- 1Jerzy Haber Institute of Catalysis and Surface Chemistry Polish Academy of Sciences, Kraków, Poland
- 2Institut für Molekulare Mikrobiologie und Biotechnologie, Westfälische Wilhelms-Universität Münster, Münster, Germany
- 3Environmental Sciences Department, King Abdulaziz University, Jeddah, Saudi Arabia
- 4Department of Product Technology and Ecology, Cracow University of Economics, Kraków, Poland
- 5Department of Management Process, Cracow University of Economics, Kraków, Poland
Over the past decades, enormous progress has been achieved with regard to research on environmentally friendly polymers. One of the most prominent families of such biopolymers are bacterially synthesized polyhydroxyalkanoates (PHAs) that have been known since the 1920s. However, only as recent as the 1990s have extensive studies sprung out exponentially in this matter. Since then, different areas of exploration of these intriguing materials have been uncovered. However, no systematic review of undertaken efforts has been conducted so far. Therefore, we have performed an unbiased search of up-to-date literature to reveal trending topics in the research of PHAs over the past three decades by data mining of 2,227 publications. This allowed us to identify eight past and current trends in this area. Our study provides a comprehensive review of these trends and speculates where PHA research is heading.
Introduction
Polyhydroxyalkanoates (PHAs) have been researched since their discovery in 1920. An exponential burst of scientific publications started in the early 1990s. Since then, yearly, more and more scientific documents are appearing, increasing our knowledge in this realm of biopolymers. A systematic literature review is an important method of understanding the field of study. However, due to high labor intensity, it is usually limited to narrow subjects. They are, in most cases, narrative and qualitative (Tranfield et al., 2003). To study the whole field, it is necessary to adopt text-mining toolset and quantitative methods. It is relatively easy to perform analysis based on metadata related to publications, mostly titles, keywords, and abstracts. The required software is readily available. Unfortunately, research by Blake (2010) revealed that authors report <8% of scientific claims in abstracts. Moreover, keywords used in publications are in many cases limited or modified by publishers. Therefore, studies based on metadata cannot be treated as fully reliable. The following limitation of most text-mining studies is predefinition of themes, clusters, or categories. This can prevent the discovery of new topics that were not predefined by researchers. Additionally, in the case of qualitative methods, the risk of researcher bias grows significantly.
The approach offered in this study was designed to overcome such limitations. The analysis was performed using full texts of publications, and categories were discovered in data, not predefined. The well-known quantitative approach to text-mining was supplemented with new and original tools (for a full description, please see the Materials and Methods section). This allowed authors to identify not only categories but also trends. A diagram of the proposed approach compared with systematic literature review steps is presented in Figure 1. The procedure is consistent with general rules for systematic literature reviews (Ananiadou et al., 2009). In this article, we have sampled scientific publications from Web of Science Core Collection that were firmly related to PHA research and performed an in silico analysis of the most frequently appearing words within the main text. We have omitted on purpose reviews that are not original per se, thus focusing only on scientific publications concerning the generation of new data in areas related to PHAs at the time of their publications. This allowed us to identify scientifically relevant keywords that enabled us to group the publications into clusters, groups, and then trends that have been emerging since the late 1980s.
