. doi: 10.15252/embr.201745176
Qi, N., Shi, Y., Zhang, R., Zhu, W., Yuan, B., Li, X., et al. (2017). Multiple truncated isoforms of MAVS prevent its spontaneous aggregation in antiviral innate immune signalling. Nat. Commun. 8:15676. doi: 10.1038/ncomms15676
Qin, Y., Xue, B., Liu, C., Wang, X., Tian, R., Xie, Q., et al. (2017). NLRX1 Mediates MAVS degradation to attenuate the hepatitis C virus-induced innate immune response through PCBP2. J. Virol. 91:e01264-17. doi: 10.1128/jvi.01264-17
Rebsamen, M., Vazquez, J., Tardivel, A., Guarda, G., Curran, J., and Tschopp, J. (2011). NLRX1/NOD5 deficiency does not affect MAVS signalling. Cell Death Differ. 18:1387. doi: 10.1038/cdd.2011.64
Refolo, G., Ciccosanti, F., Di Rienzo, M., Basulto Perdomo, A., Romani, M., Alonzi, T., et al. (2019). Negative regulation of mitochondrial antiviral signaling protein-mediated antiviral signaling by the mitochondrial protein LRPPRC during hepatitis C virus infection. Hepatology 69, 34–50. doi: 10.1002/hep.30149
Riedl, W., Acharya, D., Lee, J.-H., Liu, G., Serman, T., Chiang, C., et al. (2019). Zika virus NS3 mimics a cellular 14-3-3-binding motif to antagonize RIG-I- and MDA5-mediated innate immunity. Cell Host Microbe 26, 493.e6–503.e6. doi: 10.1016/J.CHOM.2019.09.012
Satoh, T., Kato, H., Kumagai, Y., Yoneyama, M., Sato, S., Matsushita, K., et al. (2010). LGP2 is a positive regulator of RIG-I- and MDA5-mediated antiviral responses. Proc. Natl. Acad. Sci. U.S.A. 107, 1512–1517. doi: 10.1073/pnas.0912986107
Seth, R. B., Sun, L., Ea, C.-K., and Chen, Z. J. (2005). Identification and characterization of MAVS, a mitochondrial antiviral signaling protein that activates NF-kappaB and IRF 3. Cell 122, 669–682. doi: 10.1016/j.cell.2005.08.012
Sharma, D., and Kanneganti, T. D. (2016). The cell biology of inflammasomes: mechanisms of inflammasome activation and regulation. J. Cell Biol. 213, 617–629. doi: 10.1083/jcb.201602089
Shi, Y., Yuan, Zhu, Zhang, Li, Hao, et al. (2017). Ube2D3 and Ube2N are essential for RIG-I-mediated MAVS aggregation in antiviral innate immunity. Nat. Commun. 8:15138. doi: 10.1038/ncomms15138
Soares, F., Tattoli, I., Wortzman, M. E., Arnoult, D., Philpott, D. J., and Girardin, S. E. (2013). NLRX1 does not inhibit MAVS-dependent antiviral signalling. Innate Immun. 19, 438–448. doi: 10.1177/1753425912467383
Song, T., Wei, C., Zheng, Z., Xu, Y., Cheng, X., Yuan, Y., et al. (2010). c-Abl tyrosine kinase interacts with MAVS and regulates innate immune response. FEBS Lett. 584, 33–38. doi: 10.1016/j.febslet.2009.11.025
Song, N., Qi, Q., Cao, R., Qin, B., Wang, B., Wang, Y., et al. (2019). MAVS O-GlcNAcylation is essential for host antiviral immunity against lethal RNA viruses. Cell Rep. 28, 2386.e5–2396.e5. doi: 10.1016/j.celrep.2019.07.085
Soonthornvacharin, S., Rodriguez-Frandsen, A., Zhou, Y., Galvez, F., Huffmaster, N. J., Tripathi, S., et al. (2017). Systems-based analysis of RIG-I-dependent signalling identifies KHSRP as an inhibitor of RIG-I receptor activation. Nat. Microbiol. 2:17022. doi: 10.1038/nmicrobiol.2017.22
Streicher, F., and Jouvenet, N. (2019). Stimulation of innate immunity by host and viral RNAs. Trends Immunol. 40, 1134–1148. doi: 10.1016/j.it.2019.10.009
Subramanian, N., Natarajan, K., Clatworthy, M. R., Wang, Z., and Germain, R. N. (2013). The adaptor MAVS promotes NLRP3 mitochondrial localization and inflammasome activation. Cell 153, 348–361. doi: 10.1016/j.cell.2013.02.054
