- Department of Biomedical Engineering, Virginia Commonwealth University, Richmond, VA, United States
Epithelial-Mesenchymal Transition (EMT) is a critical process in embryonic development in which epithelial cells undergo a transdifferentiation into mesenchymal cells. This process is essential for tissue patterning and organization, and it has also been implicated in a wide array of pathologies. While the intracellular signaling pathways that regulate EMT are well-understood, there is increasing evidence that the mechanical properties and composition of the extracellular matrix (ECM) also play a key role in regulating EMT. In turn, EMT drives changes in the mechanics and composition of the ECM, creating a feedback loop that is tightly regulated in healthy tissues, but is often dysregulated in disease. Here we present a review that summarizes our understanding of how ECM mechanics and composition regulate EMT, and how in turn EMT alters ECM mechanics and composition.
1. Introduction
Epithelial-Mesenchymal Transition (EMT) is a transdifferentiation process in which epithelial cells progressively lose key hallmarks of the phenotype, including strong cell-cell contacts, an apicobasal polarity, a cobblestone morphology, and collective migration. Loss of the epithelial phenotype corresponds with an acquisition of a mesenchymal one, with hallmarks including front-back polarity, independent migration, and an elongated cell shape. This process, along with the inverse process, Mesenchymal-Epithelial Transition, is absolutely critical to tissue patterning and organization in developing embryos. However, the past three decades have revealed that dysregulation of EMT is associated with a wide array of pathologies, including fibrotic diseases and cancer. Maintenance of the epithelial phenotype, and subsequent loss of the phenotype during EMT, are highly influenced and controlled by the surrounding extracellular matrix (ECM).
This review summarizes the myriad interactions between the ECM, epithelial polarization, cell migration, and EMT, and details the following progression of ECM/EMT interactions, illustrated in Figure 1: the composition of the basal lamina drives epithelial differentiation; during routine tissue remodeling, MMPs activate migration through structural changes to the ECM, release of bioactive fragments, and shedding of cell-bound receptors. Each of these events, intended to maintain healthy tissue, prevents an avenue for dysregulation. Conversion to a fibrillar matrix upregulates mesenchymal transcription factors and represses epithelial markers to drive EMT. In a feed-forward process, EMT in turn upregulates many of these fibrillar ECM components. In addition to presenting an ECM composition which facilitates the mesenchymal phenotype, fibrillar ECM also drives changes in tissue stiffness, which further add to epithelial repression and mesenchymal differentiation. Finally, a host of matricellular proteins modify the ECM response to further regulate EMT. Pathways involved in these processes are summarized in Figure 2.
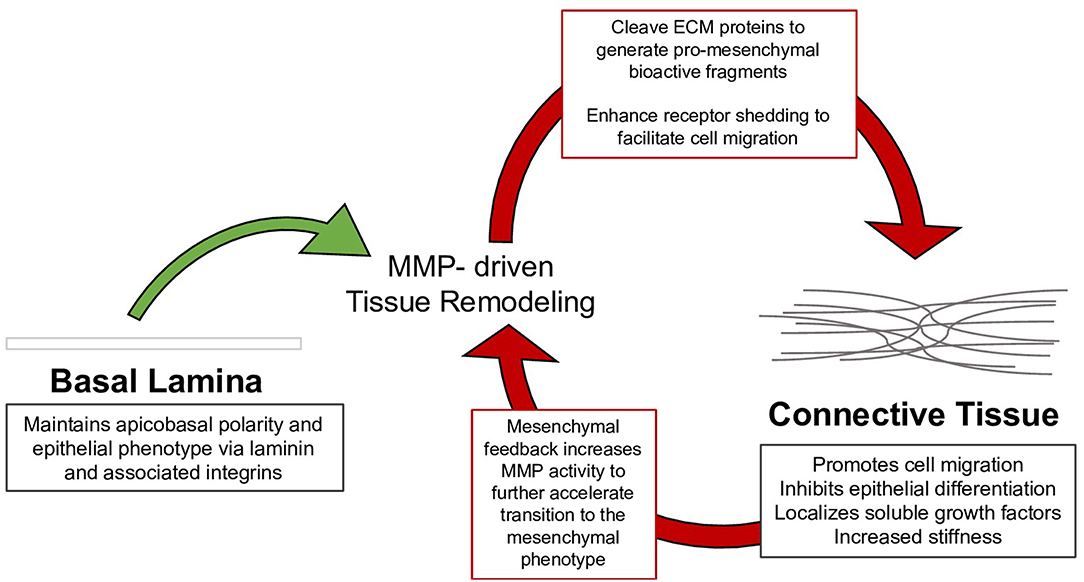
Figure 1. Tissue remodeling of the basal lamina is necessary to maintain healthy epithelium; however, mysregulation of this process drives the assembly of connective tissue, which can in turn facilitate the mesenchymal phenotype. The mesenchymal phenotype further drives tissue remodeling to continue the process in EMT.
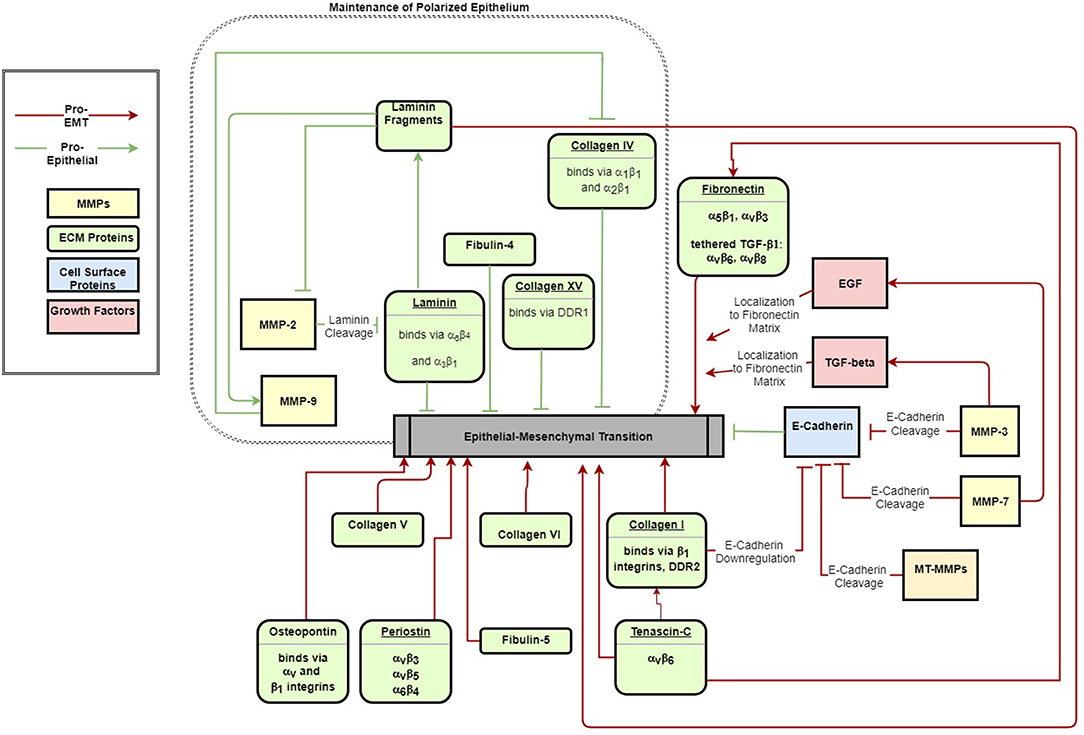
Figure 2. ECM signals both maintain epithelial polarization (green arrows) and drive EMT (red arrows). The schematic indicates major ECM components that have been identified to activate or inhibit EMT.
2. The Basal Lamina and Epithelial Differentiation
In a polarized epithelium, attachment to the ECM orients the apicobasal axis by defining the basal surface (Morrissey and Sherwood, 2015). Basement membranes are thin, dense specialized ECM that self-assemble at the basal surface of the epithelial sheet as a structural scaffolding for epithelial attachment (McKee et al., 2007). The innermost layers of the basement membrane are the cell-adherent layers, and include the laminin-rich lamina lucida and the collagen IV-rich lamina densa. Together these layers, joined by glycoprotein nidogen and heparan sulfate proteoglycans perlecan, agrin, and collagen VII, comprise a supramolecular, reticular structure known as the basal lamina (Hohenester and Yurchenco, 2013).
2.1. Basal Lamina Establishes the Epithelial Apicobasal Polarity
Laminins are a family of glycoproteins consisting of an α-β-γ heterotrimer that forms three separate short arms (laminin-αAβBγG) and an extended triple helical coiled-coil long arm arranged in a cross-like confirmation. Overlapping lateral interactions along the triple-helical domain and N- and C-terminal domains provides stability to the collagen IV network (Khoshnoodi et al., 2008). Collagen VII (Rousselle et al., 1997) and microfibril collagen VI (Yurchenco, 2011) stabilize the lamina densa and link it to the reticular lamina. Mammary epithelia exhibit normal physiological function when in contact with laminin, but lose this when exposed to fibrillar matrix components, such as collagen I and fibronectin. Developmental studies indicate that laminin not only maintains epithelial differentiation but is also the progenitor of the basement membrane and epithelial polarization during gastrulation (reviewed in Li et al., 2003), suggesting laminin contributes to epithelial differentiation rather than mesenchymal suppression (Zutter et al., 1995; Ramirez et al., 2011).
Laminins form a cell-adherent sheet-like matrix at the cell surface through interactions with its receptors. The laminin G domain located at the α-chain C-terminus of the long arm, together with adjacent coiled-coil domains, mediates cell attachment to integrin, dystroglycan, sulfated glycolipids, and heparan sulfate chains (Yurchenco and Patton, 2009). While laminin deposition and organization at the cell surface requires attachment to dystroglycan (Henry and Campbell, 1998; Henry et al., 2001) and β1 integrin (Li et al., 2002), assembly of laminin into sheets occurs spontaneously (Hamill et al., 2009). The laminin specific integrin receptors α3β1 and α6β4 additionally contribute to the integrity of the epithelium by reinforcing cell-cell junctions: integrin α3β1 is localized to the cytoplasmic plaque of cell-cell junctions, where it forms a complex with α-actinin and links the subcortical actin network to the catenin complex of cell-cell junctions (Wang et al., 1999); integrin α6β4 is localized to a multiprotein complex known as the hemidesmosome, which anchors the cytoskeleton to the basal lamina, provides attachment for intermediate filaments, and confers tensile strength to the epithelium (Borradori and Sonnenberg, 1999). Though not necessary for assembly, laminin-integrin ligation does stimulate small GTPases to restructure the cytoskeleton to support epithelial polarization (reviewed in Lee and Streuli, 2014). Integrin receptors for collagen and laminin were further shown to have a dynamic role in sensing the basal cue through distinct GTPase activity (Myllymäki et al., 2011): while α6β4 (along with collagen receptor α2β1) activates Rac-mediated actin cytoskeleton reorganization that guides apicobasal polarity, α3β1 stimulates Cdc42-mediated microtubule rearrangement, which guides polarity complexes to the respective apical and basolateral domain. These contacts, together with a basement membrane linked to connective tissue, form a cohesive and mechanically coupled structure.
2.2. Basal Lamina Components Regulate EMT
Despite its role in maintaining epithelial differentiation, elements of the basal lamina can also promote EMT and tumor growth. For example, laminin receptors α3β1 and α6β4 have been implicated in EMT and cancer progression. In a study of keratinocytes, α3β1 and α6β4 integrin ligation was sufficient to form tumors despite abnormal cell attachment (Waterman et al., 2007). Deletion of the α3β1 gene itga3 deletion prevented tumor initiation with an associated decrease in downstream activation of EMT-linked FAK, Rac1, MAPK, and JNK pathways (Cagnet et al., 2014). Studies of alveolar epithelium (Kim et al., 2009a,b) and hepatocellular carcinoma (Giannelli et al., 2005) demonstrate cooperative activity between α3β1 and transforming growth factor β1 (TGF-β1) to suppress the epithelial phenotype. Colocalization and endocytosis of α3β1 with the TGF-β receptor type I (TGFβRI) receptor led to phosphorylation of Smad2 and of β-catenin on Y654, resulting in formation of a pSmad2-Y654-β-catenin complex, though it is unclear how this complex suppresses the epithelial phenotype. A separate study in immortalized mouse keratinocytes demonstrated that α3β1-TGF-β1 cooperativity induces tissue remodeling of the basal lamina by stimulating MMP9 (Sugiura and Berditchevski, 1999; Morini et al., 2000) and induces epithelial transcriptional suppressors, Snail and Slug (Zhang et al., 2003). Hepatocellular carcinomas overexpressing α6β4 exhibit aberrant cell proliferation and invasion associated with downregulation of the epithelial phenotype by PI3K/Akt signaling dependent upregulation of Slug (Li et al., 2017).
