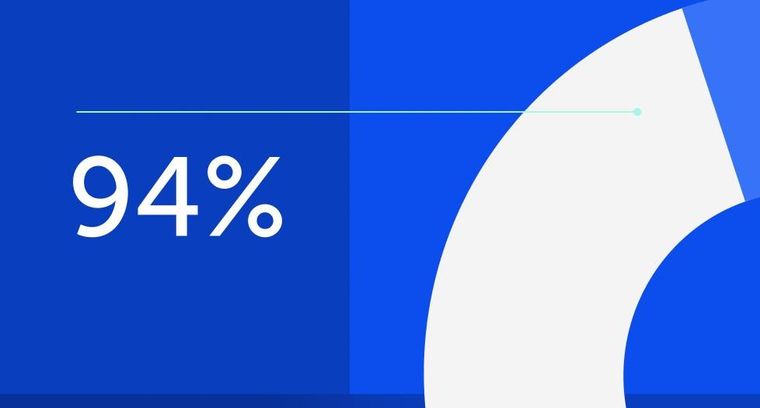
94% of researchers rate our articles as excellent or good
Learn more about the work of our research integrity team to safeguard the quality of each article we publish.
Find out more
REVIEW article
Front. Cell Dev. Biol., 10 December 2019
Sec. Cell Growth and Division
Volume 7 - 2019 | https://doi.org/10.3389/fcell.2019.00332
This article is part of the Research TopicUnexpected Consequences of Telomere and Telomerase DysfunctionView all 3 articles
Telomerase plays a critical role in stem cell function and tissue regeneration that depends on its ability to elongate telomeres. For nearly two decades, it turned out that TERT regulates a broad spectrum of functions including signal transduction, gene expression regulation, and protection against oxidative damage that are independent of its telomere elongation activity. These conclusions that were mainly obtained in cell lines overexpressing telomerase were further strengthened by in vivo models of ectopic expression of telomerase or models of G1 TERT knockout mice without detectable telomere dysfunction. However, the later models were questioned due to the presence of aberrantly shortened telomere in the germline of the parents TERT+/– that were used to create the G1 TERT–/– mice. The physiological relevance of the functions associated with overexpressed telomerase raised also some concerns due to artifactual situations and localizations and complications to quantify the level of TERT. Another concern with non-canonical functions of TERT was the difficulty to separate a direct TERT-related function from secondary effects. Despite these concerns, more and more evidence accumulates for non-canonical roles of telomerase that are non-obligatory extra-telomeric. Here, we review these non-canonical roles of the TERT subunit of telomerase. Also, we emphasize recent results that link TERT to mitochondria and protection to reactive oxygen species suggesting a protective role of TERT in neurons. Throughout this review, we dissect some controversies regarding the non-canonical functions of telomerase and provide some insights to explain these discrepancies. Finally, we discuss the importance of understanding these alternative functions of telomerase for the development of anticancer strategies.
Mammalian telomeres are specialized DNA and protein structures found at the very ends of linear chromosomes. They consist of hexameric DNA 5′-TTAGGGn repeats associated with a specific shelterin complex of six proteins and are essential for chromosomal maintenance and genomic stability (de Lange, 2018). Telomeres shorten when normal cells undergo each replication due to the “end replication problem,” resulting mostly from incomplete lagging-strand DNA synthesis and the induction of exonucleolytic processing at the leading strand (Lingner et al., 1995; Faure et al., 2010; Lam et al., 2010; Wu et al., 2012). Telomere shortening is compensated by telomerase, a specialized ribonucleoprotein (RNP) complex that contains at least two major components and several accessory proteins (Shay and Wright, 2019). The first major component is a protein with reverse transcriptase (RT) activity, the human telomerase RT (hTERT). This enzyme extends the telomeric DNA by adding short repetitive DNA sequences. The other component is a functional RNA, the human telomerase RNA (hTR), which functions as a template to direct the synthesis of telomeric repeats by hTERT. The complex is associated with accessory proteins such as dyskerin (DKC1), telomerase Cajal body protein 1 (TCAB1), non-histone chromosome protein 2 (NHP2), nucleolar protein 10 (NOP10), and GAR1 RNP (GAR1). Telomerase homeostasis depends highly on the coordinated regulation of telomerase RNP assembly, trafficking, and recruitment to telomeres (Schmidt and Cech, 2015).
Telomerase is expressed in adult stem cells, including skin, intestines, and hematopoietic stem/progenitor cells. Telomerase is transiently activated only in certain proliferating stem-like cells, such as T cell upon activation. However, upon differentiation, this transient activation goes off (Holt et al., 1996; Greenberg et al., 1998). The mechanisms of this transient activation and subsequent repression upon differentiation remained yet unknown. In cancer cells, the situation is different since telomerase that counteracts telomere shortening and senescence is reactivated (Bodnar et al., 1998). Telomerase activation occurs in 85–90% of all human cancers (Kim et al., 1994; Shay and Bacchetti, 1997) while 10–15% of cancers maintain their telomeres through a DNA recombination pathway (named ALT for alternative lengthening of telomeres) (Bryan et al., 1997). Reactivation of telomerase activity is mainly dependent on the increased expression of the hTERT gene (Yamaguchi et al., 2003) that appears to be the limiting component in cancer cells for the formation of the active enzyme. However, even though hTR is broadly expressed in normal cells (Feng et al., 1995), it is also often deregulated during tumorigenesis (Soder et al., 1997, 1998; Heine et al., 1998; Yamaguchi et al., 2003). Different estimations of the endogenous levels of hTR and hTERT protein and the assembled telomerase RNP were reported (Yi et al., 2001; Cohen et al., 2007; Xi and Cech, 2014). However, telomerase quantification was hampered by the difficulty to detect low level of the endogenous protein and also by technical limitations (see below). Studies using both RT-qPCR and northern report that hTR levels were often in excess over telomerase RNP complexes in cancer cells (Xi and Cech, 2014) (approximately 1150 hTR molecules in HeLa cells, whereas only approximately 500 molecules of hTERT) suggesting the existence of unassembled components. Therefore, a pool of hTERT-free hTR might assemble with other protein components and could demonstrate alternative functions independent of telomerase in cell survival and apoptosis as mentioned by some reports (Kedde et al., 2006; Gazzaniga and Blackburn, 2014). In the same vein, in HEK293 and HeLa approximately 240 telomerase complexes per cell have been estimated suggesting that free hTERT protein may not be assembled to hTR raising the question of independent functions of hTR and hTERT. As hTERT has a favored affinity for RNA, it is likely to interact with other long non-coding RNA than hTR (Nelson and Shippen, 2015; El Hajj et al., 2018). This possibility of hTERT interaction with non-conventional partners (not only proteins but also RNAs) provides interesting new insights into extratelomeric functions of telomerase.
Indeed, in the last 15 years it appeared that telomerase functions could not be restricted to telomeres and the list of telomere-unrelated functions progressively increased. Increasing studies reported a wider spectrum of telomerase functions including signal transduction pathways, gene expression regulation, and mitochondrial function with consequences on control cell survival, proliferation, differentiation, migration, and regeneration (Passos et al., 2007; Martinez and Blasco, 2011; Chiodi and Mondello, 2012; Li and Tergaonkar, 2014; Zhou et al., 2014; Miwa and Saretzki, 2017). These non-canonical functions of telomerase appear not only in mammals but have been also discovered in zebrafish (Imamura et al., 2008; Alcaraz-Perez et al., 2014). However, even though evidence indicates that telomerase elicits other functions, it is not excluded that some of the consequences associated with telomerase expression even if they are not related to telomerase elongation function, may be explained by functions at telomeres such as protective functions or telomere chromatin regulation. Some of them necessitate the RT domain, while some are TR independent. In this review, we briefly described the consequences of the critical shortening of telomeres and then summarize and discuss the proposed non-canonical roles of telomerase in particular in the context of its reactivation in cancer. Since many excellent reviews have been published on the extra-telomeric functions of TERT (Ding et al., 2013; Li and Tergaonkar, 2014; Saretzki, 2014; Maida and Masutomi, 2015; Teichroeb et al., 2016; Romaniuk et al., 2019; Yuan and Xu, 2019), the main purpose of this review will be to discuss some discrepancies found in the literature regarding the non-canonical functions of telomerase and discuss how can we explain these differences.
The main pathway in response to telomere erosion is the p53-dependent tumor suppressive mechanism that downregulates the expression of several factors involved not only in cell cycle control but also in DNA repair and telomere maintenance (Vogelstein et al., 2000). This transcriptional repression is coordinated by the p21–DREAM complex that acts downstream p53 and binds E2F and cell cycle genes homology region (CHR) elements (Engeland, 2018). Activation of p53 in response to telomere dysfunction is also associated with defective mitochondrial biogenesis through repression of peroxisome proliferator-activated receptor gamma co-activator 1 alpha/beta (PGC-1α/β) (Sahin et al., 2011) and downregulation of all sirtuins including mitochondrial sirtuins Sirt3, 4, and 5 further extending the links between short telomeres and metabolic control (Amano et al., 2019). Besides, changes in telomere structure due to telomere shortening may release telomeric factors such as Rap1 and TRF2 that can associate with non-telomeric sites (Martinez et al., 2010; Yang et al., 2011; Ye et al., 2014). Such binding of telomeric factors outside telomeric regions can influence the transcription of genes involved in processes as different as metabolism, immunity, and differentiation (Martínez et al., 2013; Yeung et al., 2013; Cherfils-Vicini et al., 2019; Zizza et al., 2019). Along the same line, the shelterin protein TIN2 can be post-translationally processed in mitochondria and promotes oxidative phosphorylation unveiling unsuspected links between a shelterin protein and ROS production (Chen et al., 2012). In addition, short telomeres can trigger epigenetic changes at telomeric and subtelomeric chromatin and in TElomeric Repeat-containing RNA (TERRA) transcription that may perturb cell homeostasis (Porro et al., 2014a, b; Tardat and Déjardin, 2018). These results illustrate the complex response to telomere erosion and the intimate relationship between telomere attrition and exhaustion of proliferative potential, metabolic control, differentiation, and reduced lifespan. This was exemplified in vivo by the fact that the degenerative phenotypes, including neurodegeneration of mice with severe telomere dysfunction associated with repressed mouse TERT (mTERT) expression, were rescued by reactivating the expression of telomerase (Jaskelioff et al., 2011).
