- 1Department of Psychiatry, Harvard Medical School, Boston, MA, United States
- 2Angiogenesis and Brain Development Laboratory, Division of Basic Neuroscience, McLean Hospital, Belmont, MA, United States
- 3Department of Pharmacology, The University of Illinois at Chicago, Chicago, IL, United States
Exosomes have been described as nanoscale membranous extracellular vesicles that emerge from a variety of cells and tissues and are enriched with biologically active genomic and non-genomic biomolecules capable of transducing cell to cell communication. Exosome release, and exosome mediated signaling and cross-talks have been reported in several pathophysiological states. Therefore, exosomes have the potential to become suitable for the diagnosis, prognosis and treatment of specific diseases, including endothelial cell (EC) dysfunction and regeneration. The role of EC-derived exosomes in the mechanisms of cardiovascular tissue regenerative processes represents currently an area of intense research activity. Recent studies have described the potential of exosomes to influence the pathophysiology of immune signaling, tumor metastasis, and angiogenesis. In this review, we briefly discuss progress made in our understanding of the composition and the roles of exosomes in relation to EC regeneration as well as revascularization of ischemic tissues.
Introduction
Exosomes are nanometer (30–100 nm) sized membranous vesicles originating during the formation of multivesicular bodies (MVBs) (Dignat-George and Boulanger, 2011; Ribeiro et al., 2013; Boulanger et al., 2017; Théry et al., 2018). Biochemically, exosomes are characterized by the presence of CD63, CD14, TSG101, heat shock protein and flotillin; these exosomes can be sedimented at 120,000 × g (Théry et al., 2006; Vlassov et al., 2012; Kowal et al., 2016). Exosomes and apoptotic bodies are broadly classified as extracellular vesicles; in contrast to exosomes, apoptotic bodies are larger (500–5000 nm) (Caruso and Poon, 2018), and they sediment at 1,200–10,000 × g (Théry et al., 2006). Exosomal biogenesis occurs in the endosomal pathway and is characterized by biochemical properties (Table 1), whereas apoptotic bodies are characterized by membrane blebbing and their unique cell surface markers, e.g., phosphatidylserine and Annexin-V (Henson et al., 2001). Exosomes are usually released into the extracellular space when MVBs fuse with the plasma membrane, and exosomes can transport lipids, mRNAs and proteins that can alter cellular behavior in a paracrine or autocrine manner (Sahoo and Losordo, 2014; Kishore and Khan, 2016). Depending on the tissue microenvironment, and the exosome content, these vesicles mediate an array of cellular functions (Ribeiro et al., 2013). A classic example of an altered tissue microenvironment is the maintenance and repair of tissues in response to injury. Studies are beginning to document cell-cell signaling events that mediate restorative functions in the tissue microenvironment. In this context, the mechanisms of exosome uptake by target cells might be mediated by more than one mechanism. In cultured cells, exosome uptake can occur through: (a) clathrin-dependent endocytosis, (b) caveolae-dependent endocytosis, (c) phagocytosis, and (d) micropinocytosis (McKelvey et al., 2015). Whether exosome uptake by target cells is a physiologically regulated process remains incompletely understood.
Tissue repair mechanisms entail effective endothelial cell (EC) regeneration and reestablishment of blood flow in damaged and ischemic tissues. To accomplish this repair process, ECs that form the innermost linings of the blood vessels undergo regeneration and angiogenesis to support the restoration of tissue homeostasis (Carmeliet, 2005; Liu et al., 2019; Miyagawa et al., 2019; Williams and Wu, 2019). EC regeneration is a complex biological process that include EC migration, EC survival, rapid proliferation, tube formation, and ultimately reperfusion of injured tissues to restore homeostasis of the tissue microenvironment (Carmeliet, 2005; Bentley and Chakravartula, 2017; McDonald et al., 2018; Liu et al., 2019; Williams and Wu, 2019). Although several studies have attempted to understand the process of EC or vascular regeneration, the molecular mechanism that drives this process remains incompletely understood. Given the biological properties of exosomes and the events that they can regulate, the idea that exosomes derived from various cell types, including ECs themselves in the damaged tissue niche, can modulate EC regeneration remains an active area of research (Ibrahim et al., 2014; Li et al., 2016; Abid Hussein et al., 2017; Balbi et al., 2017; Adamiak and Sahoo, 2018; Dougherty et al., 2018; Ju et al., 2018; Bian et al., 2019; Cheng et al., 2019). In support of this notion, we describe a compendium of studies conducted over the past decade that highlight both EC- and non-EC derived exosomal molecular cargoes which drive this regenerative process. The idea and the discussions that exosomes might provide therapeutic benefit in the settings of ischemic cardiovascular diseases involving physiological injuries that might otherwise transition to disease states, should be rewarding efforts.
EC Regeneration Likely Involves More Than One Mechanism
Broadly, there are at least three major types of ECs in mammalian systems, related to arterial, venous, and lymphatic vessels (Coultas et al., 2005; Adams and Alitalo, 2007; Park et al., 2013; Qiu and Hirschi, 2019). These mature ECs are known to be arrested at the G0-phase of the cell cycle, and they have a limited turn-over rate, cycling once every 3–5 years in vivo. Thus, ECs are considered terminally differentiated cells. In principle, ECs could regenerate from adult EC stem cells; however, there is conflicting evidence regarding whether adult EC-stem cells actually exist in vivo. Many studies suggest the existence of adult hemangioblast and or angioblast, on the basis of CD34 and Flk1 expression (and other stem cell markers, e.g., Brachyury and Er71/Etv2), and the ability of these cells to form tube-like structures (Asahara et al., 1997; Loges et al., 2004; Hirschi, 2012). On the contrary, others have argued that these cells are likely to be present in low numbers in adults in vivo (Rafii, 2000; Park et al., 2013; Yoder, 2018; Qiu and Hirschi, 2019). Critiques have noted that bone marrow-derived monocytes and macrophages might have been misidentified as endothelial progenitor cells, thus confusing even the experts (Medina et al., 2017). However, genetic lineage tracing experiments in mice remain inconclusive regarding the presence of EC-stem cells. Recent article summarized the proangiogenic benefit observed in preclinical and clinical studies from over 700 patients in clinical trials of CD34 + cell therapy (Sietsema et al., 2019). Nevertheless, developmental studies suggest that venous ECs can be derived from arterial ECs, whereas lymphatic ECs can be derived from venous ECs (Wang et al., 1998; Yang et al., 2012). However, depending on the type of injury or damage experienced by the ECs, more than one mechanism is likely to activate EC regeneration. Our own studies have suggested that ECs become proliferative after experimental ischemia or myocardial infarction (Kohler et al., 2014; Baruah et al., 2017). Another mechanism is dedifferentiation followed by redifferentiation of ECs in the aftermath of ischemia, a process that can also be activated by administration of low-dose small molecule inhibitors of GSK-3b called BIO (6-bromoindirubin-3-oxime) and tideglusib/NP12 (Kohler et al., 2014; Baruah et al., 2017). Yet another mechanism might be the endothelial to mesenchymal transition (EndoMT) (Dejana and Lampugnani, 2018), a biological process that occurs during the formation of cardiac valves and contributes to the emergence of several other cell lineages (Monaghan et al., 2016), and is also a response to ischemia (Manavski et al., 2018).