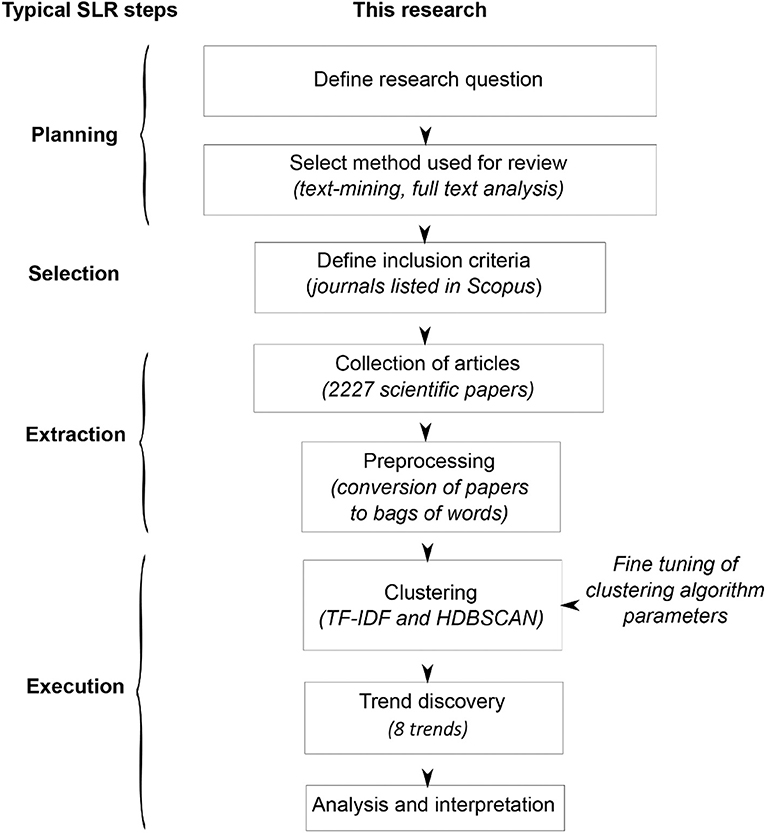
Figure 1. Diagram of the method and steps of systematic literature review (SLR). HDBSCAN, Hierarchical Density-Based Spatial Clustering of Applications with Noise; TF-IDF, term frequency–inverted document frequency.
Finding Trends
Collection of Publications
In order to collect publications, it was necessary to define search criteria, which included:
- the main subject of a publication related to PHA polymers (search topic term “polyhydroxyalkanoate”),
- availability of the full text of the publication,
- published between 1988 and 2018,
- only scientific publications were included.
The search was performed in the scientific database Web of Science Core Collection using the command “all fields.” In the result, 2,432 publications were found. Publications from 1988 to 1994 were excluded from the analysis due to an overall small number over these years (34 publications). After removing duplicated, editorials, reviews, and other non-scientific publications, 2,227 studies were left. The number of publications ordered by journal title was presented in Table 1. Only publications from journals, which published more than 10 studies in total with relation to the keyword “polyhydroxyalkanoate” were presented in this table.
Each publication was converted into a text file and prepared for automatic analysis using computer algorithms. In this research, algorithms have been created using Python libraries, including grobid, nltk, scikit-learn, hdbscan, and scipy (Jones et al., 2001; Lopez, 2009; Pedregosa et al., 2011; McInnes et al., 2017).
The corpus of the research can be presented as a network of authors of publications and authors indicated by them in the references. This means that not only the most frequently quoted authors but also the relations between them can be discovered. The source of the relations, for example, includes similarity of covered topics, joint research, and long-term cooperation. The network of relations, therefore, helps to understand trends in the literature better. The network was prepared using Gephi (Figure 2). For the sake of clarity, only links with a number of citations >10 were presented. An analysis of all relations would contain over 60,000 authors and over 530,000 relationships. However, it would not significantly impact the list of the most often quoted authors and the most important relations.
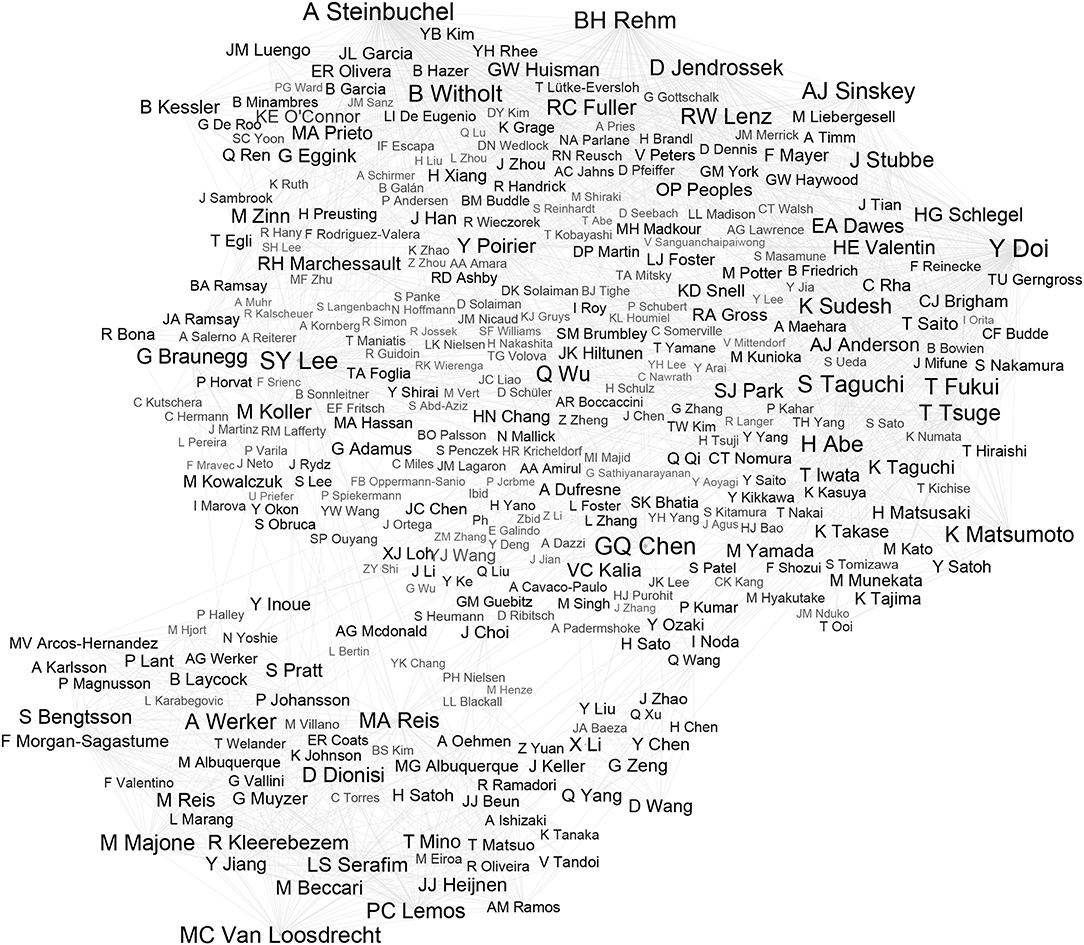