Telomerase was first recognized for its telomere-lengthening activity. However, it is now well established that TERT participates in a variety of biological pathways independently of its telomere-lengthening function. These pathways are involved both in physiological processes including stem cell function, tissue homeostasis, and aging, and oncogenesis by promoting many features involved in tumorigenesis, tumor progression, and resistance to treatments. TERT is now well recognized to act as a transcription (co-)factor to regulate gene expression. Some examples are discussed below, the two most-studied signaling pathways regulated by hTERT being the Wnt/β-catenin and the nuclear factor kappa-light-chain-enhancer of activated B cells (NF-κB) pathways.
In vivo studies carried out in mice lay out the premises for extratelomeric roles of TERT in tumorigenesis. mTERT expression at high levels in transgenic mice (associated with an increased telomerase activity) in several tissues such as mammary gland was associated with spontaneous development of mammary intraepithelial neoplasia and invasive mammary carcinomas (Artandi et al., 2002). This suggested that TERT expression could promote the development of spontaneous cancer in mice with wild-type (WT) telomere length (Artandi et al., 2002). In another experiment using the GM847 cell line that maintains its telomere by ALT, expression of oncogenic RAS failed to fully transform the cells. Nonetheless, these cells acquired a tumorigenic phenotype upon ectopic expression of TERT suggesting that TERT had an additional function required for cellular transformation independent of its ability to maintain telomeres (Stewart et al., 2002). Along the same line, ectopic expression of telomerase in primary human mammary epithelial cells (HMECs) identified an apparently telomere-independent function of hTERT that allowed HMECs to proliferate in mitogen-deficient conditions through the induction of several growth-promoting genes, including the epidermal growth factor receptor (EGFR) (Smith et al., 2003). Moreover, transcriptional profiling of normal and TERT-immortalized fibroblasts identified 172 differentially expressed genes in TERT-immortalized cells, one of them epiregulin being a potent growth factor associated with cancer. These results suggested that both activation of telomerase and subsequent induction of epiregulin were required to sustain cell proliferation (Lindvall et al., 2003). These observations, therefore, suggested that telomerase activation promotes tumorigenesis and immortalization independently of its essential function in telomere elongation.
Despite pieces of evidence indicating telomerase had extratelomeric roles, some controversies persisted in the field. In a landmark paper (Sarin et al., 2005), mTERT was expressed under the control of a tetracycline responsive promoter allowing the conditional expression of mTERT. Activation of mTERT in mice (that were otherwise WT for the endogenous telomerase) promoted the proliferation of hair follicle bulge cells. Importantly, the effects related to this artificially induced expression of mTERT did not require the telomerase RNA component (Sarin et al., 2005) and was proficient in a telomerase mutant carrying a point mutation abolishing telomerase catalytic activity (Choi et al., 2008). These data provided an in vivo evidence that ectopically expressed mouse telomerase, likely above a certain level, promoted proliferation of resting cells. As an ectopic expression of telomerase did not reflect a physiological situation, it remains, however, to be determined whether it could be relevant in the context of cancer cells (that are known to reactivate telomerase expression). Therefore, the question arises about whether these extratelomeric functions of TERT operate in cancer cells, and above which threshold. It was further shown by the same group that mTERT interacted with BRG1, the central catalytic subunit of numerous chromatin-modifying enzymatic complexes (Trotter and Archer, 2008) either in co-immunoprecipitation with ES cells or in vitro in pull-down experiments (Park et al., 2009). Because BRG1 interacts with members of the Wnt signaling pathway (Barker et al., 2001) whose stimulation activates quiescent epidermal stem cells, and also because transcriptomic profiling unveiled similarities between TERT and Wnt transcriptional responses (Choi et al., 2008), Park et al. (2009) tested whether mTERT could activate the Wnt pathway ex vivo (in cells depleted or not for BRG1) and in vivo by inducing the expression of mTERT placed under the control of a tetracycline regulated promoter. They measured Wnt signaling in the gastrointestinal tract using mice engineered with a Wnt-reporter (Park et al., 2009). Collectively, their data showed that mTERT overexpression stimulated the transcription of targets of the Wnt/β-catenin pathway in vitro in a BRG1-dependent way and in vivo in progenitors of the small intestine (Park et al., 2009). Again, the question related to this work was whether mTERT could activate the Wnt pathway in a WT mouse. Strikingly, they observed that 50% of G1 mTERT–/– mice exhibited homeotic transformation of the vertebrate, resembling the one observed in mice carrying a hypomorphic mutation of WNT3A, while none of the WT counterparts showed this abnormality (Park et al., 2009). The authors elegantly proposed that stimulation of progenitor cells by the Wnt pathway should somehow be coordinated to telomere maintenance and such coupling could have led to the incorporation of telomerase as a co-factor of β-catenin in the Wnt signaling pathway (Park et al., 2009). Finally, the same group showed in mouse that conditional expression of mTERT or a catalytically dead mTERT in the kidney induced podocyte proliferation and resulted in collapsing glomerulopathy (Shkreli et al., 2011). Consistent with their previous results, Wnt signaling was activated in the podocytes that re-enter the cell-cycle suggesting that Wnt signaling could underline podocyte activation upon mTERT expression.
However, these conclusions were questioned in several independent articles. By analyzing phenotypes of heterozygous mTERT+/– mice in backgrounds with different telomere length settings, Strong et al. (2011) reported that mTERT+/– and mTERT–/– displayed similar phenotypes with progressive breeding that correlated with telomere shortening. Moreover, they found that in the CAST/EiJ genetic background (short telomere setting), mTERT and mTR deficiencies produced indistinguishable phenotypes. In addition, Wnt was activated similarly in WT or mTERT–/– cells (Strong et al., 2011). These results strengthened the admitted notion that short telomeres, and not the absence of telomerase, are at the root of tissue renewal limitation. Of note, these results were not really in contradiction with the results from Park et al. (2009) since the latter mainly investigate the effects of ectopic/overexpression of telomerase and not its absence. The only apparent discrepancy between the two studies was the fact that Strong et al. (2011) could not find abnormal ribs in mTERT–/– mice in contrast to what was observed by Park et al. (2009), when they examined homeotic transformations in G1 TERT–/– mice. To explain this discrepancy, it was suggested that mTERT function in regulating Wnt could be compensated in the germline of telomerase knockout mice.
In a second article, Listerman et al. (2014) analyzed the ability of hTERT to modulate Wnt signaling in several breast cancer cell lines endowed with different levels of telomerase activity. In breast cancer cell lines expressing a high level of telomerase, additional overexpression of hTERT did not activate Wnt signaling reporters while a mild activation was observed in a cell line that was originally endowed with mild-telomerase activity. The authors concluded that hTERT did not stimulate Wnt target transcription universally but instead did so in a context-dependent way. These results did not support those from Park et al. (2009) but they were not strictly contradictory. More annoying was the questioning of the interaction between hTERT and BRG1. However, both co-immunoprecipitations were done in different contexts. While Park et al. (2009) immunoprecipitated endogenous HA-tagged mTERT (tagged at its locus) in mouse ES-cells and Listerman et al. (2014) analyzed the interaction between overproduced FLAG-hTERT and BRG1 in HeLa cells. Again, the context was different. Of note, in an independent study, ectopically expressed FLAG-hTERT isolated from HeLa cells was shown to interact with endogenous BRG1 and nucleostemin. This complex was shown to assemble during mitosis and to play a role in the maintenance of heterochromatin at repetitive elements found at centromeres and retrotransposons (Maida et al., 2014). Noteworthy, nucleostemin is involved in telomere protection through the regulation of TRF1 sumoylation and its subsequent association with the promyelocytic leukemia protein isoform IV (PMLIV) in telomerase-negative cells (Hsu et al., 2012). Finally, BRG1 and p54 (nrb) were reported to cooperate in regulating TERT splicing and promoting the production of full-length TERT mRNA. This action seems independent of its interaction with β-catenin (Ito et al., 2008).
It turned out that in a more recent article, late-generation hTR–/– mice were shown to exhibit downregulation of either factor involved of the canonical WNT signaling, or targets of WNT pathway in the intestinal crypt epithelia (Yang et al., 2017). Pharmacological treatments that restored expression of components of the WNT pathway as well as one of the WNT targets in the epithelium of hTR–/– also restored telomere capping in the mutant epithelium. Importantly, this improved telomere capping was explained by the restoration of shelterin protein expression, including TRF2 and Pot1a rather than by telomere lengthening (Yang et al., 2017). This result was consistent with a previous study showing that TRF2 was transcriptionally activated by the Wnt/β-catenin signaling pathway in mouse intestinal tissues (Diala et al., 2013). Given that telomerase expression is also positively regulated by Wnt in the context of stem cells and cancer (Hoffmeyer et al., 2012), the results indicate that the Wnt signaling pathway, telomerase expression, and telomere capping by shelterin proteins mutually reinforce themselves through a positive feedback loop (Yang et al., 2017). In the light of these results, the same team proposed that the developmental transformations in the axial skeleton that were observed in G1 mTERT–/– could be due to the presence of one or a few numbers of single aberrantly shortened telomere in the germline of the parents TERT+/– that were used to create the G1 TERT–/– mice (Fernandez and Johnson, 2018). Whether one or a few uncapped telomeres could downregulate the canonical WNT signaling pathway remains an open question.