In addition, collagen IV, which is a primary component of the basement membrane, can suppress epithelial differentiation and induce expression of the EMT transcription factors Snail and Slug. Hepatocellular carcinomas express collagen IV receptors α1β1 and α2β1 to facilitate local invasion across the basal lamina and lamina reticularis (Yang et al., 2003). In mammary epithelial cells, collagen IV induces epithelial repressors Snail and Slug by upstream FAK/ERK signaling and NFκB activation (Espinosa Neira and Salazar, 2012).
Another component of the basement membrane is Collagen XV, which forms an unusual supramolecular structure resembling a figure-of-eight/pretzel configuration (Myers et al., 2007). This structure links banded fibrils in the basement membrane (Amenta et al., 2005) to provide tensile strength and join the basement membrane to connective tissue. Collagen receptor discoidin domain receptor 1 (DDR1) is distributed to the lateral membrane of epithelium to stabilize cell-cell junctions. This suppresses Cdc42 activity (Wang et al., 2009) and prevents α2β1-DDR1 cooperative activation of migration. In pancreatic adenocarcinoma cells, collagen XV was shown to stabilize cell-cell junctions by DDR1, suggesting antagonistic EMT potential (Clementz et al., 2013).
3. Tissue Remodeling and EMT
Tissue remodeling in development (Brauer, 2006) and tissue repair (Page-McCaw et al., 2007; Rodríguez et al., 2010) requires fine spatiotemporal control over ECM degradation, which is often dysregulated in fibrosis (Giannandrea and Parks, 2014; Craig et al., 2015; Pardo et al., 2016) and cancer progression (Nabeshima et al., 2002; Têtu et al., 2006; Kessenbrock et al., 2010; Deryugina and Quigley, 2015). Through ECM and growth factor proteolysis, matrix metalloproteinases (MMPs) modify the molecular and mechanical characteristics of the extracellular microenvironment to facilitate cellular migration (Sternlicht and Werb, 2001). MMPs consist of a catalytic domain, an autoinhibitory prodomain, and hemopexin domain. The six classifications of MMPs–collagenase, gelatinase, stromelysin, matrilysin, membrane-type and non-classified MMPs–delineate substrate specificity that is further tied to cellular and extracellular localization (Nagase et al., 2006). Functionally, MMPs regulate motility motifs by proteolytically processing ECM components and its sequestered latent signals, as well as membrane receptor docking and shedding (Sternlicht and Werb, 2001). Receptor docking spatially confines remodeling, whereas proteolytic byproducts act as soluble signals to engage feedback loops that temporally maintain ECM degradation.
3.1. Growth Factors and Bioactive Fragments Activate EMT
Beyond the structural effects on ECM, MMP proteolytic processing of the basal lamina produces bioactive fragments (Horejs, 2016), many of which regulate angiogenesis (Xu et al., 2001) and migration (Horejs et al., 2014) in a paracrine fashion. For example: Collagen IV fragment α5(IV) binds collagen receptor DDR1, preventing distribution to cell-cell junctions, and activates ERK (Xiao et al., 2015), a downstream signal of TGF-β1-induced EMT (Xie et al., 2004; Buonato and Lazzara, 2014), and a laminin-111 β-chain fragment competitively binds α3β1 integrin to upregulate mesenchymal markers and switch gelatinase A (MMP2) production in the inner lamina lucida to gelatinase B (MMP9) in the outer lamina densa and reticular lamina.
Additionally, MMPs dock with cell adhesion receptors, facilitating proteolytic activation of latent signaling molecules sequestered within the ECM and inducing survival and migratory signaling pathways (Illman et al., 2006; Chaturvedi and Hass, 2011; Mori et al., 2013). Redistribution of MMPs to the migratory front mediates focal proteolysis that is spatially confined to the invadopodia (reviewed in Jacob and Prekeris, 2015): gelatinases (Yu and Stamenkovic, 2000), stromelysin (MMP3) (Maeda et al., 2002), and membrane type (MT)-MMPs (Mu et al., 2002) each proteolytically activate latent form of TGF-β1, and the subsequent TGF-β1 signaling upregulates gelatinases, creating a self-sustaining loop of matrix remodeling (Krstic and Santibanez, 2014). To this point, knockdown of MMP9 abrogated mesenchymal markers and inhibited TGF-β1-induced invasion and migration in a study of esophageal squamous cell carcinoma (Bai et al., 2017).
3.2. Receptor Shedding Enhances Mobility
Another means by which tissue remodeling drives EMT is through MMP-mediated receptor shedding. MT-MMP (Rozanov et al., 2004), MMP3 (Yamashita et al., 2011), MMP9, and matrilysin (MMP7) (McGuire et al., 2003) localize to adherens junctions to shed the E-cadherin ectodomain, producing a soluble fragment frequently increased in the serum of cancer patients (Repetto et al., 2014). The 80 kDa ectodomain fragment acts as a paracrine/autocrine signal that reduces cell aggregation by competitive homophilic binding with E-cadherin (Noe et al., 2001) and promotes MMP production via EGFR (David and Rajasekaran, 2012). MMP3 additionally cleaves E-cadherin, which specifically activates Rac1 splice variant Rac1b that in turn activates ROS/Snail (Radisky et al., 2005).
3.3. EMT Induces MMP Production
Soluble factors released during tissue remodeling can induce EMT, which in turn stimulates MMP production to sustain remodeling of the ECM for migration (reviewed in Gilles et al., 2013 and Bonnans et al., 2014). Activation of EMT transcription factors corresponds with an increased secretion of gelatinases in human mammary breast cancer epithelium (Octavio et al., 2015) and oral squamous cell carcinoma (Qiao et al., 2010). Specifically, Slug directly enhances MMP1 transcription in breast cancer cells (Shen et al., 2017a) as well as MMP9 in oral squamous cell carcinoma (Joseph et al., 2009), and Snail upregulates MMP9 in MDCKs (Jordà et al., 2005). Additionally, Kruppel-like factor 8 (KLF8), a downstream transcription factor of TGF-β1 signaling, upregulates MMP9 (Wang et al., 2011) and MT1-MMP, both directly (by activating MT1-MMP promoter) and indirectly (through β-catenin nuclear translocation and TCF1 upregulation) (Lu et al., 2014). Proteolytically activated growth factors induce these transcription factors, resulting in a positive feedback loop. TGF-β1-dependent EMT triggers gelatinases in oral squamous cell carcinoma by upregulated Snail (Qiao et al., 2010) and MMP10 in keratinocytes (Wilkins-Port and Higgins, 2007). Wnt stimulates MMP production that amplifies tissue remodeling and cell mobility. For example, MT3-MMP is a soluble and membrane bound proteinase that cleaves pro-MMP2 and is associated with progression of hepatocellular (Wu et al., 2007) and colorectal (Shen et al., 2017b) carcinomas.
4. Connective Tissue and Mesenchymal Differentiation
4.1. Fibrous Matrix Promotes Attachment and Migration
Unlike the basal lamina, the ECM of connective tissue is fibrillar and primarily deposited by activated fibroblasts (Hosper et al., 2013). Assembly of fibrillar matrix on the basement membrane plays a significant role in repressing the epithelial phenotype and inducing EMT. The primary component of connective tissue, fibrillar collagen, is crudely aligned parallel to the basal lamina to provide mechanical support by resisting tensile forces. Non-fibrillar collagens, proteoglycans, and glycoproteins organize the fibrillar matrix and link the connective tissue to the basement membrane. Fibronectin, a 230–270 kDa fibrous homodimer glycoprotein, provides a scaffold for cell attachment and de novo fibrillogenesis (Hynes, 1982). Secreted as a soluble dimer, maturation of the fibronectin matrix requires integrin attachment and cell contractility for polymerization into an insoluble fibrillar matrix (Mao and Schwarzbauer, 2005; Weinberg et al., 2017). Integrin binding facilitates fibronectin stretch, which exposes additional binding sites for ECM deposition and growth factor binding (Singh et al., 2010). As a scaffold for de novo ECM assembly, polymerized fibronectin provides binding sites for collagenous matrix deposition (Sottile et al., 2002). However, in a negative feedback loop that halts de novo ECM synthesis, maturation of collagenous matrix stabilizes polymerized fibronectin but shields it from cell contractility (Kubow et al., 2015).
Integrin and syndecan receptors link the fibronectin matrix to the cytoskeleton and signaling pathways during migration (Elfenbein and Simons, 2013). Integrins α5β1 and αvβ3 dynamically bind to the fibronectin matrix to extend pseudopodia and form contractile filaments via small GTPases (Morgan et al., 2009). Fibronectin binding organizes nascent cell contacts into stable focal adhesions that activate the conventional integrin signaling pathways associated with growth and motility. Antagonistic α5β1 recycling to the migratory front maintains cell-matrix adhesion whereas αvβ3 is recycled to the migratory front to form nascent focal adhesions to fibronectin and stimulate Rac-mediated cytoskeletal rearrangement (White et al., 2007; Lawson and Burridge, 2014). The sustained β1 integrin signaling stimulates Rho-mediated contractility as the nascent adhesion moves peripherally to centrally and matures to a fibrillar adhesion near the center and posterior of the cell. Syndecan, as a co-receptor for cell attachment, facilitates integrin clustering into focal adhesions (Woods et al., 2000); mediates small GTPase activity (Bass et al., 2007; Brooks et al., 2012); regulates extracellular matrix assembly (Klass et al., 2000); and, specifically, binds to the fibronectin type III12−14 heparin-binding domain adjacent to α5β1 to facilitate cell attachment (Tumova et al., 2000).
Collagens form the bulk of connective tissue, consisting of a family of 28 members classified as either fibril forming or network forming (Ricard-Blum, 2011). Three polypeptide α-chains of repeating gly-X-Y sequence and interrupting non-collagenous domains form a helical trimer, which is stabilized by glycine, proline and hydroxyproline, hydrogen bonds, and electrostatic interactions, allowing collagen to assemble unique structures and participate in biological activity (Shoulders and Raines, 2009). Collagen receptors β1 integrin heterodimers (α1, α2, α10, and α11) and DDRs relay adhesion signals at the cell-cell and cell-matrix interface to exert transcriptional regulation of migration and survival signaling pathways (Boraschi-Diaz et al., 2017). DDR1 stabilizes adherens junctions in the stable epithelium but redistributes to cell-matrix focal adhesions to regulate motility (Huang et al., 2009; Chen et al., 2016). Minor collagens, elastic fibers, and proteoglycans support fibrillar collagens to confer structural integrity to connective tissues (Frantz et al., 2010). Collagen V associates with fibrillar collagens I and III to regulate assembly, and collagen VI to stabilize the ECM (Mak et al., 2016). Collagen VI forms beaded filament networks at the stroma-basement membrane interface and organizes three-dimensional tissue architecture by linking connective tissue with collagen IV (Kuo et al., 1997) and laminin (Cescon et al., 2015) of the basal lamina.
Proteoglycans, owing largely to their glycosaminoglycan polysaccharide chains, hydrate tissues and provide mechanical support by resisting compression. Hyaluronan, a glycosaminoglycan synthesized by hyaluronan synthase (HAS), is secreted in a high molecular weight form consisting of N-acetylglucosamine and glucuronic acid repeats. The hyaluronan receptor CD44 activates Src, Rho, and Ras signaling pathways to alter cytoskeletal arrangement and promote motility (Turley et al., 2002). CD44 in particular regulates mobility by interacting with and stimulating production of MMPs. For example, hyaluronan-CD44 binding stimulates gelatinase secretion to regulate cancer invasion (Park et al., 2002; Zhang et al., 2002; Guo et al., 2012) and MMP9 docks with CD44 to remodel the ECM at the invasive front (Peng et al., 2007). Thus, hyaluronan and its receptors mediate mobility through connective tissue.