Thus, it is reasonable to hypothesize that exosome-mediated regeneration of ECs is likely to include at least three distinct mechanisms, but not limited to:
• Exosomes that induce EC proliferation and survival, e.g., vasculogenesis and angiogenesis.
• Exosomes that induces EC dedifferentiation/redifferentiation (not a proven mechanism): for example, exosomes that upregulate Cyclin-D1 and down-regulate p53, p21, and p27 mRNAs should induce EC-dedifferentiation and rapid cell cycle progression.
• Exosomes that mediate EndoMT (not a proven mechanism); in principle, exosomes containing microRNAs (miRNAs) that downregulate VE-cadherin and up-regulate Twist, Slug and Snail, and matrix metalloproteases (MMPs) could mediate EndoMT.
Thus, genomic and non-genomic cargoes in exosomes that are capable of inducing signaling to one of the above events should provide EC regenerative benefit. In addition, regeneration of ECs might be possible via exosomes that mediate transdifferentiation of somatic cells or by directly reprogramming somatic cells into ECs.
In support of this idea, a few groups have addressed the possibility of using exosome mediated reprogramming of ECs for vascular regeneration (Cheng et al., 2017; Lee et al., 2017). For example, exosomes secreted by tumor cells carry a number of potent pro-angiogenic factors such as VEGF, TGFβ, bFGF, MMP2, and MMP9, mediated angiogenic activities of ECs (Skog et al., 2008; Giusti et al., 2016; Ludwig and Whiteside, 2018). This idea is currently being explored further in several laboratories in the settings of cardiovascular regeneration and rejuvenation. However, it remains to be seen if the exosome(s) mediated reprogrammed ECs have the ability to repair effectively and reestablish blood supply productively, in aftermath of ischemic episodes.
Exosomes With Non-Genomic Cargoes That Mediate EC Regeneration
Myocardial infarction represents a major cause of death among all cardiovascular diseases. Injured cardiac tissues due to myocardial infarction or ischemic insult trigger a series of adaptive response, to initiate and drive repair the injured heart. Therefore, it was surmised that in the aftermath of myocardial infarction the injured myocardium might release extracellular vesicles and exosomes that could induce a regenerative program. Cardiac extracellular vesicles or exosomes are now known to be present in both normal and infarcted heart (Chistiakov et al., 2016). Therefore, these exosomes that are secreted in an infarcted heart mediate various cell to cell communication events, including exosome biogenesis which provide cardiovascular regenerative benefits, improved cardiac function, and normalize tissue homeostasis (Barile et al., 2012; Waldenström et al., 2012; Wang et al., 2016). In a study, human pediatric cardiac progenitor cell (CPCs) prepared from the right atrial appendages from children of different ages undergoing cardiac surgery for congenital heart defects were isolated and cultured under hypoxic or normoxic conditions. In their, study, the authors found that CPC exosomes derived from neonates improved cardiac function, mediated angiogenesis, and reduced fibrosis, independent of culture oxygen levels (Agarwal et al., 2017). However, there are many open questions that need to be addressed (Bollini et al., 2018). A detailed overview of exosomes and their regenerative potential in infarcted heart can be found elsewhere (Bollini et al., 2018; Shanmuganathan et al., 2018).
At the cellular level, EC proliferation and survival represent two key events in the process of EC regeneration (Park et al., 2013; Qiu and Hirschi, 2019). These cells must proliferate rapidly and survive to make up for the loss of cells or to replace damaged and non-functional cells. Inadequate proliferation or enhanced cellular death might initiate or augment pathological event. Therefore, well-coordinated cellular proliferation and survival events are quintessential to normalizing damaged tissues (McDonald et al., 2018).
A study on exosome cargo and EC interaction has been conducted by Nazarenko et al. (2010) in a tumor microenvironment. This study has described a role of Tetraspanin (Tspan8) containing exosomes, which efficiently induce angiogenesis in tumors and tumor-free tissues. The authors have found that Tspan8 contributes to selective recruitment of proteins and mRNAs into exosomes; these markers include CD106 and CD49d, which have been implicated in exosome-EC binding and EC internalization. Exosome uptake induces vascular endothelial growth factor (VEGF)–independent regulation of several angiogenesis-related genes, including von Willebrand factor, Tspan8, the chemokines CXCL5, and MIF, the chemokine receptor CCR1 and, together with VEGF, VEGF receptor 2 (Nazarenko et al., 2010). EC uptake of Tspan8-CD49d complex–containing exosomes is accompanied by enhanced angiogenic activities of EC, such as proliferation, migration, and sprouting. Several studies subsequently exploited the potential of exosome cargoes in a tumor-free environment. Accordingly, one elegant investigation by Sahoo et al. (2011) has shown that exosomes derived from human CD34+ stem cells mediate EC proliferation and survival, thereby stimulating the angiogenic activities of ECs. As expected, exosomes purified from human induced pluripotent stem cells have been found to induce angiogenesis and improve recovery in a mouse model of hind limb ischemia (Hu et al., 2015). Bian et al. (2013) have examined the effects of mesenchymal stem cell (MSC) derived extracellular vesicles which also included exosomes, and found that exosomes mediated efficient regeneration of ECs in a rat model of acute myocardial infarction. Although this study did not conclusively identify the types of molecules involved in this process, it highlighted the potential role of exosomes in mediating angiogenic processes in an injured tissue microenvironment (Shabbir et al., 2015; Teng et al., 2015). The Wnt/b-catenin signaling pathway is crucial in regulating both developmental and therapeutic angiogenesis (Dejana and Kühl, 2010). Interestingly, MSC exosomes express Wnt4, which induces translocation of (β-catenin into the nuclei of recipient ECs, thereby promoting angiogenic events in a rat skin burn model (Zhang et al., 2015). Similar studies have demonstrated that the Sonic hedgehog signaling pathway, the presence of platelet derived growth factor receptor in the extracellular vesicles or PKA signaling might contribute to the proangiogenic activity (Benameur et al., 2010; Ma et al., 2017; Xue et al., 2018). In a study, cardiomyocyte derived exosomes containing heat shock protein (Hsp20) showed increased EC proliferation by interacting with VEGF receptor-2 (Zhang et al., 2012). This finding highlighted the key role of exosomes in tissue restorative processes. Notch-Dll4 signaling has been extensively studied in relation to angiogenesis, whereby the expression of Dll4 ligand in tip cells regulates the sprouting of ECs (Gerhardt et al., 2003; Kangsamaksin et al., 2014; Pitulescu et al., 2017). In a 3D matrix microenvironment, exosomes containing Dll4 freely moved to target ECs and mediated efficient Notch activation upon interaction with the recipient ECs (Sharghi-Namini et al., 2014). In addition, Dll4-containing exosomes increased EC motility while decreased proliferation. Dll4 is known to be present during tissue reparative processes, and targeting Dll4 will be critical to mediating efficient angiogenic recovery in injured tissues. Angiogenesis is also regulated by the activities of MMPs, which mediate cell-matrix or cell-cell interaction during the migratory phase. In this context, MMP14 containing exosomes have been shown to cleave VEGFR1 and promote VEGF-A induced migration and proliferation of ECs (Han et al., 2019).