The first evidence indicating that TERT regulates NF-κB-dependent gene expression was reported in multiple myeloma cells in which NF-κBp65 subunit was found to modulate the nuclear translocation of TERT (Akiyama et al., 2003). Ten years later, it was reported in another landmark paper that TERT stimulated the expression of a number of genes whose transcription is controlled by the NF-κB pathway such as IL6, IL8, and tumor necrosis factor-α (TNFα) (Ghosh et al., 2012). This result was reinforced by the observation that human TERT regulates MMP expression via NF-κB-dependent transcription independently of TERT telomere elongation activity (Ding et al., 2013). Several evidences indicated that TERT regulates NF-κB-dependent gene expression by binding to the NF-κB p65 subunit although no evidence of a direct protein/protein interaction was provided (Ghosh et al., 2012). Because TERT transcription can be stimulated ex vivo by expressing NF-κB (Yin et al., 2000), it was proposed a “feed-forward” regulation between TERT and NF-κB (Li and Tergaonkar, 2014). Since NF-κB is a key regulator of inflammatory and developmental processes, hTERT is likely to regulate inflammation and development through its interplay with NF-κB.
Whereas MYC has been described mostly as a direct activator of TERT transcription by binding to the E-box (5′-CACGYG-3′) in the TERT promoter region (Greenberg et al., 1999; Wu et al., 1999), it appears that TERT also regulates c-Myc. Indeed, recent results indicate that first-generation TERT-null mice exhibit a delayed onset of MYC-induced lymphomagenesis in a lymphoma murine model (Koh et al., 2015). The authors further showed that TERT stabilizes MYC protein levels and regulates its binding to target promoters contributing to either activation or repression of MYC-target genes, independently of its telomeric role. These results unveil another example of feed-forward mechanism potentializing MYC-dependent oncogenesis.
Vascular endothelial growth factor (VEGF) is another example of a factor whose expression is regulated by TERT. TERT was first reported to activate the transcription of VEGF in embryonic lung cells and HeLa cells (Zhou et al., 2009). In support of this finding Liu et al. (2016) observed that in tumor xenograft assays of lung carcinoma carried out in WT or G1 TERT knockout mice (without detectable telomere dysfunction), tumor development as well as VEGF expression were reduced in TERT–/– mice compared to WT suggesting that TERT deficiency affects tumorigenesis and tumor vascular development (Liu et al., 2016). Mechanistically, this activation may occur through hTERT binding to the transcription factor Sp1 at the VEGF promoter in order to stimulate angiogenesis (Liu et al., 2016).
In 2003 was published the first study reporting a novel function of hTERT in the regulation of DNA methyltransferases (DNMTs) (Young et al., 2003). Telomerase introduction in normal human fibroblasts upregulates the expression and the activity of the DNMT1. DNMT1 activity is to maintain the pattern of DNA methylation. Therefore, even though the precise mechanism was not elucidated, the consequence of this increase is the prevention of changes in gene expression related to cellular aging. Recently, by exploring the correlation between hTERT and DNMT3B (DNMTs 3B) in both hepatocarcinoma (HCC) cell lines and primary HCC tumors, Yu et al. (2018) reported that TERT up-regulated DNMT3B expression. This DNMTs that is required for de novo methylation of CpG dinucleotides cooperated with Sp1 to activate DNMT3B promoter activity. Indeed, Sp1 inhibition significantly attenuated TERT-mediated DNMT3B up-regulation, while its over-expression restored DNMT3B expression in TERT-depleted cancer cells (Yu et al., 2018). One of the consequences of TERT-induced DNMT3B up-regulation was the aberrant methylation of the tumor suppressor PTEN promoter and its subsequent silencing. As PTEN inhibits PI3K/AKT signaling, TERT-mediated PTEN silencing led to an increase of AKT activity which in turn promoted cell survival and proliferation providing another example of the role of TERT in carcinogenesis.
Physical protection of telomeres by capping may explain in some cases the prevention of telomere damages due to oxidative stress (as reported in Fanconi Anemia) (Uziel et al., 2008). An additional function of TERT in modulating the DNA damage response (DDR) was proposed by Masutomi et al. (2005) who reported that the down-regulation of hTERT reduced the level of H2AX phosphorylation and ATM autophosphorylation in fibroblasts exposed to DNA damaging agents while telomere length was unchanged. They proposed that the sustained loss of hTERT expression compromised the DDR through an alteration of chromatin structure. Recently, it was discovered that small non-coding RNAs (dilncRNA) were produced at DNA double-strand breaks that were critical for DDR activation (Michelini et al., 2017). It is tempting to speculate that the effect mediated by TERT on the DDR might be due to interference between TERT and the generation of the dilncRNA.
Many other articles reported in different cellular systems that telomerase was protecting against oxidative stress and that this protection was associated with reduced levels of reactive oxygen species (ROS) (Armstrong et al., 2005; Ahmed et al., 2008; Haendeler and Klotz, 2008; Indran et al., 2011; Mattiussi et al., 2012; Spilsbury et al., 2015). However, opposing findings complicated the understanding of the interplay between telomerase ectopic expression and oxidative stress. Several reports initially showed that hTERT was found not only in the nucleus but also in the cytoplasm and mitochondria (Haendeler et al., 2003; Santos et al., 2004; Sharma et al., 2012). The move out of hTERT from the nucleus into the mitochondria was in particular triggered by oxidative stress (Santos et al., 2006; Ahmed et al., 2008; Singhapol et al., 2013). It was shown in human fibroblasts that the accumulation of overexpressed TERT in mitochondria under oxidative stress protected mitochondria DNA (mtDNA) against acute or oxidative damage and decreased superoxide production and cellular ROS levels (Ahmed et al., 2008). In an independent study, it was shown that an overexpressed C-terminus Myc-tagged TERT was localized into the mitochondrial matrix consistent with the fact that TERT has a matrix-targeting sequence at its N-terminus (Haendeler et al., 2009). In the same study, it was shown by chromatin immunoprecipitation that TERT-Myc bound two mtDNA regions called ND1 and ND2 (NADH ubiquinone oxidoreductase subunits 1 and 2) of complex I and protected mtDNA from UV-light damage. However, this result was challenged by the finding that hTERT rather binds to mtDNA non-specifically or through a widely distributed cis-element (Sharma et al., 2012). Haendeler et al. (2009) further showed that inactivating the catalytic activity of TERT reduced by 30% the respiratory chain activity of TERT-transfected cells suggesting that TERT catalytic activity was required to protect the respiratory chain. They proposed that TERT under certain circumstances increased the respiratory chain activity through complex I (Haendeler et al., 2009; Ale-Agha et al., 2014). Related studies indicated that mitochondrial hTERT worked as a RT with tRNAs or other RNAs involved in mtDNA replication but not with hTR (Sharma et al., 2012). Whether hTERT contributes to replication or repair of mtDNA during oxidative stress and how its RT activity contributes to this process remains unknown. Further work is required to directly link the mitochondrial action of hTERT to its mitochondria protective effect.
Oddly, the mitochondria protective effect of hTERT was opposite to the one reported by Santos et al. (2004, 2006) few years ago in which ectopic expression of hTERT in response to H2O2 resulted in higher levels of mtDNA damage and increase in apoptotic cell death in a way that required the hTERT catalytic activity (Santos et al., 2004, 2006). It may be that expressing TERT at different levels differentially regulate signaling pathway as described above raising the possibility that different effects of TERT overlap to produce the observed phenotypes. The same authors recently reported a differential activation of autophagy in human fibroblasts according to the subcellular localization of TERT in response to H2O2. They proposed that the expression of an hTERT mutant lacking mitochondrial localization exhibited increased autophagy as a rescue pathway to compensate for the loss of hTERT in mitochondria (Green et al., 2019).
Beyond the mtDNA centric view of hTERT action, other explanations were provided to account for reduced endogen ROS production in response to oxidative stress. The effect of hTERT would result from the combined effects of nuclear and mitochondrial hTERT. Within the nucleus, the modulation of signaling pathways by hTERT (such as the NF-κB pathway) would contribute to antioxidant hTERT effect by promoting the transcription of antioxidant factors including MnSOD and g-glutamylcysteine (Indran et al., 2011). In mitochondria, the imported hTERT would improve the mitochondrial activity as illustrated by the higher activity of cytochrome C oxidase (Indran et al., 2011). Related to this study, the impact of hTERT was evaluated in response to TNF-α, an activator of NF-κB signaling pathway and a powerful inducer of endogenous ROS (Mattiussi et al., 2012). It was shown that hTERT overexpression in human foreskin fibroblasts repressed ROS-dependent activation of ERK1/2 protein kinases and of superoxide dismutase 2 (SOD2) providing additional insights that hTERT represses ROS-dependent intracellular signaling (Mattiussi et al., 2012). A recent study also identifies a BH3-like motif in hTERT protein (Jin et al., 2018). This motif is found in most Bcl2 family proteins. The same study reports an interaction between hTERT and two anti-apoptotic Bcl2 protein family, Mcl1 and Bcl-xL, and also with BECN1, a BH3-only protein involved in autophagy supporting another functional link between hTERT and the mitochondria biology.