4.2. Fibrillar Matrix Inhibits Epithelial Differentiation
Dysregulation of fibrillar matrix is widely implicated in fibrosis (Karsdal et al., 2017) and cancer progression (Fang et al., 2014; Kaushik et al., 2016; Wang and Hielscher, 2017), owing to the suppression of epithelial differentiation (Shintani et al., 2008b). As a downstream target of Wnt (Gradl et al., 1999) and TGF-β1 (Kolosova et al., 2011) signaling, fibronectin is a marker of mesenchymal differentiation (Petrini et al., 2016). Fibronectin accumulation at cleft-forming sites during salivary gland and lung branching morphogenesis induces Slug to suppress the epithelial phenotype (Onodera et al., 2010). At the invadopodia, αvβ3 ligation induces Slug expression (Knowles et al., 2013) and α5β1 integrin stimulates Rho-mediated contractility (Mierke et al., 2011).
Fibrillar collagens, acting through canonical β1 integrin/FAK/Src signaling, suppress epithelial differentiation at the transcriptional level and disrupt the cadherin complex to enhance cell mobility (reviewed in Imamichi and Menke, 2007). In ovarian and prostate cancer cells, collagen-β1 binding alters E-cadherin expression through both PI3K- (Cheng and Leung, 2011) and Src-dependent mechanisms (Menke et al., 2001). Collagen I promotes Snail and LEF1 through ILK-dependent activation of NF-κB and inhibition of GSK3β, which drives transcriptional activation of Snail (Medici and Nawshad, 2010). In a separate study of pancreatic carcinoma cells, DDR1 and β1 integrin concomitant activation converges on JNK signaling to increase expression of N-cadherin (Shintani et al., 2008a), which is a marker of EMT. However, H-Ras-induced Zeb reduces DDR1 expression in mammary epithelial cells, suggesting a negative feedback loop during EMT (Koh et al., 2015).
Given its role in maintaining adherens junctions, collagen-DDR signaling may indicate a switch from cell-cell to cell-matrix adhesion. Switching from epithelial-associated to mesenchymal-associated DDR drives mesenchymal differentiation by activating and stabilizing EMT transcription factors Snail and Zeb, and by inducing gelatinases to promote invasion (reviewed in Rammal et al., 2016). Collagen I-DDR2 ligation induces invasion of metastatic mammary epithelium in vivo and in vitro by activating Src/ERK signaling to phosphorylate Snail, which facilitates stabilization and nuclear translocation (Zhang et al., 2013). Similarly, in human renal proximal tube epithelial cells, increased DDR2 expression by TGF-β1 suppresses epithelial differentiation via NF-κB and LEF-1 activation (Walsh et al., 2011). As a result, fibrillar collagens, specifically type I and III, are potent suppressors of epithelial differentiation, reducing cell aggregation by transcriptional regulation and disrupting junctions.
Reinforcing other collagenous components of connective tissue also regulates EMT. As a gene target of TGF-β1, aberrant collagen V expression is characteristic of fibrosis and cancer in various tissues (Mak et al., 2016). Overexpression of collagen V in idiopathic pulmonary fibrosis is driven via both a Twist-dependent mechanism (Lei et al., 2016) and STIM1, an endoplasmic reticulum Ca2+ sensor that regulates Ca2+ influx and promotes invasion of colorectal cancer (Zhang et al., 2015, 2017). Co-immunoprecipitation identified TGF-β1 and MMP2 as binding partners of collagen V (Symoens et al., 2011), suggesting collagen V sequesters TGF-β1 and MMP2 in the ECM to restrict bioavailability and exert spatiotemporal control over tissue remodeling. Collagen VI and its non-integrin receptor NG2, a transmembrane chondroitin sulfate proteoglycan, are highly expressed in tumors (reviewed in Chen et al., 2013) and at the invasive front (Park and Scherer, 2012) where they synergize with canonical TGF-β1 signaling to enhance EMT. NG2 stabilizes cytoplasmic β-catenin and phosphorylates GSK3β downstream of β1 integrin-induced Akt (Chekenya et al., 2008), resulting in activation of TCF/LEF and nuclear accumulation of β-catenin (Iyengar et al., 2005).
Hyaluronan is another key component of the connective tissue ECM that stimulates EMT. CD44 mediates hyaluronan-induced invasion through interactions with MMPs that result in focal proteolysis and CD44 cleavage: CD44 ligation localizes MMP9 to the migratory front where it remodels the ECM and cleaves CD44 (Chetty et al., 2012). MT1-MMP additionally localizes to invadopodia where it sheds the CD44 ectodomain to promote motility (Kajita et al., 2001). Aberrant expression of hyaluronan is observed in the tumor stroma of breast, lung, and prostate cancer; urine of bladder cancer; and serum of ovarian, head and neck, and prostate cancer(reviewed in Chanmee et al., 2016) where it primes the cell and microenvironment for invasion(reviewed in Toole, 2004). Hyaluronan induces LOX-mediated matrix stiffening, FAK/Erk signaling, and Twist downstream of CD44 in the progression of breast carcinoma (El-Haibi et al., 2012). In addition, downstream PI3K/Akt and GSK3β phosphorylation results in β-catenin nuclear translocation (Zoltan-Jones et al., 2003), suggesting hyaluronan regulates the epithelial phenotype by inhibiting the catenin complex formation. Despite accumulation in the tumor microenvironment and association with aggressiveness, neither hyaluronan nor CD44 are required to induce mesenchymal transcriptional promoters. Instead, hyaluronan synthase 2 (HAS2) regulates TGF-β1-induced EMT in normal murine mammary gland cells independent of hyaluronan synthesizing activity (Porsch et al., 2013). Knockdown of HAS2 resulted in decreased TGF-β1-induced migration, suggesting a potential role downstream of TGF-β1 signaling. Thus, hyaluronan, along with its synthase and CD44 receptor, not only create a microenvironment to support migration, but also actively suppress epithelial proteins to promote EMT.
4.3. Connective Tissue Regulates Soluble Factor Localization
Just as in the basal lamina, proteoglycans of connective tissue sequester soluble factors as a means to regulate bioavailability or to spatially confine activation. Neighboring the primary cell attachment domain of fibronectin, a growth factor binding domain localizes growth factor signaling near the cell attachment for simultaneous activation of signaling pathways that promote survival and migration (Vega and Schwarzbauer, 2016). One example of this in EMT is the latent TGF-β-binding protein (LTBP), which binds to fibronectin at the type III12-14 repeat and sequesters TGF-β1 in a conformationally latent form until mechanically or proteolytically activated (Zilberberg et al., 2012; Robertson et al., 2015). Confining TGF-β1 to the ECM in this latent form allows for spatial and temporal control over TGF-β1 activation (Rifkin, 2005). In the ECM-bound latent form, TGF-β1 is activated through cell contractility or proteolytic cleavage. The αv integrins, namely αvβ6 and αvβ8, bind to the RGD sequences in fibronectin and latent TGF-β complex LAP to conformationally alter LAP and activate TGF-β1 (Mamuya and Duncan, 2012).
Taken together, conventional integrin signaling and growth factor availability regulation represent two distinct but interacting mechanisms by which fibrillar ECM regulates EMT (Hynes, 2009). Previous studies of mammary breast epithelium suggest fibronectin, but not laminin, is necessary for TGF-β1-induced EMT, likely due to binding the fibronectin receptor α5β1 integrin and latent TGF-β1 localization (Park and Schwarzbauer, 2014; Griggs et al., 2017). Fibronectin receptor αvβ3 integrin has also been shown to phosphorylate TGFβRII at Y284 to activate p38/MAPK signaling, separately from canonical Smad signaling, and promote tumor invasion (Galliher and Schiemann, 2007).
4.4. Tissue Mechanics Inhibit Epithelial Differentiation
In addition to the compositional aspects of ECM-EMT regulation, the mechanical properties of the ECM play a key role in regulating epithelial differentiation and EMT. Prior studies have demonstrated that induction of EMT is dependent on the mechanical properties of the underlying tissue; in vitro, TGF-β induces EMT on surfaces with a high elastic modulus, but induces apoptosis on surfaces with a lower elastic modulus (Leight et al., 2012). Inherent tension within a tissue also induces EMT; areas of higher stress within a colony of epithelial cells correlates with EMT, while ares of lower stress maintain the epithelial phenotype (Gomez et al., 2010). As such, the mechanics of the ECM play a critical role in driving EMT.
Mechanical coupling between a cell and its environment allows for rapid signal transduction and propagation across the tissue. Mechanotransducers, the cell adhesion receptors and focal adhesion proteins that link the ECM to the cytoskeleton and intracellular signaling cascades, reorient the cytoskeleton to mitigate anisotropic tension (Tseng et al., 2012). Expression of the EMT marker vimentin, which is assembled into intermediate filaments, has been shown to enhance mechanotransduction (Conway and Schwartz, 2014) and mediate growth of focal adhesions (Liu et al., 2015); similarly, organization of the microtubule network regulates both contractile and propulsive force generation (Kent and Lele, 2016), which facilitates migration and protrusion during EMT (Whipple et al., 2010; Gu et al., 2016). Although connective tissue lacks the inherent organization of self-assembled basal lamina, tension pulls the fibers into alignment, parallel to the direction of applied force. The fibrillar components stiffen the ECM and shift cell adhesion and cytoskeletal arrangement toward a migratory scheme.
Deposition and organization of the ECM is sensitive to substrate stiffness (Eisenberg et al., 2011). Fibronectin assembly correlates with substrate stiffness (Williams et al., 2008; Scott et al., 2015), which is not the case with self-assembled laminin in basal lamina. A proposed mechanism suggests stretch of fibronectin type III repeats exposes additional growth factor and ECM binding sites (Weinberg et al., 2017). Stiffer matrices promote greater fibrillogenesis, which in turn facilitates excessive matrix deposition, growth factor tethering, and further stiffening of the ECM (Kubow et al., 2015). It has been proposed that one possible role for collagen in fibronectin deposition is to provide a rigid collagen network that increases tension in the matrix to facilitate fibronectin assembly (Singh et al., 2010). This mechanism would be similar to the tensional effects of cell-cell interactions on fibronectin assembly in Xenopus embryos (Dzamba et al., 2009).
Mechanical feedback at cell-matrix interfaces is an important regulator of EMT (Le Bras et al., 2012). In addition to matrix deposition, the effects of matrix stiffening may enhance signaling of tethered growth factors. In alveolar epithelial cells, fibronectin facilitates stiffness-dependent EMT induced by TGF-β. The requirement for integrin αv that binds both fibronectin and the TGF-β1 complex suggests cell contractility mediates the substrate stiffness response to TGF-β1-induced EMT (Markowski et al., 2012; Brown et al., 2013). The αv integrin activates latent TGF-β, which in turn induces LOX production (Sethi et al., 2011; Voloshenyuk et al., 2011), crosslinks collagen, and stiffens the ECM. Together, these findings suggest integrin receptors mediate the stiffness trends of fibronectin-TGF-β1 activation of EMT in a contractility-dependent manner. In NMuMG cells, matrix rigidity regulates the switch between TGF-β1 induced apoptosis and EMT via FAK/PI3K/Akt signaling (Leight et al., 2012). Additionally, ECM stiffness plays a role in nuclear signaling: nuclear localization of Twist is observed with increased substrate stiffness due to β1 integrin activation (Wei et al., 2015); Yes-associated protein (YAP) and transcriptional co-activator with PDZ binding motif (TAZ) are nuclear relays of matrix rigidity downstream of Rho GTPase (Dupont et al., 2011); and nuclear translocation of Hippo effectors YAP and TAZ regulate a RhoA-dependent feedforward mechanism (Calvo et al., 2013) of cell spreading that controls focal adhesion assembly (Nardone et al., 2017). Mechanical feedback also drives rearrangement of cytoskeletal components in EMT, which in turn, can drive EMT (reviewed in Sun et al., 2015).
Similarly, mechanical signaling facilitates events at the cell-cell junction. Cadherin complexes transduce tension between adjacent cells during early epithelialization (Huang et al., 2012). Adherens junctions recruit actin to reinforce adhesion on substrates of high traction stress and strain (Collins et al., 2017). Tensile forces that are exerted on cadherin complexes result in the unfolding of α-catenin to reveal cryptic vinculin-binding sites, which nucleate polymerization of new actin microfilaments (Yonemura et al., 2010). G-actin depletion from the cytoplasmic pool stimulates nuclear accumulation of myocardin-related transcription factor (MRTF)-A, a regulator of actin alignment, to promote cellular contraction (O'Connor et al., 2015). MRTF-A activity also regulates myogenic features (Seifert and Posern, 2017), and is a downstream mediator of TGF-β1-induced EMT (Gomez et al., 2010).