Ding et al. (2019) have studied the effects of exosomes derived from bone marrow MSCs and found that they have superior angiogenic properties and enhance cell proliferation. The authors additionally found that deferoxamine conditioned exosomes activate the PI3/AKT pathway, thereby enhancing cell proliferation and decreasing wound lesions (Ding et al., 2019). Interestingly, exosomes derived from MSCs released high levels of the proangiogenic molecule stromal cell derived factor 1 (SDF1), which not only prevented apoptotic cell death of myocardial cells but also induced cardiac EC regeneration in a mouse model of myocardial infarction (Gong et al., 2019). Hypoxia inducible factor-1α (HIF-1α) is an important mediator of angiogenic activity during ischemic insult. Exosomes prepared from human umbilical cord MSCs have been found to enhance fracture repair and angiogenesis in a rat model of stabilized fracture through HIF1α (Zhang et al., 2019). Beyond studies of heart and skin injury models, the ability of exosome mediated regeneration has also been tested in a mouse model of traumatic brain injury (Gao et al., 2018). Here, the authors addressed the role of exosomes derived from endothelial colony forming cells in their ability to restore the blood brain barrier continuity (Gao et al., 2018). However, whether exosomes can also mediate EC regeneration via EndoMT in addition to the above mentioned mechanisms remain incompletely understood. Figure 1 and Table 2 summarize some of the key genomic and non-genomic cargoes implicated in the regeneration of ECs and angiogenesis.
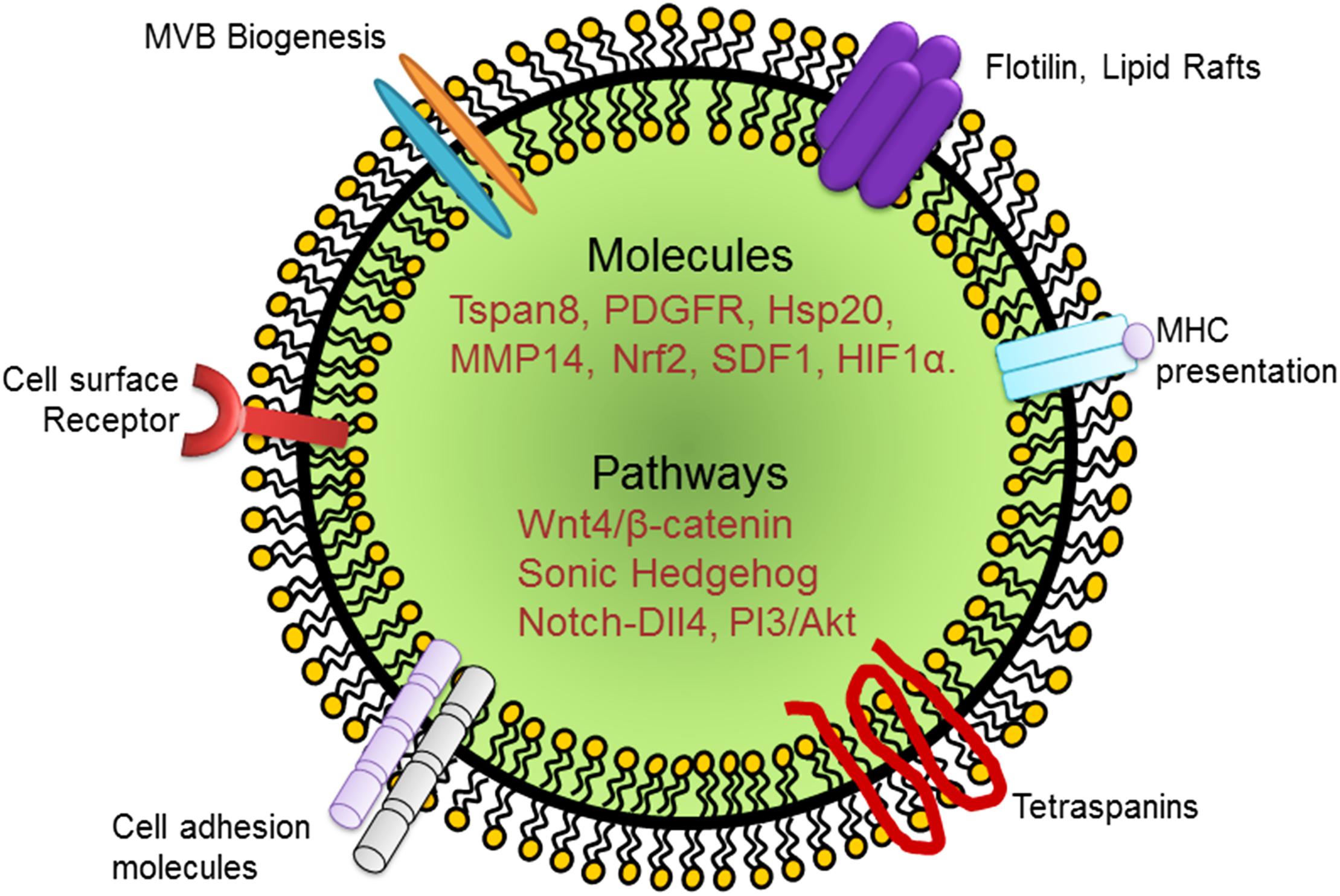
Figure 1. Schematic representation of an exosome and its cargo carrying growth factors, cytokines, and signaling molecules that have been implicated in EC regeneration. PDGFR, platelet derived growth factor receptor; Hsp, heat shock protein; MMP, matrix metalloprotease; SDF, stromal derived factor; HIF, hypoxia inducible factor.
Exosomes With Genomic Cargoes That Mediate EC Regeneration
In addition to transporting growth factors and receptors, exosomes possess the unique ability to transfer miRNAs to recipient cells. miRNAs regulate downstream signaling events through base pairing of their seed sequence with complementary mRNA (Lu and Clark, 2012). MSCs, as well as ECs, contain different regulatory miRNAs, which alter cellular function in target cells (Hromada et al., 2017; Ferguson et al., 2018). In this context, miRNA-146a enriched exosomes secreted from cardiosphere-derived cells have been shown to enhance angiogenesis while simultaneously stimulating proliferation and inhibiting apoptosis of cardiomyocytes (Ibrahim et al., 2014). Exosomes derived from embryonic stem cells have also been exploited in this regard. Accordingly, mouse ESC-derived exosomes have been shown to provide beneficial effects in regeneration after myocardial injury via miR-294 (Khan et al., 2015). Different miRNAs have been implicated in this reparative process. In a more recent study, miR-21-3p enriched exosomes secreted by human umbilical cord blood cells have been shown to accelerate cutaneous wound healing and promote angiogenic events (Hu et al., 2018). Yet another interesting study conducted in a patient population with myocardial ischemia has reported that coronary serum exosomes regulated angiogenesis through miR-939 in this sample group (Li et al., 2018). Human adipose derived stem cell exosomes also exert similar proangiogenic effects via miR-423-5p and Sufu (Xu et al., 2019). In addition, exosomes loaded with miR-210 exert beneficial effects favoring EC function and reoxygenation (Ma et al., 2018). Thus, exosomes can transport different combinations of miRNAs depending on the tissue environment and cell type (Kim et al., 2012; Ferguson et al., 2018). We have listed a select group of miRNA cargoes transported by exosomes known to regulate EC regeneration and angiogenesis in Figure 2 and Tables 2, 3. Nevertheless, continued analyses of the miRNA compositions of various exosomes should be useful in designing custom exosomes for the induction of potent EC regeneration in relation to angiogenesis and revascularization of ischemic cardiovascular tissues. A complete understanding of the role of exosomes in regenerative process in the aftermath of myocardial infarction could bridge an important gap in knowledge of the repair mechanism after myocardial injury.