All these results indicate intricate links between telomerase, ROS, and mitochondria. However, many of these results were obtained in cellular systems designed to strongly overproduce hTERT. The question remains about the relevance of the anti-oxidative function of hTERT during development or in response to stresses in adult in specific tissues or in cancer cells. Recent results indicate that the protein TERT persists in the adult brain while telomerase activity was shut-down before birth (Ulaner et al., 1998; Iannilli et al., 2013; Ishaq et al., 2016; Miwa et al., 2016; Miwa and Saretzki, 2017). In mouse, TERT is expressed in several regions of the adult brain such as the olfactory bulb, hippocampus, cortex, and cerebellum (Caporaso et al., 2003; Flanary and Streit, 2003; Eitan et al., 2012). Interestingly, in mouse Purkinje neurons, TERT localization to mitochondria was increased after exposure to X-ray radiation or to high glutamate levels suggesting that telomerase could have specific roles in protecting neurons exposed to excitotoxic and genotoxic stresses (Eitan et al., 2016). Further work using animal models will be required to confirm the relevance of these fascinating observations.
Although much is going to be learned upon these new telomere unrelated roles of telomerase, studies may generate different interpretations because of the multiplicity of the models and the lack of accurate tools to detect the endogenous TERT and measure its activity. This can explain some of the discrepancies in the published results pointing out that alternative approaches have to be taken to quantify the level of TERT, determine its cellular localization, or identify its partners. Overall, there is a need to develop appropriate experimental tools to go further in the elucidation of the TERT functions in normal physiology and the context of tumors.
Despite the presence of telomerase activity in cancer cells, the mRNA abundance is low compared to other genes even in tumor cells (Yi et al., 2001; Xi and Cech, 2014) making it difficult to detect and quantify accurately and this represents a major obstacle. Telomerase activity is currently measured using a PCR-based assay (telomerase repeated amplification protocol or TRAP assay), which is very sensitive but not very accurate and susceptible to artifacts. Experiments based on TERT overexpression (either WT or mutant variants) have to be taken with caution since the levels obtained are generally much more important than those observed in tumor cells. Indeed, overexpression can alter hTERT localization (high expression in both nuclei and cytoplasm) and the recruitment to telomeres or give rise to artificial protein interactions. Furthermore, TERT overexpression in telomerase positive cells can affect telomere length. It is now documented that telomere length can control some gene expression near the ends of the chromosome either by telomere position effect (TPE) or by TPE over long distances (TPE-OLD) (Baur et al., 2001; Koering et al., 2002; Kim et al., 2016). Therefore, TERT overexpression may modulate gene expression by these processes. This modulation, therefore, occurs indirectly through a telomere-dependent mechanism. Of note, the human TERT gene is located at the very end of chromosome 5, near the telomere. Therefore, TPE-OLD mechanisms could be effective mechanisms in TERT gene regulation. In contrast, in mice TERT gene is on chromosome 13 and the telomeric location is not conserved. Consequently, the local genomic structure around TERT locus is quite different between mice and humans. These observations suggest that different strategies for TERT regulation have evolved between human and mice. Therefore, although mouse models prove to be extremely useful to unravel telomerase functions, we have to be aware that many of them do not systematically mimic human diseases, including cancers, and caution must be used in drawing definitive conclusions.
Reliable detection of the endogenous TERT protein has proven difficult due to the low abundance of TERT protein and the lack of available specific antibodies (Wu et al., 2006). Several experiments demonstrating interaction involving TERT protein rely mainly on immunoprecipitation using TERT antibodies that were not properly validated. Another concern is that in several experiments tags have been fused to TERT to detect the presence of the protein or its activity. However, it has to be checked whether the modifications introduced in the TERT protein does not modify its functions. Indeed, it has been shown, for example, that C-terminal tagging impairs the ability of telomerase to elongate telomeres within cells even though the activity of the protein measured in vitro was retained (Banik et al., 2002).
Among the mechanisms involved in TERT regulation, one is alternative splicing. To date, 22 and 31 splice variants of hTERT have been described in human and in chicken, respectively (Kilian et al., 1997; Wick et al., 1999; Hisatomi et al., 2003; Chang and Delany, 2006; Saeboe-Larssen et al., 2006; Amor et al., 2010). As most of these variants are disrupted in the major functional domains of the TERT protein, they may result in an inactive telomerase complex in terms of telomere elongation. However, accumulating evidence points to potential functions for some of these variants. For example, a human variant, containing an in-frame deletion that removed exons 4–13 (Δ4–13) and encoding the catalytic domain of telomerase was described (Hrdlickova et al., 2012). This variant (that produces a truncated protein) was expressed in telomerase-negative as well as in telomerase-positive cell lines (Hrdlickova et al., 2012). Importantly, its overexpression leads to elevated proliferation rates of several cell types by stimulation of Wnt signaling. Note that another study of splice variants reported that the deletion variant lacking most of the RT domain is highly expressed in stem and cancer cells. It inhibits telomerase activity and confers a growth advantage to breast cancer cells independent of telomere maintenance, by inhibiting cisplatin-induced apoptosis (Listerman et al., 2013). It is known that alternative splicing is deregulated in cancers leading to the expression of splice variants detected during development but silenced in normal cells. Therefore, many cancers show changes in TERT splicing patterns. As TERT splicing plays a major role in dictating the activity of telomerase at the telomeres, it remains to be assessed whether these splice variants have other non-conventional activities. The fact that many non-canonical functions of full-length TERT do not require its catalytic activity support evidence for possible functions of alternatively spliced TERT isoforms. This notion is further corroborated by the study using a panel of TERT mutants transduced into HMECs, which shows that TERT had biological actions in cell proliferation and DNA damage signaling that could be genetically separated and functionally independent (Mukherjee et al., 2011).
The existence of the TERT splice variants may complicate greatly the interpretation of expression data as these are generally not taken into account in the quantification of TERT mRNA levels because of technical and methodological limitations to quantify their expression. The most common assay to measure hTERT expression is quantitative RT-PCR using primers designed to detect exons 5–9 localized in the catalytic RT domain. However, these exons are precisely belonging to those subjected to splicing, leading to misinterpretation of the data regarding the expression of hTERT. Furthermore, depending on the cell line or tissue studied, the amount of the full-length protein coding TERT RNA can represent 1–90% of all the TERT transcripts (Yi et al., 2001). It is also important to note that alternative splicing of TERT is not the same in human and mice (Sayed et al., 2019). The major transcript in mice for TERT is the full-length transcript whereas in humans most TERT transcripts are alternatively spliced to variants incompetent for the elongation of telomeres. However, these variants may have conserved the alternative functions of TERT or even novel functions that remained to be identified. Of note, certain normal cells have been observed to express “non-functional” transcripts (Fan et al., 2005; Li et al., 2008; Wong et al., 2014). The physiological significance of this observation remains unclear but support that these variants could have a role in cell physiology. As previously mentioned, the poor availability of TERT antibody and the lack of reliable methods for the quantification of each isoform variant transcript hamper the functional characterization of these variants. Indeed, the most common assays to measure hTERT transcripts are designed to detect exons 5–9 in the RT domain. However, since in human these exons are spliced out in most transcripts, the conclusions drawn may have been misinterpreted. In a recent study, it was reported that TERT transcript are expressed in normal cell and tissues at a level similar to the one measured in cancer cells suggesting that the major difference in telomerase activity between normal and cancer cells relies in a shift in splicing (Kim et al., 2016). Emerging sequencing technologies will prove helpful to quantify splice variants. Finally, it is also important to note that TERT alternative splicing in the mouse is not the same as in human (Sayed et al., 2019). Indeed, in mouse cells the majority of TERT transcripts contain the RT domain able to produce an active TERT protein while in humans the RT domain of human TERT is spliced out in most of the transcripts.
As pointed out, an accumulation of evidence indicates that TERT plays important functions far from telomere maintenance. These functions can impact cancer cells by giving them a selective advantage. Presently, most telomerase inhibitors designed to inhibit telomerase activity (i.e., synthesis of the telomeric repeats) are not well tolerated by the patients. Indeed, therapy must be prolonged because it could take a long period before telomeres are shortened enough to induce death of the cancer cell. To be fully efficient, it could be necessary to target different functions of hTERT instead of solely those that inhibit its enzymatic activity or accessibility to telomeres. In this case, therapeutic strategies targeting directly hTERT expression will be highly effective and more prompt as it will target all TERT functions. In this line of view, it has been shown that retinoic acid can inhibit the transcription of hTERT leading to cell death. The induction of the death can be the result of telomere shortening but it has been also demonstrated that the repression of hTERT can sensitize cells to conventional chemotherapy in a telomere independent manner (Dudognon et al., 2004; Deville et al., 2011; Samy et al., 2012). Indeed TERT can increase resistance to chemotherapeutic agents. These results encourage the integration of strategies targeting the expression of hTERT (as retinoic acid) into extended trials performed in vitro and clinical practice. Besides, it is clear that in some cases as telomerase is associated with many extratelomeric functions, total inhibition of its expression could induce severe and unpredictable side effects. It is now clear that the therapeutic exploitation of the biology of the telomeres requires the integration of the multiple roles of telomerase in the physiology of not only cancer cells but also normal cells. Therefore, to develop new strategies of telomerase inhibition for anticancer therapy, a more thorough understanding of all the functions of the telomerase complex (conventional and alternative) and the interplay between these functions in the physiology of the cell is necessary.
Telomerase has been described as having a key role in several signaling pathways that are frequently altered in cancers. The major role of hTERT in cancer has been related to the maintenance of telomere length that is a prerequisite event for the tumor cells to survive and proliferate. However, telomere unrelated functions have emerged to allow increased proliferation, resistance to cell death, inflammation, invasion, and metastasis. Therefore, endowed with these non-telomeric functions, TERT can participate to all the major characteristics of the cancer phenotype described by Hanahan and Weinberg (2000, 2011). However, as pointed out in this review, the complexity of the interconnecting signaling networks and feed-forward responses illustrates the difficulty of drawing mechanistic conclusions.