Sun, X., Sun, L., Zhao, Y., Li, Y., Lin, W., Chen, D., et al. (2016). MAVS maintains mitochondrial homeostasis via autophagy. Cell Discov. 2:16024. doi: 10.1038/celldisc.2016.24
Sun, Z., Ren, H., Liu, Y., Teeling, J. L., and Gu, J. (2011). Phosphorylation of RIG-I by casein kinase II inhibits its antiviral response. J. Virol. 85, 1036–1047. doi: 10.1128/jvi.01734-10
Swanson, K. V., Deng, M., and Ting, J. P. Y. (2019). The NLRP3 inflammasome: molecular activation and regulation to therapeutics. Nat. Rev. Immunol. 19, 477–489. doi: 10.1038/s41577-019-0165-0
Tait Wojno, E. D., Hunter, C. A., and Stumhofer, J. S. (2019). The immunobiology of the interleukin-12 family: room for discovery. Immunity 50, 851–870. doi: 10.1016/j.immuni.2019.03.011
Takashima, K., Oshiumi, H., Takaki, H., Matsumoto, M., and Seya, T. (2015). RIOK3-mediated phosphorylation of MDA5 interferes with its assembly and attenuates the innate immune response. Cell Rep. 11, 192–200. doi: 10.1016/j.celrep.2015.03.027
Tal, M. C., and Iwasaki, A. (2009). Autophagic control of RLR signaling. Autophagy 5, 749–750. doi: 10.4161/auto.5.5.8789
Tan, P., He, L., Cui, J., Qian, C., Cao, X., Lin, M., et al. (2017). Assembly of the WHIP-TRIM14-PPP6C mitochondrial complex promotes RIG-I-mediated antiviral signaling. Mol. Cell 68, 293.e5–307.e5. doi: 10.1016/j.molcel.2017.09.035
Tan, X., Sun, L., Chen, J., and Chen, Z. J. (2018). Detection of microbial infections through innate immune sensing of nucleic acids. Annu. Rev. Microbiol. 72, 447–478. doi: 10.1146/annurev-micro-102215-095605
Tang, E. D., and Wang, C.-Y. (2009). MAVS self-association mediates antiviral innate immune signaling. J. Virol. 83, 3420–3428. doi: 10.1128/jvi.02623
Varga, Z. T., Grant, A., Manicassamy, B., and Palese, P. (2012). Influenza virus protein PB1-F2 Inhibits the induction of Type I interferon by binding to MAVS and decreasing mitochondrial membrane potential. J. Virol. 86, 8359–8366. doi: 10.1128/jvi.01122-12
Vazquez, C., and Horner, S. M. (2015). MAVS coordination of antiviral innate immunity. J. Virol. 89, 6974–6977. doi: 10.1128/JVI.01918-14
Venkataraman, T., Valdes, M., Elsby, R., Kakuta, S., Caceres, G., Saijo, S., et al. (2007). Loss of DExD/H box RNA helicase LGP2 manifests disparate antiviral responses. J. Immunol. 178, 6444–6455. doi: 10.4049/jimmunol.178.10.6444
Vitour, D., Dabo, S., Ahmadi Pour, M., Vilasco, M., Vidalain, P.-O., Jacob, Y., et al. (2009). Polo-like kinase 1 (PLK1) regulates interferon (IFN) induction by MAVS. J. Biol. Chem. 284, 21797–21809. doi: 10.1074/jbc.M109.018275
Wagner, S. A., Beli, P., Weinert, B. T., Schölz, C., Kelstrup, C. D., Young, C., et al. (2012). Proteomic analyses reveal divergent ubiquitylation site patterns in murine tissues. Mol. Cell. Proteomics 11, 1578–1585. doi: 10.1074/mcp.M112.017905
Wang, B., Xi, X., Lei, X., Zhang, X., Cui, S., Wang, J., et al. (2013). Enterovirus 71 protease 2A pro targets MAVS to inhibit anti-viral type I interferon responses. PLoS Pathog. 9:e1006243. doi: 10.1371/journal.ppat.1003231
Wang, L., Zhao, W., Zhang, M., Wang, P., Zhao, K., Zhao, X., et al. (2013). USP4 positively regulates RIG-I-mediated antiviral response through deubiquitination and stabilization of RIG-I. J. Virol. 87, 4507–4515. doi: 10.1128/jvi.00031-13
Wang, P., Yang, L., Cheng, G., Yang, G., Xu, Z., You, F., et al. (2013). UBXN1 interferes with Rig-I-like receptor-mediated antiviral immune response by targeting MAVS. Cell Rep. 3, 1057–1070. doi: 10.1016/j.celrep.2013.02.027