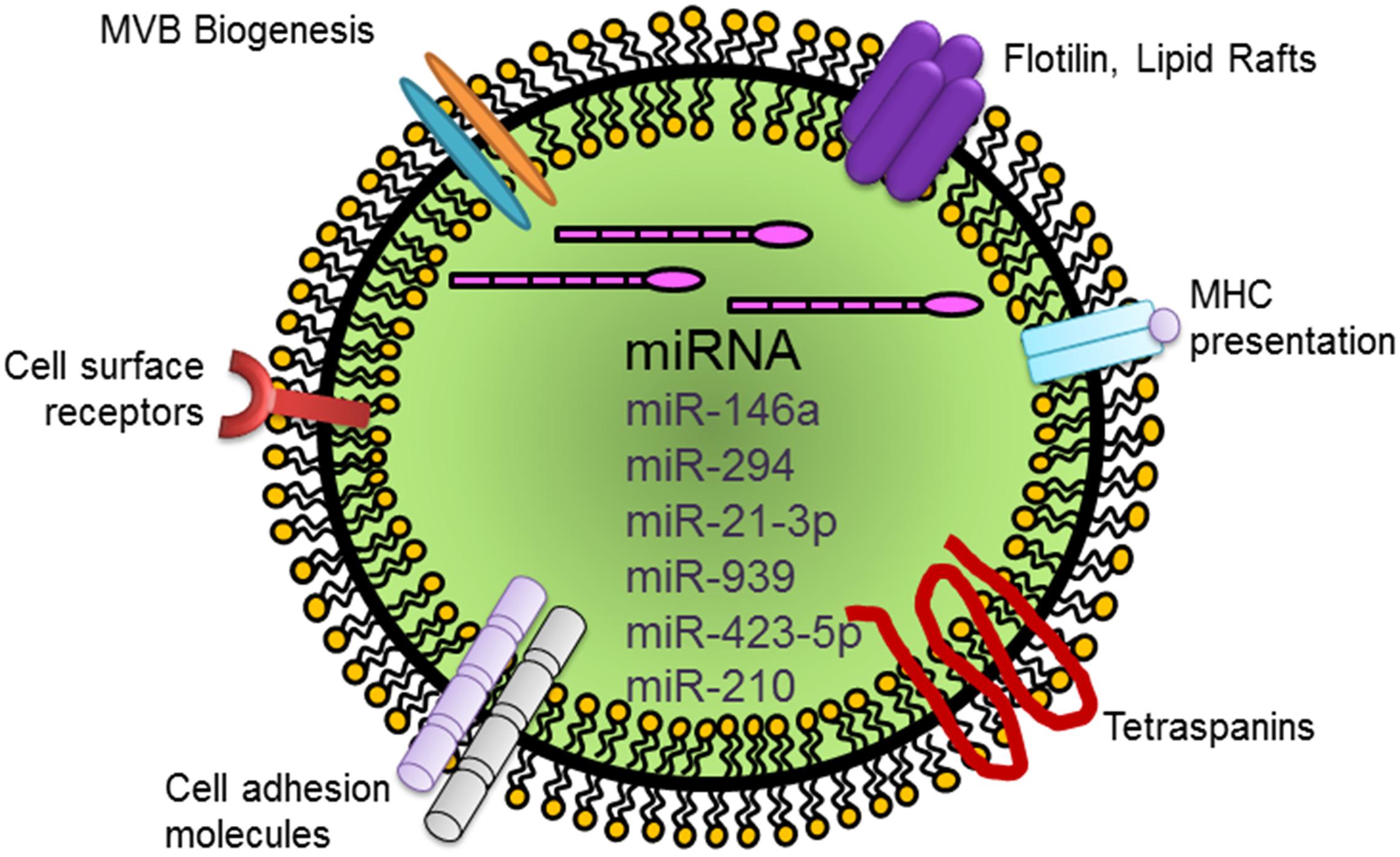
Figure 2. Schematic representation of a generic exosome and its cargo carrying miRNAs that mediate EC regeneration. Abbreviation: miRNA, microRNA.
Future Perspectives
The capacity of the exosomes to induce EC regeneration should benefit organ repair and survival after injury. EC regeneration and the ways in which therapeutic exosomes contribute to this process have the potential in treating ischemic cardiovascular diseases. Thus, substantial progress has been made in the field of exosome research, providing insights into exosome composition and function. Ongoing methodological and technical innovations are beginning to help further synthesize new knowledge, functional understanding and potential applications. However, detail studies are needed to address the possible heterogeneity of exosomes and how this new knowledge could benefit the understanding of EC regeneration and EC pathology. For example, are there specific stimuli that induce the release of “exosomes” that mediate angiogenic activities of ECs, but do not alter the behavior of any other cell type? Are there exosomes that induce rapid proliferation of ECs, but not non-ECs? Are there specific exosomes that inhibit fibrosis, but induce productive wound healing in the aftermath of acute myocardial infarction? These are some of the few questions that come to mind as we ponder the future of exosomes in applications in EC regeneration and re-establishing blood flow to the ischemic cardiovascular organs. Studies have attempted to determine the regenerative ability of exosomes primarily in inbred (e.g., C57BL/6) mouse strains, in experiments such as hind limb ischemia and myocardial infarction. Usually, C57BL/6 mice show robust EC regenerative activities. The question remains whether exosomes provide potent EC regenerative responses in a strain-specific manner. Experiments are also needed in clinically relevant models, for example, mice with defective revascularization potential, such as diabetes. Unraveling the molecular and functional attributes of exosomes and how they may be harnessed should contribute meaningfully to the pursuit of controlling the biology of ECs for regenerative therapy. The answers to these questions and concerns should arrive soon, as new technological innovations such as organoids, data science, computational modeling and artificial intelligence are being incorporated into cardiovascular research (Garikipati et al., 2018; Trac et al., 2019).
Conclusion
In principle, more than one mechanism is likely to be involved in regulating EC repair and regeneration, and reestablishing flow of blood to the ischemic organs. However, there are technical challenges that must be addressed before exosomes could be used as therapeutic biologics from bench to bedside. In this review, we have attempted to summarize how the cargo composition of exosomes derived from several human and non-human sources might benefit EC repair and regeneration. It would be a “giant leap” to be able to reprogram autologous somatic cells directly to ECs by using exosomes, thereby eliminating the use of viral vectors. However, continued research will be required before this interesting idea can be translated into therapy through EC regeneration and restoration of cardiovascular function.