5. The Functional Role of ECM Matricellular Proteins
In addition to the specific ECM components discussed here, there are a host of non-structural matricellular proteins that play a role in EMT signaling. A functional role is observed both in the extracellular environment, by soluble signal sequestering and receptor binding, and the intracellular environment, by orchestrating cytoskeletal arrangement or activating mesenchymal transcriptional promoters. Matricellular proteins also are involved in the cellular mechanical response, as mechanosensor integrins are receptors for many of the proteins.
5.1. Osteopontin
Osteopontin is a 44 kDa aspartic acid rich, N-linked glycosylated phosphoprotein with widespread expression across tissues. The osteopontin receptors, integrins αv and β1, and CD44, stimulate tissue remodeling, inflammation, and biomineralization signaling pathways. Due to its role in tissue remodeling and inflammation, aberrant expression is frequently associated with fibrosis and cancer (reviewed in Zhao et al., 2018), owing to induction of the mesenchymal pathways PI3K and MAPK and canonical transcriptional regulators Twist, Snail, and Zeb (Kothari et al., 2016). Together, these suggest osteopontin potently elicits EMT, further supported by the interaction with TGF-β1 to activate fibroblasts for tissue remodeling.
5.2. SPARC
Secreted protein acidic and rich in cysteine (SPARC) is a glycoprotein consisting of Ca2+ binding domains and a disulfide, copper binding follistatin domain. SPARC influences collagen I and IV organization, and sequesters growth factors to inhibit receptor binding (reviewed in Murphy-Ullrich and Sage, 2014). Overexpression is observed in a number of cancers (Arnold and Brekken, 2009) and is accompanied by increased expression of mesenchymal markers. Epidermal melanocytes exhibit E-cadherin suppression as a result of increased expression of Snail (Robert et al., 2006) and Slug dependent on PI3K/Akt signaling (Fenouille et al., 2012).
5.3. Periostin
Periostin consists of four alternatively spliced isoforms that consist of a small cysteine-rich module, fasciclin-like domains, and a hydrophilic carboxy terminal. The fasciclin-like domains mediate cell adhesion, while the hydrophilic carboxy terminal binds ECM proteins, such as collagen, fibronectin, tenascin-C, and heparin. Periostin is both a marker and promoter of EMT, which contributes to the progression of a number of tumor types (Morra and Moch, 2011). Expression is regulated by EMT transcription factor Twist and growth factors TGF-β1, BMP-2, PDGF, bFGF, TNFα, IL-4, IL-13, angiotensin II, and oncostatin (González-González and Alonso, 2018). Studies indicate periostin elicits a pronounced EMT response by downregulating miR-381, a Twist and Snail repressor, via MAPK (Hu et al., 2017).
5.4. Tenascin
Tenascins are a four member family of ECM glycoproteins consisting of N-terminal heptad repeats, EGF-like repeats, fibronectin type III repeats, and a C-terminal fibrinogen-like globular domain (Hsia and Schwarzbauer, 2005). Tenascin-C assembles into hexamers that regulate integrin, proteoglycan, and immunoglobulin binding to the ECM, primarily through interactions with fibronectin. FAK phosphorylation and Src activity together with β-catenin nuclear localization suggest tenascin induces EMT by integrin binding (Yoshida et al., 2015). TGF-β1 stimulates significantly greater tenascin-C secretion for mesenchymal cells relative to epithelial cells via ERK/MAPK but not PI3K signaling (Maschler et al., 2004).
5.5. Fibulin
Fibulins are a five isoform family of alternatively spliced anaphylatoxin-like repeats, calcium binding EGF-like repeats, and a fibulin-type carboxyl terminus. Calcium ligation confers structural stability to fibulin and elasticity to the ECM. Fibulins assemble into microfibrils that bind with other components of the ECM. Fibulin-4 knockdown significantly decreased expression of E-cadherin and increased expression of N-cadherin, vimentin, Snail, Slug, and Twist. Endometrial fibroblasts also exhibited a significant decrease in vimentin and α-smooth muscle actin when co-cultured with fibulin-4 expressing endometrial epithelial cells (Wang et al., 2017). Fibulin-5 contains an RGD-motif, and mediates binding to integrin receptors α4β1, α5β1, α9β5, αvβ3, and αvβ5 (Yanagisawa et al., 2009). Fibulin-5 initiates EMT and is also a gene target of TGF-β1, indicated by Twist expression. In addition, fibulin-5 stimulated gelatinase proteolytic activity (Lee et al., 2008).
6. Commentary and Outlook
In this review, we have summarized work that highlights the important role of healthy epithelial ECM in maintaining epithelial polarization, while also investigating how fibrillar ECM drives EMT, with a focus on the roles of both soluble signals embedded in the ECM and the mechanical properties of the ECM. We have also discussed how the EMT process itself remodels the ECM to enable EMT progression.
While many aspects of the bidirectional regulation between the ECM and EMT signaling are well-understood, there are also key aspects of these signaling processes that remain to be fully elucidated. Although many individual pieces of these physiological puzzles have been studied in isolation, the emergent responses in vivo are inherently more complicated to understand. For example, which mechanochemical interactions that drive dynamics are critical for epithelial polarization and EMT, and which signaling pathways act in parallel and provide redundancy? Further, what are the mechanisms driving these individual interactions between mechanics and composition at the molecular and biophysical level? Additionally, what processes and features are cell type and/or species dependent, which is a critical question to understand ultimately what aspects of epithelial maintenance and EMT suppression can be critically translated? Studies that probe these questions represent the next steps forward in understanding the complex interactions between ECM and EMT.
Author Contributions
LS, SW, and CL collectively formulated the structure and content of the review paper, wrote sections of the manuscript, and collectively edited the final manuscript.
Conflict of Interest Statement
The authors declare that the research was conducted in the absence of any commercial or financial relationships that could be construed as a potential conflict of interest.
Acknowledgments
This work was supported through the National Institutes of Health/National Institute of General Medical Sciences via Grant R01-GM115678 (CL, SW) and R01-GM122855 (CL, SW, LS). The authors would like to acknowledge Nadiah Hassan for thoughtful and thorough reading and comments.
References
Amenta, P. S., Scivoletti, N. A., Newman, M. D., Sciancalepore, J. P., Li, D., and Myers, J. C. (2005). Proteoglycan-collagen XV in human tissues is seen linking banded collagen fibers subjacent to the basement membrane. J. Histochem. Cytochem. 53, 165–176. doi: 10.1369/jhc.4A6376.2005
Arnold, S. A., and Brekken, R. A. (2009). SPARC: a matricellular regulator of tumorigenesis. J. Cell Commun. Signal. 3, 255–273. doi: 10.1007/s12079-009-0072-4
Bai, X., Li, Y.-y., Zhang, H.-y., Wang, F., He, H.-l., Yao, J.-c., et al. (2017). Role of matrix metalloproteinase-9 in transforming growth factor-β1-induced epithelial-mesenchymal transition in esophageal squamous cell carcinoma. Oncotargets Ther. 10, 2837–2847. doi: 10.2147/OTT.S134813
Bass, M. D., Roach, K. A., Morgan, M. R., Mostafavi-Pour, Z., Schoen, T., Muramatsu, T., et al. (2007). Syndecan-4'dependent Rac1 regulation determines directional migration in response to the extracellular matrix. J. Cell Biol. 177, 527–538. doi: 10.1083/jcb.200610076
Bonnans, C., Chou, J., and Werb, Z. (2014). Remodelling the extracellular matrix in development and disease. Nat. Rev. Mol. Cell Biol. 15, 786–801. doi: 10.1038/nrm3904
Boraschi-Diaz, I., Wang, J., Mort, J. S., and Komarova, S. V. (2017). Collagen type I as a ligand for receptor-mediated signaling. Front. Phys. 5:12. doi: 10.3389/fphy.2017.00012
Borradori, L., and Sonnenberg, A. (1999). Structure and function of hemidesmosomes: more than simple adhesion complexes. J. Invest. Dermatol. 112, 411–418. doi: 10.1046/j.1523-1747.1999.00546.x
Brauer, P. R. (2006). MMPs–role in cardiovascular development and disease. Front. Biosci. J. Virt. Libr. 11, 447–478. doi: 10.2741/1810
Brooks, R., Williamson, R. C., and Bass, M. D. (2012). Syndecan-4 independently regulates multiple small GTPases to promote fibroblast migration during wound healing. Small GTPases 3, 73–79. doi: 10.4161/sgtp.19301
Brown, A. C., Fiore, V. F., Sulchek, T. A., and Barker, T. H. (2013). Physical and chemical microenvironmental cues orthogonally control the degree and duration of fibrosis-associated epithelial-to-mesenchymal transitions. J. Pathol. 229, 25–35. doi: 10.1002/path.4114
Buonato, J. M., and Lazzara, M. J. (2014). ERK1/2 blockade prevents epithelial–mesenchymal transition in lung cancer cells and promotes their sensitivity to EGFR inhibition. Cancer Res. 74, 309–319. doi: 10.1158/0008-5472.CAN-12-4721
Cagnet, S., Faraldo, M. M., Kreft, M., Sonnenberg, A., Raymond, K., and Glukhova, M. A. (2014). Signaling events mediated by α3β1 integrin are essential for mammary tumorigenesis. Oncogene 33, 4286–4295. doi: 10.1038/onc.2013.391
Calvo, F., Ege, N., Grande-Garcia, A., Hooper, S., Jenkins, R. P., Chaudhry, S. I., et al. (2013). Mechanotransduction and YAP-dependent matrix remodelling is required for the generation and maintenance of cancer-associated fibroblasts. Nat. Cell Biol. 15, 637–646. doi: 10.1038/ncb2756
Cescon, M., Gattazzo, F., Chen, P., and Bonaldo, P. (2015). Collagen VI at a glance. J. Cell Sci. 128, 3525–3531. doi: 10.1242/jcs.169748
Chanmee, T., Ontong, P., and Itano, N. (2016). Hyaluronan: a modulator of the tumor microenvironment. Cancer Lett. 375, 20–30. doi: 10.1016/j.canlet.2016.02.031
Chaturvedi, S., and Hass, R. (2011). Extracellular signals in young and aging breast epithelial cells and possible connections to age-associated breast cancer development. Mech. Ageing Dev. 132, 213–219. doi: 10.1016/j.mad.2011.04.002
Chekenya, M., Krakstad, C., Svendsen, A., Netland, I. A., Staalesen, V., Tysnes, B. B., et al. (2008). The progenitor cell marker NG2/MPG promotes chemoresistance by activation of integrin-dependent PI3k/Akt signaling. Oncogene 27, 5182–5194. doi: 10.1038/onc.2008.157
Chen, H.-R., Yeh, Y.-C., Liu, C.-Y., Wu, Y.-T., Lo, F.-Y., Tang, M.-J., et al. (2016). DDR1 promotes E-cadherin stability via inhibition of integrin-β1-Src activation-mediated E-cadherin endocytosis. Sci. Rep. 6:36336. doi: 10.1038/srep36336
Chen, P., Cescon, M., and Bonaldo, P. (2013). Collagen VI in cancer and its biological mechanisms. Trends Mol. Med. 19, 410–417. doi: 10.1016/j.molmed.2013.04.001
Cheng, J.-C., and Leung, P. C. K. (2011). Type I collagen down-regulates E-cadherin expression by increasing PI3kca in cancer cells. Cancer Lett. 304, 107–116. doi: 10.1016/j.canlet.2011.02.008
Chetty, C., Vanamala, S. K., Gondi, C. S., Dinh, D. H., Gujrati, M., and Rao, J. S. (2012). MMP-9 induces CD44 cleavage and CD44 mediated cell migration in glioblastoma xenograft cells. Cell. Signal. 24, 549–559. doi: 10.1016/j.cellsig.2011.10.008