Wang, W., Jiang, M., Liu, S., Zhang, S., Liu, W., Ma, Y., et al. (2016). RNF122 suppresses antiviral type I interferon production by targeting RIG-I CARDs to mediate RIG-I degradation. Proc. Natl. Acad. Sci. U.S.A. 113, 9581–9586. doi: 10.1073/pnas.1604277113
Wang, Y., Tong, X., and Ye, X. (2012). Ndfip1 negatively regulates RIG-I-dependent immune signaling by enhancing E3 ligase Smurf1-mediated MAVS degradation. J. Immunol. 189, 5304–5313. doi: 10.4049/jimmunol.1201445
West, A. P., Brodsky, I. E., Rahner, C., Woo, D. K., Erdjument-Bromage, H., Tempst, P., et al. (2011). TLR signalling augments macrophage bactericidal activity through mitochondrial ROS. Nature 472, 476–480. doi: 10.1038/nature09973
Wi, S. M., Moon, G., Kim, J., Kim, S. T., Shim, J. H., Chun, E., et al. (2014). TAK1-ECSIT-TRAF6 complex plays a key role in the TLR4 signal to activate NF-κB. J. Biol. Chem. 289, 35205–35214. doi: 10.1074/jbc.M114.597187
Wies, E., Wang, M. K., Maharaj, N. P., Chen, K., Zhou, S., Finberg, R. W., et al. (2013). Dephosphorylation of the RNA sensors RIG-I and MDA5 by the phosphatase PP1 is essential for innate immune signaling. Immunity 38, 437–449. doi: 10.1016/j.immuni.2012.11.018
Willemsen, J., Wicht, O., Wolanski, J. C., Baur, N., Bastian, S., Haas, D. A., et al. (2017). Phosphorylation-dependent feedback inhibition of RIG-I by dapk1 identified by kinome-wide siRNA Screening. Mol. Cell 65, 403.e8–415.e8. doi: 10.1016/j.molcel.2016.12.021
Wu, J., Sun, L., Chen, X., Du, F., Shi, H., Chen, C., et al. (2013). Cyclic GMP-AMP is an endogenous second messenger in innate immune signaling by cytosolic DNA. Science 339, 826–830. doi: 10.1126/science.1229963
Xian, H., Yang, S., Jin, S., Zhang, Y., and Cui, J. (2019). LRRC59 modulates type I interferon signaling by restraining the SQSTM1/p62-mediated autophagic degradation of pattern recognition receptor DDX58/RIG-I. Autophagy 16, 408–418. doi: 10.1080/15548627.2019.1615303
Xiang, W., Zhang, Q., Lin, X., Wu, S., Zhou, Y., Meng, F., et al. (2016). PPM1A silences cytosolic RNA sensing and antiviral defense through direct dephosphorylation of MAVS and TBK1. Sci. Adv. 2:e1501889. doi: 10.1126/sciadv.1501889
Xing, J., Zhang, A., Minze, L. J., Li, X. C., and Zhang, Z. (2018). TRIM29 Negatively Regulates the Type I IFN Production in Response to RNA Virus. J. Immunol. 201, 183–192. doi: 10.4049/jimmunol.1701569
Xu, L., Xiao, N., Liu, F., Ren, H., and Gu, J. (2009). Inhibition of RIG-I and MDA5-dependent antiviral response by gC1qR at mitochondria. Proc. Natl. Acad. Sci. U.S.A. 106, 1530–1535. doi: 10.1073/pnas.0811029106
Xue, B., Li, H., Guo, M., Wang, J., Xu, Y., Zou, X., et al. (2018). TRIM21 Promotes Innate Immune Response to RNA Viral Infection through Lys27-Linked Polyubiquitination of MAVS. J. Virol. 92:e00321-18. doi: 10.1128/jvi.00321-18
Yang, Y., Liang, Y., Qu, L., Chen, Z., Yi, M., Li, K., et al. (2007). Disruption of innate immunity due to mitochondrial targeting of a picornaviral protease precursor. Proc. Natl. Acad. Sci. U.S.A. 104, 7253–7258. doi: 10.1073/pnas.0611506104
Yasukawa, K., Oshiumi, H., Takeda, M., Ishihara, N., Yanagi, Y., Seya, T., et al. (2009). Mitofusin 2 inhibits mitochondrial antiviral signaling. Sci. Signal. 2:ra47. doi: 10.1126/scisignal.2000287
Yates, J. R. (2019). Recent technical advances in proteomics. F1000Res. 8:F1000FacultyRev–351. doi: 10.12688/f1000research.16987.1
Yoneyama, M., Kikuchi, M., Matsumoto, K., Imaizumi, T., Miyagishi, M., Taira, K., et al. (2005). Shared and unique functions of the DExD/H-box helicases RIG-I. MDA5, and LGP2 in Antiviral Innate Immunity. J. Immunol. 175, 2851–2858. doi: 10.4049/jimmunol.175.5.2851