Author Contributions
Both authors wrote, corrected, and reviewed the final manuscript. KW submitted the manuscript online.
Funding
This work was supported in part by the American Heart Association (AHA) Grants GRNT33700162 and TPA34910205 to KW.
Conflict of Interest
The authors declare that the research was conducted in the absence of any commercial or financial relationships that could be construed as a potential conflict of interest.
References
Abid Hussein, M. N., Böing, A. N., Sturk, A., Hau, C. M., and Nieuwland, R. (2017). Inhibition of microparticle release triggers endothelial cell apoptosis and detachment. Thromb. Haemost. 98, 1096–1107. doi: 10.1160/TH05-04-0231
Adamiak, M., and Sahoo, S. (2018). Exosomes in myocardial repair: advances and challenges in the development of next-generation therapeutics. Mol. Ther. 26, 1635–1643. doi: 10.1016/j.ymthe.2018.04.024
Adams, R. H., and Alitalo, K. (2007). Molecular regulation of angiogenesis and lymphangiogenesis. Nat. Rev. Mol. Cell Biol. 8, 464–478. doi: 10.1038/nrm2183
Agarwal, U., George, A., Bhutani, S., Ghosh-Choudhary, S., Maxwell, J. T., Brown, M. E., et al. (2017). Experimental, systems, and computational approaches to understanding the MicroRNA-mediated reparative potential of cardiac progenitor cell-derived exosomes from pediatric patients. Circ. Res. 120, 701–712. doi: 10.1161/CIRCRESAHA.116.309935
Asahara, T., Murohara, T., Sullivan, A., Silver, M., van der Zee, R., Li, T., et al. (1997). Isolation of putative progenitor endothelial cells for angiogenesis. Science 275, 964–967.
Balbi, C., Piccoli, M., Barile, L., Papait, A., Armirotti, A., Principi, E., et al. (2017). First characterization of human amniotic fluid stem cell extracellular vesicles as a powerful paracrine tool endowed with regenerative potential. Stem Cells Transl. Med. 6, 1340–1355. doi: 10.1002/sctm.16-0297
Barile, L., Gherghiceanu, M., Popescu, L. M., Moccetti, T., and Vassalli, G. (2012). Ultrastructural evidence of exosome secretion by progenitor cells in adult mouse myocardium and adult human cardiospheres. J. Biomed. Biotechnol. 2012, 354605–354605. doi: 10.1155/2012/354605
Baruah, J., Hitzman, R., Zhang, J., Chaudhuri, S., Mastej, V., and Wary, K. K. (2017). The allosteric glycogen synthase kinase-3 inhibitor NP12 limits myocardial remodeling and promotes angiogenesis in an acute myocardial infarction model. J. Biol. Chem. 292, 20785–20798. doi: 10.1074/jbc.M117.814376
Beltrami, C., Besnier, M., Shantikumar, S., Shearn, A. I. U., Rajakaruna, C., Laftah, A., et al. (2017). Human pericardial fluid contains exosomes enriched with cardiovascular-expressed MicroRNAs and promotes therapeutic angiogenesis. Mol. Ther. 25, 679–693. doi: 10.1016/j.ymthe.2016.12.022
Benameur, T., Soleti, R., Porro, C., Andriantsitohaina, R., and Martínez, M. C. (2010). Microparticles carrying Sonic hedgehog favor neovascularization through the activation of nitric oxide pathway in mice. PLoS One 5:e12688. doi: 10.1371/journal.pone.0012688
Bentley, K., and Chakravartula, S. (2017). The temporal basis of angiogenesis. Philos. Trans. R. Soc. B Biol. Sci. 372:20150522. doi: 10.1098/rstb.2015.0522
Bian, S., Zhang, L., Duan, L., Wang, X., Min, Y., and Yu, H. (2013). Extracellular vesicles derived from human bone marrow mesenchymal stem cells promote angiogenesis in a rat myocardial infarction model. J. Mol. Med. 92, 387–397. doi: 10.1007/s00109-013-1110-5
Bian, X., Ma, K., Zhang, C., and Fu, X. (2019). Therapeutic angiogenesis using stem cell-derived extracellular vesicles: an emerging approach for treatment of ischemic diseases. Stem Cell Res. Ther. 10, 158–158. doi: 10.1186/s13287-019-1276-z
Bollini, S., Smits, A. M., Balbi, C., Lazzarini, E., and Ameri, P. (2018). Triggering endogenous cardiac repair and regeneration via extracellular vesicle-mediated communication. Front. Physiol. 9:1497. doi: 10.3389/fphys.2018.01497
Boulanger, C. M., Loyer, X., Rautou, P.-E., and Amabile, N. (2017). Extracellular vesicles in coronary artery disease. Nat. Rev. Cardiol. 14, 259–272. doi: 10.1038/nrcardio.2017.7
Carmeliet, P. (2005). Angiogenesis in life, disease and medicine. Nature 438, 932–936. doi: 10.1038/nature04478
Caruso, S., and Poon, I. K. H. (2018). Apoptotic cell-derived extracellular vesicles: more than just debris. Front. Immunol. 9:1486. doi: 10.3389/fimmu.2018.01486
Cheng, L., Zhang, K., Wu, S., Cui, M., and Xu, T. (2017). Focus on mesenchymal stem cell-derived exosomes: opportunities and challenges in cell-free therapy. Stem Cells Int. 2017:6305295. doi: 10.1155/2017/6305295
Cheng, M., Yang, J., Zhao, X., Zhang, E., Zeng, Q., Yu, Y., et al. (2019). Circulating myocardial microRNAs from infarcted hearts are carried in exosomes and mobilise bone marrow progenitor cells. Nat. Commun. 10:959. doi: 10.1038/s41467-019-08895-7
Chistiakov, A. D., Orekhov, N. A., and Bobryshev, V. Y. (2016). Cardiac extracellular vesicles in normal and infarcted heart. Int. J. Mol. Sci. 17:E63. doi: 10.3390/ijms17010063
Coultas, L., Chawengsaksophak, K., and Rossant, J. (2005). Endothelial cells and VEGF in vascular development. Nature 438, 937–945. doi: 10.1038/nature04479
Dejana, E., and Kühl, M. (2010). The role of Wnt signaling in physiological and pathological angiogenesis. Circ. Res. 107, 943–952. doi: 10.1161/CIRCRESAHA.110.223750
Dejana, E., and Lampugnani, M. G. (2018). Endothelial cell transitions. Science 362, 746–747. doi: 10.1126/science.aas9432
Dignat-George, F., and Boulanger, C. M. (2011). The Many Faces of Endothelial Microparticles. Arterioscler. Thromb. Vasc. Biol. 31, 27–33. doi: 10.1161/ATVBAHA.110.218123
Ding, J., Wang, X., Chen, B., Zhang, J., and Xu, J. (2019). Exosomes derived from human bone marrow mesenchymal stem cells stimulated by deferoxamine accelerate cutaneous wound healing by promoting angiogenesis. Biomed. Res. Int. 2019:9742765. doi: 10.1155/2019/9742765