Clementz, A. G., Mutolo, M. J., Leir, S.-H., Morris, K. J., Kucybala, K., Harris, H., et al. (2013). Collagen XV inhibits epithelial to mesenchymal transition in pancreatic adenocarcinoma cells. PLoS ONE 8:e72250. doi: 10.1371/journal.pone.0072250
Collins, C., Denisin, A. K., Pruitt, B. L., and Nelson, W. J. (2017). Changes in E-cadherin rigidity sensing regulate cell adhesion. Proc. Natl. Acad. Sci. U.S.A. 114, E5835–E5844. doi: 10.1073/pnas.1618676114
Conway, D. E., and Schwartz, M. A. (2014). Mechanotransduction of shear stress occurs through changes in VE-cadherin and PECAM-1 tension: implications for cell migration. Cell Adhes. Migr. 9, 335–339. doi: 10.4161/19336918.2014.968498
Craig, V. J., Zhang, L., Hagood, J. S., and Owen, C. A. (2015). Matrix metalloproteinases as therapeutic targets for idiopathic pulmonary fibrosis. Am. J. Respir. Cell Mol. Biol. 53, 585–600. doi: 10.1165/rcmb.2015-0020TR
David, J. M., and Rajasekaran, A. K. (2012). Dishonorable discharge: the oncogenic roles of cleaved E-cadherin fragments. Cancer Res. 72, 2917–2923. doi: 10.1158/0008-5472.CAN-11-3498
Deryugina, E. I., and Quigley, J. P. (2015). Tumor angiogenesis: MMP-mediated induction of intravasation- and metastasis-sustaining neovasculature. Matrix Biol. 44–46, 94–112. doi: 10.1016/j.matbio.2015.04.004
Dupont, S., Morsut, L., Aragona, M., Enzo, E., Giulitti, S., Cordenonsi, M., et al. (2011). Role of YAP/TAZ in mechanotransduction. Nature 474, 179–183. doi: 10.1038/nature10137
Dzamba, B. J., Jakab, K. R., Marsden, M., Schwartz, M. A., and DeSimone, D. W. (2009). Cadherin adhesion, tissue tension, and noncanonical Wnt signaling regulate fibronectin matrix organization. Dev Cell 16, 421–432. doi: 10.1016/j.devcel.2009.01.008
Eisenberg, J. L., Safi, A., Wei, X., Espinosa, H. D., Budinger, G. S., Takawira, D., et al. (2011). Substrate stiffness regulates extracellular matrix deposition by alveolar epithelial cells. Res. Rep. Biol. 2011, 1–12. doi: 10.2147/RRB.S13178
Elfenbein, A., and Simons, M. (2013). Syndecan-4 signaling at a glance. J. Cell Sci. 126, 3799–3804. doi: 10.1242/jcs.124636
El-Haibi, C. P., Bell, G. W., Zhang, J., Collmann, A. Y., Wood, D., Scherber, C. M., et al. (2012). Critical role for lysyl oxidase in mesenchymal stem cell-driven breast cancer malignancy. Proc. Natl. Acad. Sci. U.S.A. 109, 17460–17465. doi: 10.1073/pnas.1206653109
Espinosa Neira, R., and Salazar, E. P. (2012). Native type IV collagen induces an epithelial to mesenchymal transition-like process in mammary epithelial cells MCF10a. Int. J. Biochem. Cell Biol. 44, 2194–2203. doi: 10.1016/j.biocel.2012.08.018
Fang, M., Yuan, J., Peng, C., and Li, Y. (2014). Collagen as a double-edged sword in tumor progression. Tumour Biol. 35, 2871–2882. doi: 10.1007/s13277-013-1511-7
Fenouille, N., Tichet, M., Dufies, M., Pottier, A., Mogha, A., Soo, J. K., et al. (2012). The epithelial-mesenchymal transition (EMT) regulatory factor SLUG (SNAI2) is a downstream target of SPARC and AKT in promoting melanoma cell invasion. PLoS ONE 7:e40378. doi: 10.1371/journal.pone.0040378
Frantz, C., Stewart, K. M., and Weaver, V. M. (2010). The extracellular matrix at a glance. J. Cell Sci. 123, 4195–4200. doi: 10.1242/jcs.023820
Galliher, A. J., and Schiemann, W. P. (2007). Src phosphorylates Tyr284 in TGF-beta type II receptor and regulates TGF-beta stimulation of p38 MAPK during breast cancer cell proliferation and invasion. Cancer Res. 67, 3752–3758. doi: 10.1158/0008-5472.CAN-06-3851
Giannandrea, M., and Parks, W. C. (2014). Diverse functions of matrix metalloproteinases during fibrosis. Dis. Models Mech. 7, 193–203. doi: 10.1242/dmm.012062
Giannelli, G., Bergamini, C., Fransvea, E., Sgarra, C., and Antonaci, S. (2005). Laminin-5 with transforming growth factor-β1 induces epithelial to mesenchymal transition in hepatocellular carcinoma. Gastroenterology 129, 1375–1383. doi: 10.1053/j.gastro.2005.09.055
Gilles, C., Newgreen, D. F., Sato, H., and Thompson, E. W. (2013). Matrix Metalloproteases and Epithelial-to-Mesenchymal Transition: Implications for Carcinoma Metastasis. Austin, TX: Landes Bioscience.
Gomez, E. W., Chen, Q. K., Gjorevski, N., and Nelson, C. M. (2010). Tissue geometry patterns epithelial-mesenchymal transition via intercellular mechanotransduction. J. Cell. Biochem. 110, 44–51. doi: 10.1002/jcb.22545
González-González, L., and Alonso, J. (2018). Periostin: a matricellular protein with multiple functions in cancer development and progression. Front. Oncol. 8:225. doi: 10.3389/fonc.2018.00225
Gradl, D., Kühl, M., and Wedlich, D. (1999). The Wnt/Wg signal transducer β-Catenin controls fibronectin expression. Mol. Cell. Biol. 19, 5576–5587. doi: 10.1128/MCB.19.8.5576
Griggs, L. A., Hassan, N. T., Malik, R. S., Griffin, B. P., Martinez, B. A., Elmore, L. W., et al. (2017). Fibronectin fibrils regulate TGF-β1-induced epithelial-mesenchymal transition. Matrix Biol. 60–61, 157–175. doi: 10.1016/j.matbio.2017.01.001
Gu, S., Liu, Y., Zhu, B., Ding, K., Yao, T.-P., Chen, F., et al. (2016). Loss of α-tubulin acetylation is associated with TGF-β-induced epithelial-mesenchymal transition. J. Biol. Chem. 291, 5396–5405. doi: 10.1074/jbc.M115.713123
Guo, M.-S., Wu, Y.-Y., and Liang, Z.-B. (2012). Hyaluronic acid increases MMP-2 and MMP-9 expressions in cultured trabecular meshwork cells from patients with primary open-angle glaucoma. Mol. Vis. 18, 1175–1181. doi: 10.1167/iovs.13-13088
Hamill, K. J., Kligys, K., Hopkinson, S. B., and Jones, J. C. R. (2009). Laminin deposition in the extracellular matrix: a complex picture emerges. J. Cell Sci. 122, 4409–4417. doi: 10.1242/jcs.041095
Henry, M. D., and Campbell, K. P. (1998). A role for dystroglycan in basement membrane assembly. Cell 95, 859–870. doi: 10.1016/S0092-8674(00)81708-0
Henry, M. D., Satz, J. S., Brakebusch, C., Costell, M., Gustafsson, E., Fassler, R., et al. (2001). Distinct roles for dystroglycan, (β)1 integrin and perlecan in cell surface laminin organization. J. Cell Sci. 114, 1137–1144.
Hohenester, E., and Yurchenco, P. D. (2013). Laminins in basement membrane assembly. Cell Adhes. Migr. 7, 56–63. doi: 10.4161/cam.21831
Horejs, C.-M. (2016). Basement membrane fragments in the context of the epithelial-to-mesenchymal transition. Eur. J. Cell Biol. 95, 427–440. doi: 10.1016/j.ejcb.2016.06.002
Horejs, C.-M., Serio, A., Purvis, A., Gormley, A. J., Bertazzo, S., Poliniewicz, A., et al. (2014). Biologically-active laminin-111 fragment that modulates the epithelial-to-mesenchymal transition in embryonic stem cells. Proc. Natl. Acad. Sci. U.S.A. 111, 5908–5913. doi: 10.1073/pnas.1403139111
Hosper, N. A., van den Berg, P. P., de Rond, S., Popa, E. R., Wilmer, M. J., Masereeuw, R., et al. (2013). Epithelial-to-mesenchymal transition in fibrosis: Collagen type I expression is highly upregulated after EMT, but does not contribute to collagen deposition. Exp. Cell Res. 319, 3000–3009. doi: 10.1016/j.yexcr.2013.07.014
Hsia, H. C., and Schwarzbauer, J. E. (2005). Meet the Tenascins: multifunctional and mysterious. J. Biol. Chem. 280, 26641–26644. doi: 10.1074/jbc.R500005200
Hu, W.-W., Chen, P.-C., Chen, J.-M., Wu, Y.-M., Liu, P.-Y., Lu, C.-H., et al. (2017). Periostin promotes epithelial-mesenchymal transition via the MAPK/miR-381 axis in lung cancer. Oncotarget 8, 62248–62260. doi: 10.18632/oncotarget.19273
Huang, R. Y.-J., Guilford, P., and Thiery, J. P. (2012). Early events in cell adhesion and polarity during epithelial-mesenchymal transition. J. Cell Sci. 125, 4417–4422. doi: 10.1242/jcs.099697
Huang, Y., Arora, P., McCulloch, C. A., and Vogel, W. F. (2009). The collagen receptor DDR1 regulates cell spreading and motility by associating with myosin IIA. J. Cell Sci. 122, 1637–1646. doi: 10.1242/jcs.046219
Hynes, R. O. (1982). Fibronectins: multifunctional modular glycoproteins. J. Cell Biol. 95, 369–377. doi: 10.1083/jcb.95.2.369
Hynes, R. O. (2009). Extracellular matrix: not just pretty fibrils. Science 326, 1216–1219. doi: 10.1126/science.1176009
Illman, S. A., Lehti, K., Keski-Oja, J., and Lohi, J. (2006). Epilysin (MMP-28) induces TGF-β mediated epithelial to mesenchymal transition in lung carcinoma cells. J. Cell Sci. 119, 3856–3865. doi: 10.1242/jcs.03157
Imamichi, Y., and Menke, A. (2007). Signaling pathways involved in collagen-induced disruption of the E-cadherin complex during epithelial-mesenchymal transition. Cells Tissues Organs 185, 180–190. doi: 10.1159/000101319
Iyengar, P., Espina, V., Williams, T. W., Lin, Y., Berry, D., Jelicks, L. A., et al. (2005). Adipocyte-derived collagen VI affects early mammary tumor progression in vivo, demonstrating a critical interaction in the tumor/stroma microenvironment. J. Clin. Invest. 115, 1163–1176. doi: 10.1172/JCI200523424
Jacob, A., and Prekeris, R. (2015). The regulation of MMP targeting to invadopodia during cancer metastasis. Front. Cell Dev. Biol. 3:4. doi: 10.3389/fcell.2015.00004
Jordà, M., Olmeda, D., Vinyals, A., Valero, E., Cubillo, E., Llorens, A., et al. (2005). Upregulation of MMP-9 in MDCK epithelial cell line in response to expression of the Snail transcription factor. J. Cell Sci. 118, 3371–3385. doi: 10.1242/jcs.02465
Joseph, M. J., Dangi-Garimella, S., Shields, M. A., Diamond, M. E., Sun, L., Koblinski, J. E., et al. (2009). Slug is a downstream mediator of transforming growth factor-β1-induced matrix metalloproteinase-9 expression and invasion of oral cancer cells. J. Cell. Biochem. 108, 726–736. doi: 10.1002/jcb.22309
Kajita, M., Itoh, Y., Chiba, T., Mori, H., Okada, A., Kinoh, H., et al. (2001). Membrane-type 1 matrix metalloproteinase cleaves Cd44 and promotes cell migration. J. Cell Biol. 153, 893–904. doi: 10.1083/jcb.153.5.893
Karsdal, M. A., Nielsen, S. H., Leeming, D. J., Langholm, L. L., Nielsen, M. J., Manon-Jensen, T., et al. (2017). The good and the bad collagens of fibrosis–their role in signaling and organ function. Adv. Drug Deliv. Rev. 121, 43–56. doi: 10.1016/j.addr.2017.07.014
Kaushik, S., Pickup, M. W., and Weaver, V. M. (2016). From transformation to metastasis: deconstructing the extracellular matrix in breast cancer. Cancer Metastasis Rev. 35, 655–667. doi: 10.1007/s10555-016-9650-0
Kent, I. A., and Lele, T. P. (2016). Microtubule-based force generation. Wiley Interdiscip. Rev. Nanomed. Nanobiotechnol. 9, 1–11. doi: 10.1002/wnan.1428
Kessenbrock, K., Plaks, V., and Werb, Z. (2010). Matrix metalloproteinases: regulators of the tumor microenvironment. Cell 141, 52–67. doi: 10.1016/j.cell.2010.03.015
Khoshnoodi, J., Pedchenko, V., and Hudson, B. (2008). Mammalian collagen IV. Microsc. Res. Tech. 71, 357–370. doi: 10.1002/jemt.20564
Kim, K. K., Wei, Y., Szekeres, C., Kugler, M. C., Wolters, P. J., Hill, M. L., et al. (2009a). Epithelial cell α3β1 integrin links β-catenin and Smad signaling to promote myofibroblast formation and pulmonary fibrosis. J. Clin. Invest. 119, 213–224. doi: 10.1172/JCI36940
Kim, Y., Kugler, M. C., Wei, Y., Kim, K. K., Li, X., Brumwell, A. N., et al. (2009b). Integrin α3β1-dependent β-catenin phosphorylation links epithelial Smad signaling to cell contacts. J. Cell Biol. 184, 309–322. doi: 10.1083/jcb.200806067
Klass, C. M., Couchman, J. R., and Woods, A. (2000). Control of extracellular matrix assembly by syndecan-2 proteoglycan. J. Cell Sci. 113, 493–506.