Yoo, Y.-S., Park, Y.-Y., Kim, J.-H., Cho, H., Kim, S.-H., Lee, H.-S., et al. (2015). The mitochondrial ubiquitin ligase MARCH5 resolves MAVS aggregates during antiviral signalling. Nat. Commun. 6:7910. doi: 10.1038/ncomms8910
Yoshinaka, T., Kosako, H., Yoshizumi, T., Furukawa, R., Hirano, Y., Kuge, O., et al. (2019). Structural basis of mitochondrial scaffolds by prohibitin complexes: insight into a role of the coiled-coil region. iScience 19, 1065–1078. doi: 10.1016/j.isci.2019.08.056
Yoshizumi, T., Imamura, H., Taku, T., Kuroki, T., Kawaguchi, A., Ishikawa, K., et al. (2017). RLR-mediated antiviral innate immunity requires oxidative phosphorylation activity. Sci. Rep. 7:5379
You, F., Sun, H., Zhou, X., Sun, W., Liang, S., Zhai, Z., et al. (2009). PCBP2 mediates degradation of the adaptor MAVS via the HECT ubiquitin ligase AIP4. Nat. Immunol. 10, 1300–1308. doi: 10.1038/ni.1815
Zevini, A., Olagnier, D., and Hiscott, J. (2017). Crosstalk between Cytoplasmic RIG-I and STING Sensing Pathways. Trends Immunol. 38, 194–205. doi: 10.1016/j.it.2016.12.004
Zhang, W., Wang, G., Xu, Z.-G., Tu, H., Hu, F., Dai, J., et al. (2019). lactate is a natural suppressor of RLR signaling by targeting MAVS. Cell 178, 176.e15–189.e15. doi: 10.1016/j.cell.2019.05.003
Zhao, C., Jia, M., Song, H., Yu, Z., Wang, W., Li, Q., et al. (2017). The E3 ubiquitin ligase TRIM40 attenuates antiviral immune responses by targeting MDA5 and RIG-I. Cell Rep. 21, 1613–1623. doi: 10.1016/j.celrep.2017.10.020
Zhao, K., Zhang, Q., Li, X., Zhao, D., Liu, Y., Shen, Q., et al. (2016). Cytoplasmic STAT4 promotes antiviral Type I IFN production by blocking CHIP-mediated degradation of RIG-I. J. Immunol. 196, 1209–1217. doi: 10.4049/jimmunol.1501224
Zhao, Y., Sun, X., Nie, X., Sun, L., Tang, T.-S., Chen, D., et al. (2012). COX5B regulates MAVS-mediated antiviral signaling through interaction with ATG5 and repressing ROS production. PLoS Pathog. 8:e1003086. doi: 10.1371/journal.ppat.1003086
Zhong, B., Zhang, Y., Tan, B., Liu, T.-T., Wang, Y.-Y., and Shu, H.-B. (2010). The E3 ubiquitin ligase RNF5 targets virus-induced signaling adaptor for ubiquitination and degradation. J. Immunol. 184, 6249–6255. doi: 10.4049/jimmunol.0903748
Zhou, P., Ding, X., Wan, X., Liu, L., Yuan, X., Zhang, W., et al. (2018). MLL5 suppresses antiviral innate immune response by facilitating STUB1-mediated RIG-I degradation. Nat. Commun. 9:1243. doi: 10.1038/s41467-018-03563-8
Keywords: mitochondrial antiviral signaling protein, retinoic acid-inducible gene I, proteomics, mitochondria, RNA virus infection
Citation: Refolo G, Vescovo T, Piacentini M, Fimia GM and Ciccosanti F (2020) Mitochondrial Interactome: A Focus on Antiviral Signaling Pathways. Front. Cell Dev. Biol. 8:8. doi: 10.3389/fcell.2020.00008
Received: 20 November 2019; Accepted: 10 January 2020;
Published: 14 February 2020.
Edited by:
Luisa Pieroni, Santa Lucia Foundation (IRCCS), ItalyCopyright © 2020 Refolo, Vescovo, Piacentini, Fimia and Ciccosanti. This is an open-access article distributed under the terms of the Creative Commons Attribution License (CC BY). The use, distribution or reproduction in other forums is permitted, provided the original author(s) and the copyright owner(s) are credited and that the original publication in this journal is cited, in accordance with accepted academic practice. No use, distribution or reproduction is permitted which does not comply with these terms.
*Correspondence: Gian Maria Fimia, Z2lhbm1hcmlhLmZpbWlhQGlubWkuaXQ=