Both authors contributed equally to the work and approved it for publication.
This work was supported by the “Ligue Nationale Contre le Cancer” (LNCC) (Equipe labellisée) and the “Institut National du Cancer (PLBIO-2019). The work of ES-B team was supported by grants from the French National Institute of Health and Medical Research (INSERM), the National Center for Scientific Research (CNRS), and the “Ligue Nationale Contre le Cancer”.
The authors declare that the research was conducted in the absence of any commercial or financial relationships that could be construed as a potential conflict of interest.
We thank Dmitri Churikov (CRCM, Marseille, France) and Eric Gilson (IRCAN, Nice, France) for critically reading this review.
Ahmed, S., Passos, J. F., Birket, M. J., Beckmann, T., Brings, S., Peters, H., et al. (2008). Telomerase does not counteract telomere shortening but protects mitochondrial function under oxidative stress. Journal of cell science 121(Pt 7), 1046–1053. doi: 10.1242/jcs.019372
Akiyama, M., Hideshima, T., Hayashi, T., Tai, Y. T., Mitsiades, C. S., Mitsiades, N., et al. (2003). Nuclear factor-kappaB p65 mediates tumor necrosis factor alpha-induced nuclear translocation of telomerase reverse transcriptase protein. Cancer research 63, 18–21.
Alcaraz-Perez, F., Garcia-Castillo, J., Garcia-Moreno, D., Lopez-Munoz, A., Anchelin, M., Angosto, D., et al. (2014). A non-canonical function of telomerase RNA in the regulation of developmental myelopoiesis in zebrafish. Nature communications 5, 3228. doi: 10.1038/ncomms4228
Ale-Agha, N., Dyballa-Rukes, N., Jakob, S., Altschmied, J., and Haendeler, J. (2014). Cellular functions of the dual-targeted catalytic subunit of telomerase, telomerase reverse transcriptase–potential role in senescence and aging. Exp Gerontol 56, 189–193. doi: 10.1016/j.exger.2014.02.011
Amano, H., Chaudhury, A., Rodriguez-Aguayo, C., Lu, L., Akhanov, V., Catic, A., et al. (2019). Telomere Dysfunction Induces Sirtuin Repression that Drives Telomere-Dependent Disease. Cell Metab 29, 1274.e–1290.e. doi: 10.1016/j.cmet.2019.03.001
Amor, S., Remy, S., Dambrine, G., Le Vern, Y., Rasschaert, D., and Laurent, S. (2010). Alternative splicing and nonsense-mediated decay regulate telomerase reverse transcriptase (TERT) expression during virus-induced lymphomagenesis in vivo. BMC cancer 10:571. doi: 10.1186/1471-2407-10-571
Armstrong, L., Saretzki, G., Peters, H., Wappler, I., Evans, J., Hole, N., et al. (2005). Overexpression of telomerase confers growth advantage, stress resistance, and enhanced differentiation of ESCs toward the hematopoietic lineage. Stem Cells 23, 516–529. doi: 10.1634/stemcells.2004-0269
Artandi, S. E., Alson, S., Tietze, M. K., Sharpless, N. E., Ye, S., Greenberg, R. A., et al. (2002). Constitutive telomerase expression promotes mammary carcinomas in aging mice. Proceedings of the National Academy of Sciences of the United States of America 99, 8191–8196. doi: 10.1073/pnas.112515399
Banik, S. S., Guo, C., Smith, A. C., Margolis, S. S., Richardson, D. A., Tirado, C. A., et al. (2002). C-terminal regions of the human telomerase catalytic subunit essential for in vivo enzyme activity. Mol Cell Biol 22, 6234–6246. doi: 10.1128/mcb.22.17.6234-6246.2002
Barker, N., Hurlstone, A., Musisi, H., Miles, A., Bienz, M., and Clevers, H. (2001). The chromatin remodelling factor Brg-1 interacts with beta-catenin to promote target gene activation. EMBO J 20, 4935–4943. doi: 10.1093/emboj/20.17.4935
Baur, J. A., Zou, Y., Shay, J. W., and Wright, W. E. (2001). Telomere position effect in human cells. Science 292, 2075–2077. doi: 10.1126/science.1062329
Bodnar, A. G., Ouellette, M., Frolkis, M., Holt, S. E., Chiu, C. P., Morin, G. B., et al. (1998). Extension of life-span by introduction of telomerase into normal human cells. Science 279, 349–352. doi: 10.1126/science.279.5349.349
Bryan, T. M., Englezou, A., Dalla-Pozza, L., Dunham, M. A., and Reddel, R. R. (1997). Evidence for an alternative mechanism for maintaining telomere length in human tumors and tumor-derived cell lines. Nature medicine 3, 1271–1274. doi: 10.1038/nm1197-1271
Caporaso, G. L., Lim, D. A., Alvarez-Buylla, A., and Chao, M. V. (2003). Telomerase activity in the subventricular zone of adult mice. Molecular and cellular neurosciences 23, 693–702. doi: 10.1016/s1044-7431(03)00103-9
Chang, H., and Delany, M. E. (2006). Complicated RNA splicing of chicken telomerase reverse transcriptase revealed by profiling cells both positive and negative for telomerase activity. Gene 379, 33–39. doi: 10.1016/j.gene.2006.04.021
Chen, L. Y., Zhang, Y., Zhang, Q., Li, H., Luo, Z., Fang, H., et al. (2012). Mitochondrial localization of telomeric protein TIN2 links telomere regulation to metabolic control. Mol Cell 47, 839–850. doi: 10.1016/j.molcel.2012.07.002
Cherfils-Vicini, J., Iltis, C., Cervera, L., Pisano, S., Croce, O., Sadouni, N., et al. (2019). Cancer cells induce immune escape via glycocalyx changes controlled by the telomeric protein TRF2. EMBO J 38, e100012. doi: 10.15252/embj.2018100012
Chiodi, I., and Mondello, C. (2012). Telomere-independent functions of telomerase in nuclei, cytoplasm, and mitochondria. Front Oncol 2:133. doi: 10.3389/fonc.2012.00133
Choi, J., Southworth, L. K., Sarin, K. Y., Venteicher, A. S., Ma, W., Chang, W., et al. (2008). TERT promotes epithelial proliferation through transcriptional control of a Myc- and Wnt-related developmental program. PLoS genetics 4:e10. doi: 10.1371/journal.pgen.0040010
Cohen, S. B., Graham, M. E., Lovrecz, G. O., Bache, N., Robinson, P. J., and Reddel, R. R. (2007). Protein composition of catalytically active human telomerase from immortal cells. Science 315, 1850–1853. doi: 10.1126/science.1138596
de Lange, T. (2018). Shelterin-Mediated Telomere Protection. Annu Rev Genet 52, 223–247. doi: 10.1146/annurev-genet-032918-021921
Deville, L., Hillion, J., Pendino, F., Samy, M., Nguyen, E., and Segal-Bendirdjian, E. (2011). hTERT promotes imatinib resistance in chronic myeloid leukemia cells: therapeutic implications. Molecular cancer therapeutics 10, 711–719. doi: 10.1158/1535-7163.MCT-10-0979
Diala, I., Wagner, N., Magdinier, F., Shkreli, M., Sirakov, M., Bauwens, S., et al. (2013). Telomere protection and TRF2 expression are enhanced by the canonical Wnt signalling pathway. EMBO Rep 14, 356–363. doi: 10.1038/embor.2013.16
Ding, D., Zhou, J., Wang, M., and Cong, Y. S. (2013). Implications of telomere-independent activities of telomerase reverse transcriptase in human cancer. The FEBS journal 280, 3205–3211. doi: 10.1111/febs.12258
Dudognon, C., Pendino, F., Hillion, J., Saumet, A., Lanotte, M., and Segal-Bendirdjian, E. (2004). Death receptor signaling regulatory function for telomerase: hTERT abolishes TRAIL-induced apoptosis, independently of telomere maintenance. Oncogene 23, 7469–7474. doi: 10.1038/sj.onc.1208029
Eitan, E., Braverman, C., Tichon, A., Gitler, D., Hutchison, E. R., Mattson, M. P., et al. (2016). Excitotoxic and Radiation Stress Increase TERT Levels in the Mitochondria and Cytosol of Cerebellar Purkinje Neurons. Cerebellum 15, 509–517. doi: 10.1007/s12311-015-0720-6
Eitan, E., Tichon, A., Daniel, G., and Priel, E. (2012). Telomerase expression in adult and old mouse Purkinje neurons. Rejuvenation Res 15, 206–209. doi: 10.1089/rej.2011.1285
El Hajj, J., Nguyen, E., Liu, Q., Bouyer, C., Adriaenssens, E., Hilal, G., et al. (2018). Telomerase regulation by the long non-coding RNA H19 in human acute promyelocytic leukemia cells. Molecular cancer 17, 85. doi: 10.1186/s12943-018-0835-8
Engeland, K. (2018). Cell cycle arrest through indirect transcriptional repression by p53: I have a DREAM. Cell Death Differ 25, 114–132. doi: 10.1038/cdd.2017.172
Fan, Y., Liu, Z., Fang, X., Ge, Z., Ge, N., Jia, Y., et al. (2005). Differential expression of full-length telomerase reverse transcriptase mRNA and telomerase activity between normal and malignant renal tissues. Clinical cancer research 11, 4331–4337. doi: 10.1158/1078-0432.ccr-05-0099
Faure, V., Coulon, S., Hardy, J., and Geli, V. (2010). Cdc13 and telomerase bind through different mechanisms at the lagging- and leading-strand telomeres. Mol Cell 38, 842–852. doi: 10.1016/j.molcel.2010.05.016
Feng, J., Funk, W. D., Wang, S. S., Weinrich, S. L., Avilion, A. A., Chiu, C. P., et al. (1995). The RNA component of human telomerase. Science 269, 1236–1241.