Knowles, L. M., Gurski, L. A., Engel, C., Gnarra, J. R., Maranchie, J. K., and Pilch, J. (2013). Integrin αvβ3 and fibronectin upregulate Slug in cancer cells to promote clot invasion and metastasis. Cancer Res. 73, 6175–6184. doi: 10.1158/0008-5472.CAN-13-0602
Koh, M., Woo, Y., Valiathan, R. R., Jung, H. Y., Park, S. Y., Kim, Y. N., et al. (2015). Discoidin domain receptor 1 is a novel transcriptional target of ZEB1 in breast epithelial cells undergoing H-Ras-induced epithelial to mesenchymal transition. Int. J. Cancer 136, E508–E520. doi: 10.1002/ijc.29154
Kolosova, I., Nethery, D., and Kern, J. A. (2011). Role of Smad2/3 and p38 MAP kinase in TGF-β1-induced epithelial-mesenchymal transition of pulmonary epithelial cells. J. Cell. Physiol. 226, 1248–1254. doi: 10.1002/jcp.22448
Kothari, A. N., Arffa, M. L., Chang, V., Blackwell, R. H., Syn, W.-K., Zhang, J., et al. (2016). Osteopontin–a master regulator of epithelial-mesenchymal transition. J. Clin. Med. 5:39. doi: 10.3390/jcm5040039
Krstic, J., and Santibanez, J. F. (2014). Transforming growth factor-beta and matrix metalloproteinases: functional interactions in tumor stroma-infiltrating myeloid cells. ScientificWorldJournal 2014:521754. doi: 10.1155/2014/521754
Kubow, K. E., Vukmirovic, R., Zhe, L., Klotzsch, E., Smith, M. L., Gourdon, D., et al. (2015). Mechanical forces regulate the interactions of fibronectin and collagen I in extracellular matrix. Nat. Commun. 6:8026. doi: 10.1038/ncomms9026
Kuo, H.-J., Maslen, C. L., Keene, D. R., and Glanville, R. W. (1997). Type VI collagen anchors endothelial basement membranes by interacting with type IV collagen. J. Biol. Chem. 272, 26522–26529. doi: 10.1074/jbc.272.42.26522
Lawson, C. D., and Burridge, K. (2014). The on-off relationship of Rho and Rac during integrin-mediated adhesion and cell migration. Small GTPases 5:e27958. doi: 10.4161/sgtp.27958
Le Bras, G. F., Taubenslag, K. J., and Andl, C. D. (2012). The regulation of cell-cell adhesion during epithelial-mesenchymal transition, motility and tumor progression. Cell Adhes. Migr. 6, 365–373. doi: 10.4161/cam.21326
Lee, J. L., and Streuli, C. H. (2014). Integrins and epithelial cell polarity. J. Cell Sci. 127, 3217–3225. doi: 10.1242/jcs.146142
Lee, Y.-H., Albig, A. R., Regner, M., Schiemann, B. J., and Schiemann, W. P. (2008). Fibulin-5 initiates epithelial-mesenchymal transition (EMT) and enhances EMT induced by TGF-β in mammary epithelial cells via a MMP-dependent mechanism. Carcinogenesis 29, 2243–2251. doi: 10.1093/carcin/bgn199
Lei, G.-S., Kline, H. L., Lee, C.-H., Wilkes, D. S., and Zhang, C. (2016). Regulation of collagen V expression and epithelial-mesenchymal transition by miR-185 and miR-186 during idiopathic pulmonary fibrosis. Am. J. Pathol. 186, 2310–2316. doi: 10.1016/j.ajpath.2016.04.015
Leight, J. L., Wozniak, M. A., Chen, S., Lynch, M. L., Chen, C. S., and Wang, Y.-L. (2012). Matrix rigidity regulates a switch between TGF-β1-induced apoptosis and epithelial-mesenchymal transition. Mol. Biol. Cell 23, 781–791. doi: 10.1091/mbc.e11-06-0537
Li, S., Edgar, D., Fässler, R., Wadsworth, W., and Yurchenco, P. D. (2003). The role of laminin in embryonic cell polarization and tissue organization. Dev. Cell 4, 613–624. doi: 10.1016/S1534-5807(03)00128-X
Li, S., Harrison, D., Carbonetto, S., Fässler, R., Smyth, N., Edgar, D., et al. (2002). Matrix assembly, regulation, and survival functions of laminin and its receptors in embryonic stem cell differentiation. J. Cell Biol. 157, 1279–1290. doi: 10.1083/jcb.200203073
Li, X.-L., Liu, L., Li, D.-D., He, Y.-P., Guo, L.-H., Sun, L.-P., et al. (2017). Integrin β4 promotes cell invasion and epithelial-mesenchymal transition through the modulation of Slug expression in hepatocellular carcinoma. Sci. Rep. 7:40464. doi: 10.1038/srep40464
Liu, C.-Y. Y., Lin, H.-H. H., Tang, M.-J. J., and Wang, Y.-K. K. (2015). Vimentin contributes to epithelial-mesenchymal transition cancer cell mechanics by mediating cytoskeletal organization and focal adhesion maturation. Oncotarget 6, 15966–15983. doi: 10.18632/oncotarget.3862
Lu, H., Hu, L., Yu, L., Wang, X., Urvalek, A. M., Li, T., et al. (2014). KLF8 and FAK cooperatively enrich the active MMP14 on the cell surface required for the metastatic progression of breast cancer. Oncogene 33, 2909–2917. doi: 10.1038/onc.2013.247
Maeda, S., Dean, D., Gomez, R., Schwartz, Z., and Boyan, B. (2002). The first stage of transforming growth factor β1 activation is release of the large latent complex from the extracellular matrix of growth plate chondrocytes by matrix vesicle stromelysin-1 (MMP-3). Calcif. Tissue Int. 70, 54–65. doi: 10.1007/s002230010032
Mak, K. M., Png, C. Y. M., and Lee, D. J. (2016). Type V collagen in health, disease, and fibrosis. Anat. Rec. 299, 613–629. doi: 10.1002/ar.23330
Mamuya, F. A., and Duncan, M. K. (2012). aV integrins and TGF-β-induced EMT: a circle of regulation. J. Cell. Mol. Med. 16, 445–455. doi: 10.1111/j.1582-4934.2011.01419.x
Mao, Y., and Schwarzbauer, J. E. (2005). Fibronectin fibrillogenesis, a cell-mediated matrix assembly process. Matrix Biol. 24, 389–399. doi: 10.1016/j.matbio.2005.06.008
Markowski, M. C., Brown, A. C., and Barker, T. H. (2012). Directing epithelial to mesenchymal transition through engineered microenvironments displaying orthogonal adhesive and mechanical cues. J. Biomed. Mater. Res. A 100A, 2119–2127. doi: 10.1002/jbm.a.34068
Maschler, S., Grunert, S., Danielopol, A., Beug, H., and Wirl, G. (2004). Enhanced tenascin-C expression and matrix deposition during Ras/TGF-β-induced progression of mammary tumor cells. Oncogene 23, 3622–3633. doi: 10.1038/sj.onc.1207403
McGuire, J. K., Li, Q., and Parks, W. C. (2003). Matrilysin (matrix metalloproteinase-7) mediates E-cadherin ectodomain shedding in injured lung epithelium. Am. J. Pathol. 162, 1831–1843. doi: 10.1016/S0002-9440(10)64318-0
McKee, K. K., Harrison, D., Capizzi, S., and Yurchenco, P. D. (2007). Role of laminin terminal globular domains in basement membrane assembly. J. Biol. Chem. 282, 21437–21447. doi: 10.1074/jbc.M702963200
Medici, D., and Nawshad, A. (2010). Type I collagen promotes epithelial-mesenchymal transition through ILK-dependent activation of NF-κB and LEF-1. Matrix Biol. 29, 161–165. doi: 10.1016/j.matbio.2009.12.003
Menke, A., Philippi, C., Vogelmann, R., Seidel, B., Lutz, M. P., Adler, G., et al. (2001). Down-regulation of E-cadherin gene expression by collagen type I and type III in pancreatic cancer cell lines. Cancer Res. 61, 3508–3517.
Mierke, C. T., Frey, B., Fellner, M., Herrmann, M., and Fabry, B. (2011). Integrin α5β1 facilitates cancer cell invasion through enhanced contractile forces. J. Cell Sci. 124, 369–383. doi: 10.1242/jcs.071985
Morgan, M., Byron, A., Humphries, M., and Bass, M. (2009). Giving off mixed signals–distinct functions of α5β1 and αVβ3 integrins in regulating cell behaviour. IUBMB Life 61, 731–738. doi: 10.1002/iub.200
Mori, H., Lo, A. T., Inman, J. L., Alcaraz, J., Ghajar, C. M., Mott, J. D., et al. (2013). Transmembrane/cytoplasmic, rather than catalytic, domains of Mmp14 signal to MAPK activation and mammary branching morphogenesis via binding to integrin β1. Development 140, 343–352. doi: 10.1242/dev.084236
Morini, M., Mottolese, M., Ferrari, N., Ghiorzo, F., Buglioni, S., Mortarini, R., et al. (2000). The α3β1 integrin is associated with mammary carcinoma cell metastasis, invasion, and gelatinase B (mmp-9) activity. Int. J. Cancer 87, 336–342. doi: 10.1002/1097-0215(20000801)87:3<336::AID-IJC5>3.0.CO;2-3
Morra, L., and Moch, H. (2011). Periostin expression and epithelial-mesenchymal transition in cancer: a review and an update. Virchows Arch. 459, 465–475. doi: 10.1007/s00428-011-1151-5
Morrissey, M. A., and Sherwood, D. R. (2015). An active role for basement membrane assembly and modification in tissue sculpting. J. Cell Sci. 128, 1661–1668. doi: 10.1242/jcs.168021
Mu, D., Cambier, S., Fjellbirkeland, L., Baron, J. L., Munger, J. S., Kawakatsu, H., et al. (2002). The integrin αvβ8 mediates epithelial homeostasis through MT1-MMP-dependent activation of TGF-β1. J. Cell. Biol. 157, 493–507. doi: 10.1083/jcb.200109100
Murphy-Ullrich, J. E., and Sage, E. H. (2014). Revisiting the matricellular concept. Matrix Biol. 37, 1–14. doi: 10.1016/j.matbio.2014.07.005
Myers, J. C., Amenta, P. S., Dion, A. S., Sciancalepore, J. P., Nagaswami, C., Weisel, J. W., et al. (2007). The molecular structure of human tissue type XV presents a unique conformation among the collagens. Biochem. J. 404, 535–544. doi: 10.1042/BJ20070201
Myllymäki, S. M., Teräväinen, T. P., and Manninen, A. (2011). Two distinct integrin-mediated mechanisms contribute to apical lumen formation in epithelial cells. PLoS ONE 6:e19453. doi: 10.1371/journal.pone.0019453
Nabeshima, K., Inoue, T., Shimao, Y., and Sameshima, T. (2002). Matrix metalloproteinases in tumor invasion: role for cell migration. Pathol. Int. 52, 255–264. doi: 10.1046/j.1440-1827.2002.01343.x
Nagase, H., Visse, R., and Murphy, G. (2006). Structure and function of matrix metalloproteinases and TIMPs. Cardiovasc. Res. 69, 562–573. doi: 10.1016/j.cardiores.2005.12.002
Nardone, G., Oliver-De La Cruz, J., Vrbsky, J., Martini, C., Pribyl, J., Skládal, P., et al. (2017). YAP regulates cell mechanics by controlling focal adhesion assembly. Nat. Commun. 8:15321. doi: 10.1038/ncomms15321
Noe, V., Fingleton, B., Jacobs, K., Crawford, H. C., Vermeulen, S., Steelant, W., et al. (2001). Release of an invasion promoter E-cadherin fragment by matrilysin and stromelysin-1. J. Cell Sci. 114, 111–118.