Dougherty, J. A., Kumar, N., Noor, M., Angelos, M. G., Khan, M., Chen, C.-A., et al. (2018). Extracellular vesicles released by human induced-pluripotent stem cell-derived cardiomyocytes promote angiogenesis. Front. Physiol. 9:1794. doi: 10.3389/fphys.2018.01794
Ferguson, S. W., Wang, J., Lee, C. J., Liu, M., Neelamegham, S., Canty, J. M., et al. (2018). The microRNA regulatory landscape of MSC-derived exosomes: a systems view. Sci. Rep. 8:1419. doi: 10.1038/s41598-018-19581-x
Gao, W., Li, F., Liu, L., Xu, X., Zhang, B., Wu, Y., et al. (2018). Endothelial colony-forming cell-derived exosomes restore blood-brain barrier continuity in mice subjected to traumatic brain injury. Exp. Neurol. 307, 99–108. doi: 10.1016/j.expneurol.2018.06.001
Garikipati, V. N. S., Shoja-Taheri, F., Davis, M. E., and Kishore, R. (2018). Extracellular vesicles and the application of system biology and computational modeling in cardiac repair. Circ. Res. 123, 188–204. doi: 10.1161/CIRCRESAHA.117.311215
Gerhardt, H., Golding, M., Fruttiger, M., Ruhrberg, C., Lundkvist, A., Abramsson, A., et al. (2003). VEGF guides angiogenic sprouting utilizing endothelial tip cell filopodia. J. Cell Biol. 161, 1163–1177. doi: 10.1083/jcb.200302047
Giusti, I., Delle, M. S., Di Francesco, M., Sanità, P., D’Ascenzo, S., Gravina, G. L., et al. (2016). From glioblastoma to endothelial cells through extracellular vesicles: messages for angiogenesis. Tumour Biol. 37, 12743–12753. doi: 10.1007/s13277-016-5165-0
Gong, X.-H., Liu, H., Wang, S.-J., Liang, S.-W., and Wang, G.-G. (2019). Exosomes derived from SDF1-overexpressing mesenchymal stem cells inhibit ischemic myocardial cell apoptosis and promote cardiac endothelial microvascular regeneration in mice with myocardial infarction. J. Cell. Physiol. 234, 13878–13893. doi: 10.1002/jcp.28070
Han, K.-Y., Chang, J.-H., and Azar, D. T. (2019). MMP14-containing exosomes cleave VEGFR1 and promote VEGFA-induced migration and proliferation of vascular endothelial cells. Invest. Ophthalmol. Vis. Sci. 60, 2321–2329. doi: 10.1167/iovs.18-26277
Henson, P. M., Bratton, D. L., and Fadok, V. A. (2001). Apoptotic cell removal. Curr. Biol. 11, R795–R805. doi: 10.1016/S0960-9822(01)00474-2
Hirschi, K. K. (2012). Hemogenic endothelium during development and beyond. Blood 119, 4823–4827. doi: 10.1182/blood-2011-12-353466
Hromada, C., Mühleder, S., Grillari, J., Redl, H., and Holnthoner, W. (2017). Endothelial extracellular vesicles—promises and challenges. Front. Physiol. 8:275. doi: 10.3389/fphys.2017.00275
Hu, G., Li, Q., Niu, X., Hu, B., Liu, J., Zhou, S., et al. (2015). Exosomes secreted by human-induced pluripotent stem cell-derived mesenchymal stem cells attenuate limb ischemia by promoting angiogenesis in mice. Stem Cell Res. Ther. 6, 10–10. doi: 10.1186/scrt546
Hu, H., Wang, B., Jiang, C., Li, R., and Zhao, J. (2019). Endothelial progenitor cell-derived exosomes facilitate vascular endothelial cell repair through shuttling miR-21-5p to modulate Thrombospondin-1 expression. Clin. Sci. 133, 1629–1644. doi: 10.1042/CS20190188
Hu, Y., Rao, S.-S., Wang, Z.-X., Cao, J., Tan, Y.-J., Luo, J., et al. (2018). Exosomes from human umbilical cord blood accelerate cutaneous wound healing through miR-21-3p-mediated promotion of angiogenesis and fibroblast function. Theranostics 8, 169–184. doi: 10.7150/thno.21234
Ibrahim, A. G.-E., Cheng, K., and Marbán, E. (2014). Exosomes as critical agents of cardiac regeneration triggered by cell therapy. Stem Cell Rep. 2, 606–619. doi: 10.1016/j.stemcr.2014.04.006
Johnson, T. K., Zhao, L., Zhu, D., Wang, Y., Xiao, Y., Oguljahan, B., et al. (2019). Exosomes derived from induced vascular progenitor cells promote angiogenesis in vitro and in an in vivo rat hindlimb ischemia model. Am. J. Physiol. Heart Circ. Physiol. 317, H765–H776. doi: 10.1152/ajpheart.00247.2019
Ju, C., Shen, Y., Ma, G., Liu, Y., Cai, J., Kim, I. M., et al. (2018). Transplantation of cardiac mesenchymal stem cell-derived exosomes promotes repair in ischemic myocardium. J. Cardiovasc. Transl. Res. 11, 420–428. doi: 10.1007/s12265-018-9822-0
Kangsamaksin, T., Tattersall, I. W., and Kitajewski, J. (2014). Notch functions in developmental and tumour angiogenesis by diverse mechanisms. Biochem. Soc. Trans. 42, 1563–1568. doi: 10.1042/BST20140233
Khan, M., Nickoloff, E., Abramova, T., Johnson, J., Verma, S. K., Krishnamurthy, P., et al. (2015). Embryonic stem cell-derived exosomes promote endogenous repair mechanisms and enhance cardiac function following myocardial infarction. Circ. Res 117, 52–64. doi: 10.1161/CIRCRESAHA.117.305990
Kim, H.-S., Choi, D.-Y., Yun, S. J., Choi, S.-M., Kang, J. W., Jung, J. W., et al. (2012). Proteomic analysis of microvesicles derived from human mesenchymal stem cells. J. Proteome Res. 11, 839–849. doi: 10.1021/pr200682z
Kishore, R., and Khan, M. (2016). More than tiny sacks: stem cell exosomes as cell-free modality for cardiac repair. Circ. Res 118, 330–343. doi: 10.1161/CIRCRESAHA.115.307654
Kohler, E. E., Baruah, J., Urao, N., Ushio-Fukai, M., Fukai, T., Chatterjee, I., et al. (2014). Low-dose 6-bromoindirubin-3’-oxime induces partial dedifferentiation of endothelial cells to promote increased neovascularization. Stem Cells 32, 1538–1552. doi: 10.1002/stem.1658
Kowal, J., Arras, G., Colombo, M., Jouve, M., Morath, J. P., Primdal-Bengtson, B., et al. (2016). Proteomic comparison defines novel markers to characterize heterogeneous populations of extracellular vesicle subtypes. Proc. Natl. Acad. Sci. U.S.A. 113, E968–E977. doi: 10.1073/pnas.1521230113
Lee, S., Kim, J. E., Johnson, B. A., Andukuri, A., and Yoon, Y. S. (2017). Direct reprogramming into endothelial cells: a new source for vascular regeneration. Regen. Med. 12, 317–320. doi: 10.2217/rme-2017-0022