Fernandez, R. J., and Johnson, F. B. (2018). A regulatory loop connecting WNT signaling and telomere capping: possible therapeutic implications for dyskeratosis congenita. Ann N Y Acad Sci 1418, 56–68. doi: 10.1111/nyas.13692
Flanary, B. E., and Streit, W. J. (2003). Telomeres shorten with age in rat cerebellum and cortex in vivo. J Anti Aging Med 6, 299–308. doi: 10.1089/109454503323028894
Gazzaniga, F. S., and Blackburn, E. H. (2014). An antiapoptotic role for telomerase RNA in human immune cells independent of telomere integrity or telomerase enzymatic activity. Blood 124, 3675–3684. doi: 10.1182/blood-2014-06-582254
Ghosh, A., Saginc, G., Leow, S. C., Khattar, E., Shin, E. M., Yan, T. D., et al. (2012). Telomerase directly regulates NF-κB-dependent transcription. Nat Cell Biol 14, 1270–1281. doi: 10.1038/ncb2621
Green, P. D., Sharma, N. K., and Santos, J. H. (2019). Telomerase Impinges on the Cellular Response to Oxidative Stress Through Mitochondrial ROS-Mediated Regulation of Autophagy. Int J Mol Sci 20, 1509. doi: 10.3390/ijms20061509
Greenberg, R. A., Allsopp, R. C., Chin, L., Morin, G. B., and DePinho, R. A. (1998). Expression of mouse telomerase reverse transcriptase during development, differentiation and proliferation. Oncogene 16, 1723–1730. doi: 10.1038/sj.onc.1201933
Greenberg, R. A., O’Hagan, R. C., Deng, H., Xiao, Q., Hann, S. R., Adams, R. R., et al. (1999). Telomerase reverse transcriptase gene is a direct target of c-Myc but is not functionally equivalent in cellular transformation. Oncogene 18, 1219–1226. doi: 10.1038/sj.onc.1202669
Haendeler, J., Dröse, S., Büchner, N., Jakob, S., Altschmied, J., Goy, C., et al. (2009). Mitochondrial telomerase reverse transcriptase binds to and protects mitochondrial DNA and function from damage. Arterioscler Thromb Vasc Biol 29, 929–935. doi: 10.1161/ATVBAHA.109.185546
Haendeler, J., Hoffmann, J., Brandes, R. P., Zeiher, A. M., and Dimmeler, S. (2003). Hydrogen peroxide triggers nuclear export of telomerase reverse transcriptase via Src kinase family-dependent phosphorylation of tyrosine 707. Mol Cell Biol 23, 4598–4610. doi: 10.1128/mcb.23.13.4598-4610.2003
Haendeler, J., and Klotz, L. O. (2008). Highlight: oxidative stress and senescence. Biol Chem 389, 201. doi: 10.1515/bc.2008.044
Hanahan, D., and Weinberg, R. A. (2011). Hallmarks of cancer: the next generation. Cell 144, 646–674. doi: 10.1016/j.cell.2011.02.013
Heine, B., Hummel, M., Demel, G., and Stein, H. (1998). Demonstration of constant upregulation of the telomerase RNA component in human gastric carcinomas using in situ hybridization. The Journal of pathology 185, 139–144. doi: 10.1002/(sici)1096-9896(199806)185:2<139::aid-path79>3.0.co;2-l
Hisatomi, H., Ohyashiki, K., Ohyashiki, J. H., Nagao, K., Kanamaru, T., Hirata, H., et al. (2003). Expression profile of a gamma-deletion variant of the human telomerase reverse transcriptase gene. Neoplasia 5, 193–197. doi: 10.1016/S1476-5586(03)80051-9
Hoffmeyer, K., Raggioli, A., Rudloff, S., Anton, R., Hierholzer, A., Del Valle, I., et al. (2012). Wnt/β-catenin signaling regulates telomerase in stem cells and cancer cells. Science 336, 1549–1554. doi: 10.1126/science.1218370
Holt, S. E., Wright, W. E., and Shay, J. W. (1996). Regulation of telomerase activity in immortal cell lines. Mol Cell Biol 16, 2932–2939. doi: 10.1128/mcb.16.6.2932
Hrdlickova, R., Nehyba, J., and Bose, H. R. Jr. (2012). Alternatively spliced telomerase reverse transcriptase variants lacking telomerase activity stimulate cell proliferation. Mol Cell Biol 32, 4283–4296. doi: 10.1128/MCB.00550-12
Hsu, J. K., Lin, T., and Tsai, R. Y. (2012). Nucleostemin prevents telomere damage by promoting PML-IV recruitment to SUMOylated TRF1. The Journal of cell biology 197, 613–624. doi: 10.1083/jcb.201109038
Iannilli, F., Zalfa, F., Gartner, A., Bagni, C., and Dotti, C. G. (2013). Cytoplasmic TERT Associates to RNA Granules in Fully Mature Neurons: Role in the Translational Control of the Cell Cycle Inhibitor p15INK4B. PLoS One 8:e66602. doi: 10.1371/journal.pone.0066602
Imamura, S., Uchiyama, J., Koshimizu, E., Hanai, J., Raftopoulou, C., Murphey, R. D., et al. (2008). A non-canonical function of zebrafish telomerase reverse transcriptase is required for developmental hematopoiesis. PLoS ONE 3:e3364. doi: 10.1371/journal.pone.0003364
Indran, I. R., Hande, M. P., and Pervaiz, S. (2011). hTERT overexpression alleviates intracellular ROS production, improves mitochondrial function, and inhibits ROS-mediated apoptosis in cancer cells. Cancer Res 71, 266–276. doi: 10.1158/0008-5472.CAN-10-1588
Ishaq, A., Hanson, P. S., Morris, C. M., and Saretzki, G. (2016). Telomerase Activity is Downregulated Early During Human Brain Development. Genes (Basel) 7, 27. doi: 10.3390/genes7060027
Ito, T., Watanabe, H., Yamamichi, N., Kondo, S., Tando, T., Haraguchi, T., et al. (2008). Brm transactivates the telomerase reverse transcriptase (TERT) gene and modulates the splicing patterns of its transcripts in concert with p54(nrb). The Biochemical journal 411, 201–209. doi: 10.1042/bj20071075
Jaskelioff, M., Muller, F. L., Paik, J. H., Thomas, E., Jiang, S., Adams, A. C., et al. (2011). Telomerase reactivation reverses tissue degeneration in aged telomerase-deficient mice. Nature 469, 102–106. doi: 10.1038/nature09603
Jin, Y., You, L., Kim, H. J., and Lee, H. W. (2018). Telomerase Reverse Transcriptase Contains a BH3-Like Motif and Interacts with BCL-2 Family Members. Molecules and cells 41, 684–694. doi: 10.14348/molcells.2018.0206
Kedde, M., Le Sage, C., Duursma, A., Zlotorynski, E., van Leeuwen, B., Nijkamp, W., et al. (2006). Telomerase-independent regulation of ATR by human telomerase RNA. The Journal of biological chemistry 281, 40503–40514. doi: 10.1074/jbc.m607676200
Kilian, A., Bowtell, D. D., Abud, H. E., Hime, G. R., Venter, D. J., Keese, P. K., et al. (1997). Isolation of a candidate human telomerase catalytic subunit gene, which reveals complex splicing patterns in different cell types. Human molecular genetics 6, 2011–2019. doi: 10.1093/hmg/6.12.2011
Kim, N. W., Piatyszek, M. A., Prowse, K. R., Harley, C. B., West, M. D., Ho, P. L., et al. (1994). Specific association of human telomerase activity with immortal cells and cancer. Science 266, 2011–2015. doi: 10.1126/science.7605428
Kim, W., Ludlow, A. T., Min, J., Robin, J. D., Stadler, G., Mender, I., et al. (2016). Regulation of the Human Telomerase Gene TERT by Telomere Position Effect-Over Long Distances (TPE-OLD): Implications for Aging and Cancer. PLoS biology 14:e2000016. doi: 10.1371/journal.pbio.2000016
Koering, C. E., Pollice, A., Zibella, M. P., Bauwens, S., Puisieux, A., Brunori, M., et al. (2002). Human telomeric position effect is determined by chromosomal context and telomeric chromatin integrity. EMBO Rep 3, 1055–1061. doi: 10.1093/embo-reports/kvf215
Koh, C. M., Khattar, E., Leow, S. C., Liu, C. Y., Muller, J., Ang, W. X., et al. (2015). Telomerase regulates MYC-driven oncogenesis independent of its reverse transcriptase activity. J Clin Invest 125, 2109–2122. doi: 10.1172/JCI79134
Lam, Y. C., Akhter, S., Gu, P., Ye, J., Poulet, A., Giraud-Panis, M. J., et al. (2010). SNMIB/Apollo protects leading-strand telomeres against NHEJ-mediated repair. The EMBO journal 29, 2230–2241. doi: 10.1038/emboj.2010.58
Li, W., Li, L., Liu, Z., Liu, C., Liu, Z., Straat, K., et al. (2008). Expression of the full-length telomerase reverse transcriptase (hTERT) transcript in both malignant and normal gastric tissues. Cancer letters 260, 28–36. doi: 10.1016/j.canlet.2007.10.018
Li, Y., and Tergaonkar, V. (2014). Noncanonical functions of telomerase: implications in telomerase-targeted cancer therapies. Cancer research 74, 1639–1644. doi: 10.1158/0008-5472.CAN-13-3568
Lindvall, C., Hou, M., Komurasaki, T., Zheng, C., Henriksson, M., Sedivy, J. M., et al. (2003). Molecular characterization of human telomerase reverse transcriptase-immortalized human fibroblasts by gene expression profiling: activation of the epiregulin gene. Cancer research 63, 1743–1747.