O'Connor, J. W., Riley, P. N., Nalluri, S. M., Ashar, P. K., and Gomez, E. W. (2015). Matrix rigidity mediates TGFβ1-induced epithelial-myofibroblast transition by controlling cytoskeletal organization and MRTF-A localization. J. Cell. Physiol. 230, 1829–1839. doi: 10.1002/jcp.24895
Octavio, G.-H., Cristina, G.-V., Pedro, C.-R., Emmanuel, R.-U., Sonia, C.-O., Octavio, R.-H., et al. (2015). Extracellular vesicles from women with breast cancer promote an epithelial-mesenchymal transition-like process in mammary epithelial cells MCF10a. Tumour Biol. 36, 9649–9659. doi: 10.1007/s13277-015-3711-9
Onodera, T., Sakai, T., Hsu, J. C.-f., Matsumoto, K., Chiorini, J. A., and Yamada, K. M. (2010). Btbd7 regulates epithelial cell dynamics and branching morphogenesis. Science 329, 562–565. doi: 10.1126/science.1191880
Page-McCaw, A., Ewald, A. J., and Werb, Z. (2007). Matrix metalloproteinases and the regulation of tissue remodelling. Nat. Rev. Mol. Cell Biol. 8, 221–233. doi: 10.1038/nrm2125
Pardo, A., Cabrera, S., Maldonado, M., and Selman, M. (2016). Role of matrix metalloproteinases in the pathogenesis of idiopathic pulmonary fibrosis. Respir. Res. 17:23. doi: 10.1186/s12931-016-0343-6
Park, J., and Scherer, P. E. (2012). Adipocyte-derived endotrophin promotes malignant tumor progression. J. Clin. Invest. 122, 4243–4256. doi: 10.1172/JCI63930
Park, J., and Schwarzbauer, J. E. (2014). Mammary epithelial cell interactions with fibronectin stimulate epithelial-mesenchymal transition. Oncogene 33, 1649–1657. doi: 10.1038/onc.2013.118
Park, M.-J., Kim, M.-S., Park, I.-C., Kang, H.-S., Yoo, H., Park, S. H., et al. (2002). PTEN suppresses hyaluronic acid-induced matrix metalloproteinase-9 expression in U87mg glioblastoma cells through focal adhesion kinase dephosphorylation. Cancer Res. 62, 6318–6322.
Peng, S.-T., Su, C.-H., Kuo, C.-C., Shaw, C.-F., and Wang, H.-S. (2007). CD44 crosslinking-mediated matrix metalloproteinase-9 relocation in breast tumor cells leads to enhanced metastasis. Int. J. Oncol. 31, 1119–1126. doi: 10.3892/ijo.31.5.1119
Petrini, I., Barachini, S., Carnicelli, V., Galimberti, S., Modeo, L., Boni, R., et al. (2016). ED-B fibronectin expression is a marker of epithelial-mesenchymal transition in translational oncology. Oncotarget 8, 4914–4921. doi: 10.18632/oncotarget.13615
Porsch, H., Bernert, B., Mehić, M., Theocharis, A. D., Heldin, C.-H., and Heldin, P. (2013). Efficient TGFβ-induced epithelial-mesenchymal transition depends on hyaluronan synthase HAS2. Oncogene 32, 4355–4365. doi: 10.1038/onc.2012.475
Qiao, B., Johnson, N. W., and Gao, J. (2010). Epithelial-mesenchymal transition in oral squamous cell carcinoma triggered by transforming growth factor-beta1 is Snail family-dependent and correlates with matrix metalloproteinase-2 and -9 expressions. Int. J. Oncol. 37, 663–668. doi: 10.3892/ijo_00000715
Radisky, D. C., Levy, D. D., Littlepage, L. E., Liu, H., Nelson, C. M., Fata, J. E., et al. (2005). Rac1b and reactive oxygen species mediate MMP-3-induced EMT and genomic instability. Nature 436, 123–127. doi: 10.1038/nature03688
Ramirez, N. E., Zhang, Z., Madamanchi, A., Boyd, K. L., O'Rear, L. D., Nashabi, A., et al. (2011). The α2β1 integrin is a metastasis suppressor in mouse models and human cancer. J. Clin. Invest. 121, 226–237. doi: 10.1172/JCI42328
Rammal, H., Saby, C., Magnien, K., Van-Gulick, L., Garnotel, R., Buache, E., et al. (2016). Discoidin domain receptors: potential actors and targets in cancer. Front. Pharmacol. 7:55. doi: 10.3389/fphar.2016.00055
Repetto, O., De Paoli, P., De Re, V., Canzonieri, V., and Cannizzaro, R. (2014). Levels of soluble E-cadherin in breast, gastric, and colorectal cancers. Biomed. Res. Int. 2014:408047. doi: 10.1155/2014/408047
Ricard-Blum, S. (2011). The collagen family. Cold Spring Harbor Perspect. Biol. 3:ea004978. doi: 10.1101/cshperspect.a004978
Rifkin, D. B. (2005). Latent transforming growth factor-β (TGF-β) binding proteins: orchestrators of TGF-β availability. J. Biol. Chem. 280, 7409–7412. doi: 10.1074/jbc.R400029200
Robert, G., Gaggioli, C., Bailet, O., Chavey, C., Abbe, P., Aberdam, E., et al. (2006). SPARC represses E-cadherin and induces mesenchymal transition during melanoma development. Cancer Res. 66, 7516–7523. doi: 10.1158/0008-5472.CAN-05-3189
Robertson, I. B., Horiguchi, M., Zilberberg, L., Dabovic, B., Hadjiolova, K., and Rifkin, D. B. (2015). Latent TGF-β-binding proteins. Matrix Biol. 47, 44–53. doi: 10.1016/j.matbio.2015.05.005
Rodríguez, D., Morrison, C. J., and Overall, C. M. (2010). Matrix metalloproteinases: what do they not do? New substrates and biological roles identified by murine models and proteomics. Biochim. Biophys. Acta 1803, 39–54. doi: 10.1016/j.bbamcr.2009.09.015
Rousselle, P., Keene, D. R., Ruggiero, F., Champliaud, M.-F., van der Rest, M., and Burgeson, R. E. (1997). Laminin 5 binds the NC-1 domain of type VII collagen. J. Cell Biol. 138, 719–728. doi: 10.1083/jcb.138.3.719
Rozanov, D. V., Deryugina, E. I., Monosov, E. Z., Marchenko, N. D., and Strongin, A. Y. (2004). Aberrant, persistent inclusion into lipid rafts limits the tumorigenic function of membrane type-1 matrix metalloproteinase in malignant cells. Exp. Cell Res. 293, 81–95. doi: 10.1016/j.yexcr.2003.10.006
Scott, L. E., Mair, D. B., Narang, J. D., Feleke, K., and Lemmon, C. A. (2015). Fibronectin fibrillogenesis facilitates mechano-dependent cell spreading, force generation, and nuclear size in human embryonic fibroblasts. Integr. Biol. 7, 1454–1465. doi: 10.1039/c5ib00217f
Seifert, A., and Posern, G. (2017). Tightly controlled MRTF–a activity regulates epithelial differentiation during formation of mammary acini. Breast Cancer Res. 19:68. doi: 10.1186/s13058-017-0860-3
Sethi, A., Mao, W., Wordinger, R. J., and Clark, A. F. (2011). Transforming growth factor-β induces extracellular matrix protein cross-linking lysyl oxidase (LOX) genes in human trabecular meshwork cells. Invest. Ophthal. Vis. Sci. 52, 5240–5250. doi: 10.1167/iovs.11-7287
Shen, C.-J., Kuo, Y.-L., Chen, C.-C., Chen, M.-J., and Cheng, Y.-M. (2017a). MMP1 expression is activated by slug and enhances multi-drug resistance (MDR) in breast cancer. PLoS ONE 12:e0174487. doi: 10.1371/journal.pone.0174487
Shen, Z., Wang, X., Yu, X., Zhang, Y., and Qin, L. (2017b). MMP16 promotes tumor metastasis and indicates poor prognosis in hepatocellular carcinoma. Oncotarget 8, 72197–72204. doi: 10.18632/oncotarget.20060
Shintani, Y., Fukumoto, Y., Chaika, N., Svoboda, R., Wheelock, M. J., and Johnson, K. R. (2008a). Collagen I-mediated up-regulation of N-cadherin requires cooperative signals from integrins and discoidin domain receptor 1. J. Cell Biol. 180, 1277–1289. doi: 10.1083/jcb.200708137
Shintani, Y., Maeda, M., Chaika, N., Johnson, K. R., and Wheelock, M. J. (2008b). Collagen I promotes epithelial-to-mesenchymal transition in lung cancer cells via transforming growth factor-β signaling. Am. J. Respir. Cell Mol. Biol. 38, 95–104. doi: 10.1165/rcmb.2007-0071OC
Shoulders, M. D., and Raines, R. T. (2009). Collagen structure and stability. Annu. Rev. Biochem. 78, 929–958. doi: 10.1146/annurev.biochem.77.032207.120833
Singh, P., Carraher, C., and Schwarzbauer, J. E. (2010). Assembly of fibronectin extracellular matrix. Annu. Rev. Cell Dev. Biol. 26, 397–419. doi: 10.1146/annurev-cellbio-100109-104020
Sottile, J., Hocking, D. C., and Schwartz, M. (2002). Fibronectin polymerization regulates the composition and stability of extracellular matrix fibrils and cell-matrix adhesions. Mol. Biol. Cell 13, 3546–3559. doi: 10.1091/mbc.e02-01-0048
Sternlicht, M. D., and Werb, Z. (2001). How matrix metalloproteinases regulate cell behavior. Annu. Rev. Cell Dev. Biol. 17, 463–516. doi: 10.1146/annurev.cellbio.17.1.463
Sugiura, T., and Berditchevski, F. (1999). Function of α3β1-tetraspanin protein complexes in tumor cell invasion. Evidence for the role of the complexes in production of matrix metalloproteinase 2 (Mmp-2). J. Cell Biol. 146, 1375–1389. doi: 10.1083/jcb.146.6.1375
Sun, B., Fang, Y., Li, Z., Chen, Z., and Xiang, J. (2015). Role of cellular cytoskeleton in epithelial-mesenchymal transition process during cancer progression. Biomed. Rep. 3, 603–610. doi: 10.3892/br.2015.494
Symoens, S., Renard, M., Bonod-Bidaud, C., Syx, D., Vaganay, E., Malfait, F., Ricard-Blum, S., et al. (2011). Identification of binding partners interacting with the α1-N-propeptide of type V collagen. Biochem. J. 433, 371–381. doi: 10.1042/BJ20101061
Têtu, B., Brisson, J., Wang, C. S., Lapointe, H., Beaudry, G., Blanchette, C., et al. (2006). The influence of MMP-14, TIMP-2 and MMP-2 expression on breast cancer prognosis. Breast Cancer Res. 8:R28. doi: 10.1186/bcr1503
Toole, B. P. (2004). Hyaluronan: from extracellular glue to pericellular cue. Nat. Rev. Cancer 4, 528–539. doi: 10.1038/nrc1391
Tseng, Q., Duchemin-Pelletier, E., Deshiere, A., Balland, M., Guillou, H., Filhol, O., et al. (2012). Spatial organization of the extracellular matrix regulates cell–cell junction positioning. Proc. Natl. Acad. Sci. U.S.A. 109, 1506–1511. doi: 10.1073/pnas.1106377109
Tumova, S., Woods, A., and Couchman, J. R. (2000). Heparan sulfate chains from glypican and syndecans bind the Hep II domain of fibronectin similarly despite minor structural differences. J. Biol. Chem. 275, 9410–9417. doi: 10.1074/jbc.275.13.9410
Turley, E. A., Noble, P. W., and Bourguignon, L. Y. W. (2002). Signaling properties of hyaluronan receptors. J. Biol. Chem. 277, 4589–4592. doi: 10.1074/jbc.R100038200
Vega, M. E., and Schwarzbauer, J. E. (2016). Collaboration of fibronectin matrix with other extracellular signals in morphogenesis and differentiation. Curr. Opin. Cell Biol. 42, 1–6. doi: 10.1016/j.ceb.2016.03.014
Voloshenyuk, T. G., Landesman, E. S., Khoutorova, E., Hart, A. D., and Gardner, J. D. (2011). Induction of cardiac fibroblast lysyl oxidase by TGF-β1 requires PI3k/Akt, Smad3, and MAPK signaling. Cytokine 55, 90–97. doi: 10.1016/j.cyto.2011.03.024
Walsh, L. A., Nawshad, A., and Medici, D. (2011). Discoidin domain receptor 2 is a critical regulator of epithelial-mesenchymal transition. Matrix Biol. 30, 243–247. doi: 10.1016/j.matbio.2011.03.007
Wang, C.-Z., Yeh, Y.-C., and Tang, M.-J. (2009). DDR1/E-cadherin complex regulates the activation of DDR1 and cell spreading. Am. J. Physiol. Cell Physiol. 297, C419–C429. doi: 10.1152/ajpcell.00101.2009
Wang, J. P., and Hielscher, A. (2017). Fibronectin: how its Aberrant expression in tumors may improve therapeutic targeting. J. Cancer 8, 674–682. doi: 10.7150/jca.16901
Wang, T., Wang, M., Fang, S., Wang, Q., Fang, R., and Chen, J. (2017). Fibulin-4 is associated with prognosis of endometrial cancer patients and inhibits cancer cell invasion and metastasis via Wnt/β-catenin signaling pathway. Oncotarget 8, 18991–19012. doi: 10.18632/oncotarget.15086
Wang, X., Lu, H., Urvalek, A. M., Li, T., Yu, L., Lamar, J., et al. (2011). KLF8 promotes human breast cancer cell invasion and metastasis by transcriptional activation of MMP9. Oncogene 30, 1901–1911. doi: 10.1038/onc.2010.563
Wang, Z., Symons, J. M., Goldstein, S. L., McDonald, A., Miner, J. H., and Kreidberg, J. A. (1999). (Alpha)3(beta)1 integrin regulates epithelial cytoskeletal organization. J. Cell Sci. 112, 2925–2935.