Li, H., Liao, Y., Gao, L., Zhuang, T., Huang, Z., Zhu, H., et al. (2018). Coronary serum exosomes derived from patients with myocardial ischemia regulate angiogenesis through the miR-939-mediated nitric oxide signaling pathway. Theranostics 8, 2079–2093. doi: 10.7150/thno.21895
Li, X., Chen, C., Wei, L., Li, Q., Niu, X., Xu, Y., et al. (2016). Exosomes derived from endothelial progenitor cells attenuate vascular repair and accelerate reendothelialization by enhancing endothelial function. Cytotherapy 18, 253–262. doi: 10.1016/j.jcyt.2015.11.009
Liu, M., Zhang, L., Marsboom, G., Jambusaria, A., Xiong, S., Toth, P. T., et al. (2019). Sox17 is required for endothelial regeneration following inflammation-induced vascular injury. Nat. Commun. 10:2126. doi: 10.1038/s41467-019-10134-y
Loges, S., Fehse, B., Brockmann, M. A., Lamszus, K., Butzal, M., Guckenbiehl, M., et al. (2004). Identification of the adult human hemangioblast. Stem Cells Dev. 13, 229–242. doi: 10.1089/154732804323099163
Lu, J., and Clark, A. G. (2012). Impact of microRNA regulation on variation in human gene expression. Genome Res. 22, 1243–1254. doi: 10.1101/gr.132514.111
Ludwig, N., and Whiteside, T. L. (2018). Potential roles of tumor-derived exosomes in angiogenesis. Expert Opin. Ther. Targets 22, 409–417. doi: 10.1080/14728222.2018.1464141
Ma, J., Zhao, Y., Sun, L., Sun, X., Zhao, X., Sun, X., et al. (2017). Exosomes derived from Akt-modified human umbilical cord mesenchymal stem cells improve cardiac regeneration and promote angiogenesis via activating platelet-derived growth factor D. Stem Cells Transl. Med. 6, 51–59. doi: 10.5966/sctm.2016-0038
Ma, X., Wang, J., Li, J., Ma, C., Chen, S., Lei, W., et al. (2018). Loading MiR-210 in endothelial progenitor cells derived exosomes boosts their beneficial effects on hypoxia/reoxygeneation-injured human endothelial cells via protecting mitochondrial function. Cell. Physiol. Biochem. 46, 664–675. doi: 10.1159/000488635
Manavski, Y., Lucas, T., Glaser, S. F., Dorsheimer, L., Günther, S., Braun, T., et al. (2018). Clonal expansion of endothelial cells contributes to ischemia-induced neovascularization. Circ. Res. 122, 670–677. doi: 10.1161/CIRCRESAHA.117.312310
McDonald, A. I., Shirali, A. S., Aragón, R., Ma, F., Hernandez, G., Vaughn, D. A., et al. (2018). Endothelial regeneration of large vessels is a biphasic process driven by local cells with distinct proliferative capacities. Cell Stem Cell 23, 210.e6–225.e6. doi: 10.1016/j.stem.2018.07.011
McKelvey, K. J., Powell, K. L., Ashton, A. W., Morris, J. M., and McCracken, S. A. (2015). Exosomes: mechanisms of uptake. J. Circ. Biomark 4:7. doi: 10.5772/61186
Medina, R. J., Barber, C. L., Sabatier, F., Dignat-George, F., Melero-Martin, J. M., Khosrotehrani, K., et al. (2017). Endothelial progenitors: a consensus statement on nomenclature. Stem Cells Transl. Med. 6, 1316–1320. doi: 10.1002/sctm.16-0360
Miyagawa, K., Shi, M., Chen, P. I., Hennigs, J. K., Zhao, Z., Wang, M., et al. (2019). Smooth muscle contact drives endothelial regeneration by BMPR2-notch1-mediated metabolic and epigenetic changes. Circ. Res. 124, 211–224. doi: 10.1161/CIRCRESAHA.118.313374
Monaghan, M. G., Linneweh, M., Liebscher, S., Van Handel, B., Layland, S. L., and Schenke-Layland, K. (2016). Endocardial-to-mesenchymal transformation and mesenchymal cell colonization at the onset of human cardiac valve development. Development 143, 473–482. doi: 10.1242/dev.133843
Nazarenko, I., Rana, S., Baumann, A., McAlear, J., Hellwig, A., Trendelenburg, M., et al. (2010). Cell surface tetraspanin Tspan8 contributes to molecular pathways of exosome-induced endothelial cell activation. Cancer Res. 70, 1668–1678. doi: 10.1158/0008-5472.CAN-09-2470
Ni, J., Liu, X., Yin, Y., Zhang, P., Xu, Y.-W., and Liu, Z. (2019). Exosomes derived from TIMP2-modified human umbilical cord mesenchymal stem cells enhance the repair effect in rat model with myocardial infarction possibly by the Akt/Sfrp2 pathway. Oxid. Med. Cell Longev. 2019:1958941. doi: 10.1155/2019/1958941
Park, C., Kim, T. M., and Malik, A. B. (2013). Transcriptional regulation of endothelial cell and vascular development. Circ. Res. 112, 1380–1400. doi: 10.1161/CIRCRESAHA
Pitulescu, M. E., Schmidt, I., Giaimo, B. D., Antoine, T., Berkenfeld, F., Ferrante, F., et al. (2017). Dll4 and Notch signalling couples sprouting angiogenesis and artery formation. Nat. Cell Biol. 19, 915–927. doi: 10.1038/ncb3555
Qiu, J., and Hirschi, K. K. (2019). Endothelial cell development and its application to regenerative medicine. Circ. Res. 125, 489–501. doi: 10.1161/CIRCRESAHA.119.311405
Rafii, S. (2000). Circulating endothelial precursors: mystery, reality, and promise. J. Clin. Invest. 105, 17–19. doi: 10.1172/jci8774
Ribeiro, M. F., Zhu, H., Millard, R. W., and Fan, G.-C. (2013). Exosomes function in pro- and anti-angiogenesis. Curr. Angiogenes. 2, 54–59. doi: 10.2174/22115528113020020001
Ribeiro-Rodrigues, T. M., Laundos, T. L., Pereira-Carvalho, R., Batista-Almeida, D., Pereira, R., Coelho-Santos, V., et al. (2017). Exosomes secreted by cardiomyocytes subjected to ischaemia promote cardiac angiogenesis. Cardiovasc. Res. 113, 1338–1350. doi: 10.1093/cvr/cvx118
Sahoo, S., Klychko, E., Thorne, T., Misener, S., Schultz, K. M., Millay, M., et al. (2011). Exosomes from human CD34(+) stem cells mediate their proangiogenic paracrine activity. Circ. Res. 109, 724–728. doi: 10.1161/CIRCRESAHA.111.253286
Sahoo, S., and Losordo, D. W. (2014). Exosomes and cardiac repair after myocardial infarction. Circ. Res. 114, 333–344. doi: 10.1161/CIRCRESAHA.114.300639
Shabbir, A., Cox, A., Rodriguez-Menocal, L., Salgado, M., and Van Badiavas, E. (2015). Mesenchymal stem cell exosomes induce proliferation and migration of normal and chronic wound fibroblasts, and enhance angiogenesis in vitro. Stem Cells Dev. 24, 1635–1647. doi: 10.1089/scd.2014.0316