Lingner, J., Cooper, J. P., and Cech, T. R. (1995). Telomerase and DNA end replication: no longer a lagging strand problem? Science 269, 1533–1534. doi: 10.1126/science.7545310
Listerman, I., Gazzaniga, F. S., and Blackburn, E. H. (2014). An investigation of the effects of the core protein telomerase reverse transcriptase on Wnt signaling in breast cancer cells. Mol Cell Biol 34, 280–289. doi: 10.1128/MCB.00844-13
Listerman, I., Sun, J., Gazzaniga, F. S., Lukas, J. L., and Blackburn, E. H. (2013). The major reverse transcriptase-incompetent splice variant of the human telomerase protein inhibits telomerase activity but protects from apoptosis. Cancer research 73, 2817–2828. doi: 10.1158/0008-5472.CAN-12-3082
Liu, N., Ding, D., Hao, W., Yang, F., Wu, X., Wang, M., et al. (2016). hTERT promotes tumor angiogenesis by activating VEGF via interactions with the Sp1 transcription factor. Nucleic Acids Res 44, 8693–8703. doi: 10.1093/nar/gkw549
Maida, Y., and Masutomi, K. (2015). Telomerase reverse transcriptase moonlights: Therapeutic targets beyond telomerase. Cancer science 106, 1486–1492. doi: 10.1111/cas.12806
Maida, Y., Yasukawa, M., Okamoto, N., Ohka, S., Kinoshita, K., Totoki, Y., et al. (2014). Involvement of telomerase reverse transcriptase in heterochromatin maintenance. Mol Cell Biol 34, 1576–1593. doi: 10.1128/MCB.00093-14
Martinez, P., and Blasco, M. A. (2011). Telomeric and extra-telomeric roles for telomerase and the telomere-binding proteins. Nature reviews Cancer 11, 161–176. doi: 10.1038/nrc3025
Martínez, P., Gómez-López, G., García, F., Mercken, E., Mitchell, S., Flores, J. M., et al. (2013). RAP1 protects from obesity through its extratelomeric role regulating gene expression. Cell Rep 3, 2059–2074. doi: 10.1016/j.celrep.2013.05.030
Martinez, P., Thanasoula, M., Carlos, A. R., Gómez-López, G., Tejera, A. M., Schoeftner, S., et al. (2010). Mammalian Rap1 controls telomere function and gene expression through binding to telomeric and extratelomeric sites. Nat Cell Biol 12, 768–780. doi: 10.1038/ncb2081
Masutomi, K., Possemato, R., Wong, J. M., Currier, J. L., Tothova, Z., Manola, J. B., et al. (2005). The telomerase reverse transcriptase regulates chromatin state and DNA damage responses. Proceedings of the National Academy of Sciences of the United States of America 102, 8222–8227. doi: 10.1073/pnas.0503095102
Mattiussi, M., Tilman, G., Lenglez, S., and Decottignies, A. (2012). Human telomerase represses ROS-dependent cellular responses to Tumor Necrosis Factor-α without affecting NF-κB activation. Cell Signal 24, 708–717. doi: 10.1016/j.cellsig.2011.11.004
Michelini, F., Pitchiaya, S., Vitelli, V., Sharma, S., Gioia, U., Pessina, F., et al. (2017). Damage-induced lncRNAs control the DNA damage response through interaction with DDRNAs at individual double-strand breaks. Nat Cell Biol 19, 1400–1411. doi: 10.1038/ncb3643
Miwa, S., Czapiewski, R., Wan, T., Bell, A., Hill, K. N., von Zglinicki, T., et al. (2016). Decreased mTOR signalling reduces mitochondrial ROS in brain via accumulation of the telomerase protein TERT within mitochondria. Aging 8, 2551–2567. doi: 10.18632/aging.101089
Miwa, S., and Saretzki, G. (2017). Telomerase and mTOR in the brain: the mitochondria connection. Neural Regen Res 12, 358–361. doi: 10.4103/1673-5374.202922
Mukherjee, S., Firpo, E. J., Wang, Y., and Roberts, J. M. (2011). Separation of telomerase functions by reverse genetics. Proceedings of the National Academy of Sciences of the United States of America 108, E1363–E1371. doi: 10.1073/pnas.1112414108
Nelson, A. D., and Shippen, D. E. (2015). Evolution of TERT-interacting lncRNAs: expanding the regulatory landscape of telomerase. Front Genet 6:277. doi: 10.3389/fgene.2015.00277
Park, J. I., Venteicher, A. S., Hong, J. Y., Choi, J., Jun, S., Shkreli, M., et al. (2009). Telomerase modulates Wnt signalling by association with target gene chromatin. Nature 460, 66–72. doi: 10.1038/nature08137
Passos, J. F., Saretzki, G., and von Zglinicki, T. (2007). DNA damage in telomeres and mitochondria during cellular senescence: is there a connection? Nucleic Acids Res 35, 7505–7513. doi: 10.1093/nar/gkm893
Porro, A., Feuerhahn, S., Delafontaine, J., Riethman, H., Rougemont, J., and Lingner, J. (2014a). Functional characterization of the TERRA transcriptome at damaged telomeres. Nat Commun 5, 5379. doi: 10.1038/ncomms6379
Porro, A., Feuerhahn, S., and Lingner, J. (2014b). TERRA-reinforced association of LSD1 with MRE11 promotes processing of uncapped telomeres. Cell Rep 6, 765–776. doi: 10.1016/j.celrep.2014.01.022
Romaniuk, A., Paszel-Jaworska, A., Toton, E., Lisiak, N., Holysz, H., Krolak, A., et al. (2019). The non-canonical functions of telomerase: to turn off or not to turn off. Molecular biology reports 46, 1401–1411. doi: 10.1007/s11033-018-4496-x
Saeboe-Larssen, S., Fossberg, E., and Gaudernack, G. (2006). Characterization of novel alternative splicing sites in human telomerase reverse transcriptase (hTERT): analysis of expression and mutual correlation in mRNA isoforms from normal and tumour tissues. BMC Mol Biol 7:26. doi: 10.1186/1471-2199-7-26
Sahin, E., Colla, S., Liesa, M., Moslehi, J., Müller, F. L., Guo, M., et al. (2011). Telomere dysfunction induces metabolic and mitochondrial compromise. Nature 470, 359–365. doi: 10.1038/nature09787
Samy, M., Gattolliat, C. H., Pendino, F., Hillion, J., Nguyen, E., Bombard, S., et al. (2012). Loss of the malignant phenotype of human neuroblastoma cells by a catalytically inactive dominant-negative hTERT mutant. Molecular cancer therapeutics 11, 2384–2393. doi: 10.1158/1535-7163.MCT-12-0281
Santos, J. H., Meyer, J. N., Skorvaga, M., Annab, L. A., and Van Houten, B. (2004). Mitochondrial hTERT exacerbates free-radical-mediated mtDNA damage. Aging cell 3, 399–411. doi: 10.1111/j.1474-9728.2004.00124.x
Santos, J. H., Meyer, J. N., and Van Houten, B. (2006). Mitochondrial localization of telomerase as a determinant for hydrogen peroxide-induced mitochondrial DNA damage and apoptosis. Human molecular genetics 15, 1757–1768. doi: 10.1093/hmg/ddl098
Saretzki, G. (2014). Extra-telomeric functions of human telomerase: cancer, mitochondria and oxidative stress. Current pharmaceutical design 20, 6386–6403. doi: 10.2174/1381612820666140630095606
Sarin, K. Y., Cheung, P., Gilison, D., Lee, E., Tennen, R. I., Wang, E., et al. (2005). Conditional telomerase induction causes proliferation of hair follicle stem cells. Nature 436, 1048–1052. doi: 10.1038/nature03836
Sayed, M. E., Yuan, L., Robin, J. D., Tedone, E., Batten, K., Dahlson, N., et al. (2019). NOVA1 directs PTBP1 to hTERT pre-mRNA and promotes telomerase activity in cancer cells. Oncogene 38, 2937–2952. doi: 10.1038/s41388-018-0639-8
Schmidt, J. C., and Cech, T. R. (2015). Human telomerase: biogenesis, trafficking, recruitment, and activation. Genes & development 29, 1095–1105. doi: 10.1101/gad.263863.115
Sharma, N. K., Reyes, A., Green, P., Caron, M. J., Bonini, M. G., Gordon, D. M., et al. (2012). Human telomerase acts as a hTR-independent reverse transcriptase in mitochondria. Nucleic Acids Res 40, 712–725. doi: 10.1093/nar/gkr758
Shay, J. W., and Bacchetti, S. (1997). A survey of telomerase activity in human cancer. European journal of cancer 33, 787–791. doi: 10.1016/s0959-8049(97)00062-2
Shay, J. W., and Wright, W. E. (2019). Telomeres and telomerase: three decades of progress. Nature reviews Genetics 20, 299–309. doi: 10.1038/s41576-019-0099-1
Shkreli, M., Sarin, K. Y., Pech, M. F., Papeta, N., Chang, W., Brockman, S. A., et al. (2011). Reversible cell-cycle entry in adult kidney podocytes through regulated control of telomerase and Wnt signaling. Nature medicine 18, 111–119. doi: 10.1038/nm.2550
Singhapol, C., Pal, D., Czapiewski, R., Porika, M., Nelson, G., and Saretzki, G. C. (2013). Mitochondrial telomerase protects cancer cells from nuclear DNA damage and apoptosis. PLoS One 8:e52989. doi: 10.1371/journal.pone.0052989
Smith, L. L., Coller, H. A., and Roberts, J. M. (2003). Telomerase modulates expression of growth-controlling genes and enhances cell proliferation. Nature cell biology 5, 474–479. doi: 10.1038/ncb985
Soder, A. I., Going, J. J., Kaye, S. B., and Keith, W. N. (1998). Tumour specific regulation of telomerase RNA gene expression visualized by in situ hybridization. Oncogene 16, 979–983. doi: 10.1038/sj.onc.1201620
Soder, A. I., Hoare, S. F., Muir, S., Going, J. J., Parkinson, E. K., and Keith, W. N. (1997). Amplification, increased dosage and in situ expression of the telomerase RNA gene in human cancer. Oncogene 14, 1013–1021. doi: 10.1038/sj.onc.1201066
Spilsbury, A., Miwa, S., Attems, J., and Saretzki, G. (2015). The role of telomerase protein TERT in Alzheimer’s disease and in tau-related pathology in vitro. J Neurosci 35, 1659–1674. doi: 10.1523/JNEUROSCI.2925-14.2015
Stewart, S. A., Hahn, W. C., O’Connor, B. F., Banner, E. N., Lundberg, A. S., Modha, P., et al. (2002). Telomerase contributes to tumorigenesis by a telomere length-independent mechanism. Proceedings of the National Academy of Sciences of the United States of America 99, 12606–12611. doi: 10.1073/pnas.182407599
Strong, M. A., Vidal-Cardenas, S. L., Karim, B., Yu, H., Guo, N., and Greider, C. W. (2011). Phenotypes in mTERT−/− and mTERT−/− mice are due to short telomeres, not telomere-independent functions of telomerase reverse transcriptase. Mol Cell Biol 31, 2369–2379. doi: 10.1128/mcb.05312-11
Tardat, M., and Déjardin, J. (2018). Telomere chromatin establishment and its maintenance during mammalian development. Chromosoma 127, 3–18. doi: 10.1007/s00412-017-0656-3
Teichroeb, J. H., Kim, J., and Betts, D. H. (2016). The role of telomeres and telomerase reverse transcriptase isoforms in pluripotency induction and maintenance. RNA biology 13, 707–719. doi: 10.1080/15476286.2015.1134413
Trotter, K. W., and Archer, T. K. (2008). The BRG1 transcriptional coregulator. Nucl Recept Signal 6, e004. doi: 10.1621/nrs.06004
Ulaner, G. A., Hu, J. F., Vu, T. H., Giudice, L. C., and Hoffman, A. R. (1998). Telomerase activity in human development is regulated by human telomerase reverse transcriptase (hTERT) transcription and by alternate splicing of hTERT transcripts. Cancer research 58, 4168–4172.