Waterman, E. A., Sakai, N., Nguyen, N. T., Horst, B. A. J., Veitch, D. P., Dey, C. N., et al. (2007). A laminin-collagen complex drives human epidermal carcinogenesis through phosphoinositol-3-kinase activation. Cancer Res. 67, 4264–4270. doi: 10.1158/0008-5472.CAN-06-4141
Wei, S. C., Fattet, L., Tsai, J. H., Guo, Y., Pai, V. H., Majeski, H. E., et al. (2015). Matrix stiffness drives epithelial-mesenchymal transition and tumour metastasis through a TWIST1-G3bp2 mechanotransduction pathway. Nat. Cell Biol. 17, 678–688. doi: 10.1038/ncb3157
Weinberg, S. H., Mair, D. B., and Lemmon, C. A. (2017). Mechanotransduction dynamics at the cell-matrix interface. Biophys. J. 112, 1962–1974. doi: 10.1016/j.bpj.2017.02.027
Whipple, R. A., Matrone, M. A., Cho, E. H., Balzer, E. M., Vitolo, M. I., Yoon, J. R., et al. (2010). Epithelial-to-mesenchymal transition promotes tubulin detyrosination and microtentacles that enhance endothelial engagement. Cancer Res. 70, 8127–8137. doi: 10.1158/0008-5472.CAN-09-4613
White, D. P., Caswell, P. T., and Norman, J. C. (2007). αvβ3 and α5β1 integrin recycling pathways dictate downstream Rho kinase signaling to regulate persistent cell migration. J. Cell. Biol. 177, 515–525. doi: 10.1083/jcb.200609004
Wilkins-Port, C. E., and Higgins, P. J. (2007). Regulation of extracellular matrix remodeling following transforming growth factor-β1/epidermal growth factor-stimulated epithelial-mesenchymal transition in human premalignant keratinocytes. Cells Tissues Organs 185, 116–122. doi: 10.1159/000101312
Williams, C. M., Engler, A. J., Slone, R. D., Galante, L. L., and Schwarzbauer, J. E. (2008). Fibronectin expression modulates mammary epithelial cell proliferation during acinar differentiation. Cancer Res. 68, 3185–3192. doi: 10.1158/0008-5472.CAN-07-2673
Woods, A., Longley, R. L., Tumova, S., and Couchman, J. R. (2000). Syndecan-4 binding to the high affinity heparin-binding domain of fibronectin drives focal adhesion formation in fibroblasts. Arch. Biochem. Biophys. 374, 66–72. doi: 10.1006/abbi.1999.1607
Wu, B., Crampton, S. P., and Hughes, C. C. W. (2007). Wnt signaling induces matrix metalloproteinase expression and regulates T cell transmigration. Immunity 26, 227–239. doi: 10.1016/j.immuni.2006.12.007
Xiao, Q., Jiang, Y., Liu, Q., Yue, J., Liu, C., Zhao, X., et al. (2015). Minor type IV collagen α5 chain promotes cancer progression through Discoidin domain receptor-1. PLoS Genet. 11:e1005249. doi: 10.1371/journal.pgen.1005249
Xie, L., Law, B. K., Chytil, A. M., Brown, K. A., Aakre, M. E., and Moses, H. L. (2004). Activation of the Erk pathway is required for TGF-β1-induced EMT in vitro. Neoplasia 6, 603–610. doi: 10.1593/neo.04241
Xu, J., Rodriguez, D., Petitclerc, E., Kim, J. J., Hangai, M., Yuen, S. M., et al. (2001). Proteolytic exposure of a cryptic site within collagen type IV is required for angiogenesis and tumor growth in vivo. J. Cell Biol. 154, 1069–1080. doi: 10.1083/jcb.200103111
Yamashita, C. M., Dolgonos, L., Zemans, R. L., Young, S. K., Robertson, J., Briones, N., et al. (2011). Matrix metalloproteinase 3 is a mediator of pulmonary fibrosis. Am. J. Pathol. 179, 1733–1745. doi: 10.1016/j.ajpath.2011.06.041
Yanagisawa, H., Schluterman, M. K., and Brekken, R. A. (2009). Fibulin-5, an integrin-binding matricellular protein: its function in development and disease. J. Cell Commun. Signal. 3, 337–347. doi: 10.1007/s12079-009-0065-3
Yang, C., Zeisberg, M., Lively, J. C., Nyberg, P., Afdhal, N., and Kalluri, R. (2003). Integrin α1β1 and α2β1 are the key regulators of hepatocarcinoma cell invasion across the fibrotic matrix microenvironment. Cancer Res. 63, 8312–8317. doi: 10.1186/1755-1536-6-17
Yonemura, S., Wada, Y., Watanabe, T., Nagafuchi, A., and Shibata, M. (2010). Alpha-catenin as a tension transducer that induces adherens junction development. Nat. Cell Biol. 12, 533–542. doi: 10.1038/ncb2055
Yoshida, T., Akatsuka, T., and Imanaka-Yoshida, K. (2015). Tenascin-C and integrins in cancer. Cell Adhes. Migr. 9, 96–104. doi: 10.1080/19336918.2015.1008332
Yu, Q., and Stamenkovic, I. (2000). Cell surface-localized matrix metalloproteinase-9 proteolytically activates TGF-β and promotes tumor invasion and angiogenesis. Genes Dev. 14, 163–176. doi: 10.1101/gad.14.2.163
Yurchenco, P. D. (2011). Basement membranes: cell scaffoldings and signaling platforms. Cold Spring Harbor Perspect. Biol. 3:a004911. doi: 10.1101/cshperspect.a004911
Yurchenco, P. D., and Patton, B. L. (2009). Developmental and pathogenic mechanisms of basement membrane assembly. Curr. Pharm. Des. 15, 1277–1294. doi: 10.2174/138161209787846766
Zhang, F., Tom, C. C., Kugler, M. C., Ching, T.-T., Kreidberg, J. A., Wei, Y., et al. (2003). Distinct ligand binding sites in integrin α3β1 regulate matrix adhesion and cell–cell contact. J. Cell Biol. 163, 177–188. doi: 10.1083/jcb.200304065
Zhang, K., Corsa, C. A., Ponik, S. M., Prior, J. L., Piwnica-Worms, D., Eliceiri, K. W., et al. (2013). The collagen receptor discoidin domain receptor 2 stabilizes SNAIL1 to facilitate breast cancer metastasis. Nat. Cell Biol. 15, 677–687. doi: 10.1038/ncb2743
Zhang, S., Miao, Y., Zheng, X., Gong, Y., Zhang, J., Zou, F., et al. (2017). STIM1 and STIM2 differently regulate endogenous Ca2+ entry and promote TGF-β-induced EMT in breast cancer cells. Biochem. Biophys. Res. Commun. 488, 74–80. doi: 10.1016/j.bbrc.2017.05.009
Zhang, Y., Thant, A. A., Machida, K., Ichigotani, Y., Naito, Y., Hiraiwa, Y., et al. (2002). Hyaluronan-CD44s signaling regulates matrix metalloproteinase-2 secretion in a human lung carcinoma cell line QG90. Cancer Res. 62, 3962–3965.
Zhang, Z., Liu, X., Feng, B., Liu, N., Wu, Q., Han, Y., et al. (2015). STIM1, a direct target of microRNA-185, promotes tumor metastasis and is associated with poor prognosis in colorectal cancer. Oncogene 34, 4808–4820. doi: 10.1038/onc.2014.404
Zhao, H., Chen, Q., Alam, A., Cui, J., Suen, K. C., Soo, A. P., et al. (2018). The role of osteopontin in the progression of solid organ tumour. Cell Death Dis. 9:356. doi: 10.1038/s41419-018-0391-6
Zilberberg, L., Todorovic, V., Dabovic, B., Horiguchi, M., Couroussé, T., Sakai, L. Y., et al. (2012). Specificity of latent TGF-β binding protein (LTBP) incorporation into matrix: role of fibrillins and fibronectin. J. Cell. Physiol. 227, 3828–3836. doi: 10.1002/jcp.24094
Zoltan-Jones, A., Huang, L., Ghatak, S., and Toole, B. P. (2003). Elevated Hyaluronan production induces mesenchymal and transformed properties in epithelial cells. J. Biol. Chem. 278, 45801–45810. doi: 10.1074/jbc.M308168200
Keywords: extracellular matrix (ECM), epithelial-mesenchymal transition (EMT), mechanobiology, cellular signaling, epithelial phenotype
Citation: Scott LE, Weinberg SH and Lemmon CA (2019) Mechanochemical Signaling of the Extracellular Matrix in Epithelial-Mesenchymal Transition. Front. Cell Dev. Biol. 7:135. doi: 10.3389/fcell.2019.00135
Received: 18 January 2019; Accepted: 04 July 2019;
Published: 19 July 2019.
Edited by:
Charles D. Little, University of Kansas Medical Center, United StatesReviewed by:
Lidija Radenovic, University of Belgrade, SerbiaParthasarathy Chandrakesan, University of Oklahoma Health Sciences Center, United States
Copyright © 2019 Scott, Weinberg and Lemmon. This is an open-access article distributed under the terms of the Creative Commons Attribution License (CC BY). The use, distribution or reproduction in other forums is permitted, provided the original author(s) and the copyright owner(s) are credited and that the original publication in this journal is cited, in accordance with accepted academic practice. No use, distribution or reproduction is permitted which does not comply with these terms.
*Correspondence: Christopher A. Lemmon, Y2xlbW1vbkB2Y3UuZWR1