Shanmuganathan, M., Vughs, J., Noseda, M., and Emanueli, C. (2018). Exosomes: basic biology and technological advancements suggesting their potential as ischemic heart disease therapeutics. Front. Physiol. 9:1159. doi: 10.3389/fphys.2018.01159
Sharghi-Namini, S., Tan, E., Ong, L.-L. S., Ge, R., and Asada, H. H. (2014). Dll4-containing exosomes induce capillary sprout retraction in a 3D microenvironment. Sci. Rep. 4:4031. doi: 10.1038/srep04031
Sietsema, W. K., Kawamoto, A., Takagi, H., and Losordo, D. W. (2019). Autologous CD34+ cell therapy for ischemic tissue repair. Circ. J. 83, 1422–1430. doi: 10.1253/circj.CJ-19-0240
Skog, J., Würdinger, T., van Rijn, S., Meijer, D. H., Gainche, L., Sena-Esteves, M., et al. (2008). Glioblastoma microvesicles transport RNA and proteins that promote tumour growth and provide diagnostic biomarkers. Nat. Cell Biol. 10, 1470–1476. doi: 10.1038/ncb1800
Teng, X., Chen, L., Chen, W., Yang, J., Yang, Z., and Shen, Z. (2015). Mesenchymal stem cell-derived exosomes improve the microenvironment of infarcted myocardium contributing to angiogenesis and anti-inflammation. Cell. Physiol. Biochem. 37, 2415–2424. doi: 10.1159/000438594
Théry, C., Amigorena, S., Raposo, G., and Clayton, A. (2006). Isolation and characterization of exosomes from cell culture supernatants and biological fluids. Curr. Protoc. Cell Biol. 30, 3.22.1–3.22.29. doi: 10.1002/0471143030.cb0322s30
Théry, C., Witwer, K. W., Aikawa, E., Alcaraz, M. J., Anderson, J. D., Andriantsitohaina, R., et al. (2018). Minimal information for studies of extracellular vesicles 2018 (MISEV2018): a position statement of the International Society for Extracellular Vesicles and update of the MISEV2014 guidelines. J. Extracell. Vesicles 7:1535750. doi: 10.1080/20013078.2018.1535750
Trac, D., Hoffman, J. R., Bheri, S., Maxwell, J. T., Platt, M. O., and Davis, M. E. (2019). Predicting functional responses of progenitor cell exosome potential with computational modeling. Stem Cells Transl. Med. 8, 1212–1221. doi: 10.1002/sctm.19-0059
Vlassov, A. V., Magdaleno, S., Setterquist, R., and Conrad, R. (2012). Exosomes: current knowledge of their composition, biological functions, and diagnostic and therapeutic potentials. Biochim. Biophys. Acta Gen. Subj. 1820, 940–948. doi: 10.1016/j.bbagen.2012.03.017
Waldenström, A., Gennebäck, N., Hellman, U., and Ronquist, G. (2012). Cardiomyocyte microvesicles contain DNA/RNA and convey biological messages to target cells. PLoS One 7:e34653. doi: 10.1371/journal.pone.0034653
Wang, H. U., Chen, Z.-F., and Anderson, D. J. (1998). Molecular distinction and angiogenic interaction between embryonic arteries and veins revealed by ephrin-B2 and its receptor Eph-B4. Cell 93, 741–753. doi: 10.1016/S0092-8674(00)81436-1
Wang, X., Gu, H., Huang, W., Peng, J., Li, Y., Yang, L., et al. (2016). Hsp20-mediated activation of exosome biogenesis in cardiomyocytes improves cardiac function and angiogenesis in diabetic mice. Diabetes 65, 3111–3128. doi: 10.2337/db15-1563
Williams, I. M., and Wu, J. C. (2019). Generation of endothelial cells from human pluripotent stem cells. Arterioscler. Thromb. Vasc. Biol. 39, 1317–1329. doi: 10.1161/ATVBAHA.119.312265
Xu, F., Xiang, Q., Huang, J., Chen, Q., Yu, N., Long, X., et al. (2019). Exosomal miR-423-5p mediates the proangiogenic activity of human adipose-derived stem cells by targeting Sufu. Stem Cell Res, Ther. 10:106. doi: 10.1186/s13287-019-1196-y
Xue, C., Shen, Y., Li, X., Li, B., Zhao, S., Gu, J., et al. (2018). Exosomes derived from hypoxia-treated human adipose mesenchymal stem cells enhance angiogenesis through the PKA signaling pathway. Stem Cells Dev. 27, 456–465. doi: 10.1089/scd.2017.0296
Yang, Y., García-Verdugo, J. M., Soriano-Navarro, M., Srinivasan, R. S., Scallan, J. P., Singh, M. K., et al. (2012). Lymphatic endothelial progenitors bud from the cardinal vein and intersomitic vessels in mammalian embryos. Blood 120, 2340–2348. doi: 10.1182/blood-2012-05-428607
Ye, M., Ni, Q., Qi, H., Qian, X., Chen, J., Guo, X., et al. (2019). Exosomes derived from human induced pluripotent stem cells-endothelia cells promotes postnatal angiogenesis in mice bearing ischemic limbs. Int. J. Biol. Sci. 15, 158–168. doi: 10.7150/ijbs.28392
Yoder, M. C. (2018). Endothelial stem and progenitor cells (stem cells): (2017 grover conference series). Pulm. Circ. 8:2045893217743950. doi: 10.1177/2045893217743950
Zhang, B., Wu, X., Zhang, X., Sun, Y., Yan, Y., Shi, H., et al. (2015). Human umbilical cord mesenchymal stem cell exosomes enhance angiogenesis through the Wnt4/β-catenin pathway. Stem Cells Transl. Med. 4, 513–522. doi: 10.5966/sctm.2014-0267
Zhang, X., Wang, X., Zhu, H., Kranias, E. G., Tang, Y., Peng, T., et al. (2012). Hsp20 functions as a novel cardiokine in promoting angiogenesis via activation of VEGFR2. PLoS One 7:e32765. doi: 10.1371/journal.pone.0032765
Keywords: angiogenesis, exosomes, endothelial cells, regeneration, rejuvenation
Citation: Baruah J and Wary KK (2020) Exosomes in the Regulation of Vascular Endothelial Cell Regeneration. Front. Cell Dev. Biol. 7:353. doi: 10.3389/fcell.2019.00353
Received: 16 August 2019; Accepted: 05 December 2019;
Published: 09 January 2020.
Edited by:
Sveva Bollini, University of Genoa, ItalyReviewed by:
Lucio Barile, University of Zurich, SwitzerlandMahmood Khan, The Ohio State University, United States
Copyright © 2020 Baruah and Wary. This is an open-access article distributed under the terms of the Creative Commons Attribution License (CC BY). The use, distribution or reproduction in other forums is permitted, provided the original author(s) and the copyright owner(s) are credited and that the original publication in this journal is cited, in accordance with accepted academic practice. No use, distribution or reproduction is permitted which does not comply with these terms.
*Correspondence: Kishore K. Wary, a2t3YXJ5QHVpYy5lZHU=