Uziel, O., Reshef, H., Ravid, A., Fabian, I., Halperin, D., Ram, R., et al. (2008). Oxidative stress causes telomere damage in Fanconi anaemia cells - a possible predisposition for malignant transformation. British journal of haematology 142, 82–93. doi: 10.1111/j.1365-2141.2008.07137.x
Vogelstein, B., Lane, D., and Levine, A. J. (2000). Surfing the p53 network. Nature 408, 307–310. doi: 10.1038/35042675
Wick, M., Zubov, D., and Hagen, G. (1999). Genomic organization and promoter characterization of the gene encoding the human telomerase reverse transcriptase (hTERT). Gene 232, 97–106. doi: 10.1016/s0378-1119(99)00108-0
Wong, M. S., Wright, W. E., and Shay, J. W. (2014). Alternative splicing regulation of telomerase: a new paradigm? Trends in genetics : TIG 30, 430–438. doi: 10.1016/j.tig.2014.07.006
Wu, K. J., Grandori, C., Amacker, M., Simon-Vermot, N., Polack, A., Lingner, J., et al. (1999). Direct activation of TERT transcription by c-MYC. Nature genetics 21, 220–224. doi: 10.1038/6010
Wu, P., Takai, H., and de Lange, T. (2012). Telomeric 3’ overhangs derive from resection by Exo1 and Apollo and fill-in by POT1b-associated CST. Cell 150, 39–52. doi: 10.1016/j.cell.2012.05.026
Wu, Y. L., Dudognon, C., Nguyen, E., Hillion, J., Pendino, F., Tarkanyi, I., et al. (2006). Immunodetection of human telomerase reverse-transcriptase (hTERT) re-appraised: nucleolin and telomerase cross paths. Journal of cell science 119(Pt 13), 2797–2806. doi: 10.1242/jcs.03001
Xi, L., and Cech, T. R. (2014). Inventory of telomerase components in human cells reveals multiple subpopulations of hTR and hTERT. Nucleic Acids Res 42, 8565–8577. doi: 10.1093/nar/gku560
Yamaguchi, H., Baerlocher, G. M., Lansdorp, P. M., Chanock, S. J., Nunez, O., Sloand, E., et al. (2003). Mutations of the human telomerase RNA gene (TERC) in aplastic anemia and myelodysplastic syndrome. Blood 102, 916–918. doi: 10.1182/blood-2003-01-0335
Yang, D., Xiong, Y., Kim, H., He, Q., Li, Y., Chen, R., et al. (2011). Human telomeric proteins occupy selective interstitial sites. Cell Res 21, 1013–1027. doi: 10.1038/cr.2011.39
Yang, T. B., Chen, Q., Deng, J. T., Jagannathan, G., Tobias, J. W., Schultz, D. C., et al. (2017). Mutual reinforcement between telomere capping and canonical Wnt signalling in the intestinal stem cell niche. Nat Commun 8, 14766. doi: 10.1038/ncomms14766
Ye, J., Renault, V. M., Jamet, K., and Gilson, E. (2014). Transcriptional outcome of telomere signalling. Nat Rev Genet 15, 491–503. doi: 10.1038/nrg3743
Yeung, F., Ramírez, C. M., Mateos-Gomez, P. A., Pinzaru, A., Ceccarini, G., Kabir, S., et al. (2013). Nontelomeric role for Rap1 in regulating metabolism and protecting against obesity. Cell Rep 3, 1847–1856. doi: 10.1016/j.celrep.2013.05.032
Yi, X., Shay, J. W., and Wright, W. E. (2001). Quantitation of telomerase components and hTERT mRNA splicing patterns in immortal human cells. Nucleic Acids Res 29, 4818–4825. doi: 10.1093/nar/29.23.4818
Yin, L., Hubbard, A. K., and Giardina, C. (2000). NF-kappa B regulates transcription of the mouse telomerase catalytic subunit. The Journal of biological chemistry 275, 36671–36675. doi: 10.1074/jbc.m007378200
Young, J. I., Sedivy, J. M., and Smith, J. R. (2003). Telomerase expression in normal human fibroblasts stabilizes DNA 5-methylcytosine transferase I. The Journal of biological chemistry 278, 19904–19908. doi: 10.1074/jbc.m301685200
Yu, J., Yuan, X., Sjoholm, L., Liu, T., Kong, F., Ekstrom, T. J., et al. (2018). Telomerase reverse transcriptase regulates DNMT3B expression/aberrant DNA methylation phenotype and AKT activation in hepatocellular carcinoma. Cancer letters 434, 33–41. doi: 10.1016/j.canlet.2018.07.013
Yuan, X., and Xu, D. (2019). Telomerase Reverse Transcriptase (TERT) in Action: Cross-Talking with Epigenetics. International journal of molecular sciences 20, E3338. doi: 10.3390/ijms20133338
Zhou, J., Ding, D., Wang, M., and Cong, Y. S. (2014). Telomerase reverse transcriptase in the regulation of gene expression. BMB reports 47, 8–14. doi: 10.5483/bmbrep.2014.47.1.284
Zhou, L., Zheng, D., Wang, M., and Cong, Y. S. (2009). Telomerase reverse transcriptase activates the expression of vascular endothelial growth factor independent of telomerase activity. Biochemical and biophysical research communications 386, 739–743. doi: 10.1016/j.bbrc.2009.06.116
Keywords: telomere, telomerase (TERT), signaling pathway, stem cell function, mitochondria
Citation: Ségal-Bendirdjian E and Geli V (2019) Non-canonical Roles of Telomerase: Unraveling the Imbroglio. Front. Cell Dev. Biol. 7:332. doi: 10.3389/fcell.2019.00332
Received: 02 July 2019; Accepted: 27 November 2019;
Published: 10 December 2019.
Edited by:
Maria Luisa Cayuela, Hospital Universitario Virgen de la Arrixaca, SpainCopyright © 2019 Ségal-Bendirdjian and Geli. This is an open-access article distributed under the terms of the Creative Commons Attribution License (CC BY). The use, distribution or reproduction in other forums is permitted, provided the original author(s) and the copyright owner(s) are credited and that the original publication in this journal is cited, in accordance with accepted academic practice. No use, distribution or reproduction is permitted which does not comply with these terms.
*Correspondence: Evelyne Ségal-Bendirdjian, ZXZlbHluZS5zZWdhbC1iZW5kaXJkamlhbkBpbnNlcm0uZnI=; Vincent Geli, dmluY2VudC5nZWxpQGluc2VybS5mcg==
†These authors have contributed equally to this work
Disclaimer: All claims expressed in this article are solely those of the authors and do not necessarily represent those of their affiliated organizations, or those of the publisher, the editors and the reviewers. Any product that may be evaluated in this article or claim that may be made by its manufacturer is not guaranteed or endorsed by the publisher.
Research integrity at Frontiers
Learn more about the work of our research integrity team to safeguard the quality of each article we publish.