Figure 2. The network of authors of the most frequently quoted in analyzed journals. From each publication, analyzed pairs were identified consisting of: (1) author of the paper and (2) author of referenced paper, represented by the lines in the graphic. All identical pairs in whole corpus were summed up. The greater the sum, the stronger the relation is, which is visualized by the increased font size in the iconographic. The network of relations allows to discover groups of cooperating scientists. These relations are probably based on common university, field of study, etc.
Search for the Most Important Terms
The most important terms are not always those which are the most frequent. In English, the most frequent words are “the” and “of.” In the case of this study, the word “polymer” can be found in every publication. Thus, it does not impact the results. The terms that exist in one publication only are important only for that publication. The most important terms are those which occur in a group of publications. They allow researchers to identify clusters and then trends (Salton and Yang, 1973; Cong et al., 2016). The term frequency–inverted document frequency (TF-IDF) method was designed to solve that problem. It takes into account the frequency of the term but at the same time includes several documents in which that term occurs. The following formula is used:
where:
wi,j—result for term i in document j,
tfi,j—number of occurrences of i in j,
dfi—number of documents containing i,
N—number of documents in corpus (set of documents).
In the result, each publication can be presented as a vector that consists of multiple dimensions. The number of dimensions is equal to the number of terms used in the analysis, usually several thousands. The similarity of the publications as vectors can be assessed using mathematical methods, e.g., cosine similarity (Mihalcea et al., 2006). Some other methods can be used instead of TF-IDF, e.g., Latent Semantic Indexing (LSI) or Latent Dirichlet Allocation (LDA). They can explain the meaning of a text, but the format of results makes their use in further steps of our cluster analysis difficult.
Discovery of Thematic Groups (Clusters)
The similarity of the vectors can help discover thematic groups of publications. It can be done using partitioning or hierarchical clustering methods. As a result of partitioning, all elements of the corpus has to be assigned to one of the predefined partitions, even if it is not similar to the other elements. The hierarchical clustering includes in clusters only those elements that are similar. Therefore, hierarchical clustering is better when searching for trends. The examples of partitioning methods include k-means, affinity propagation, spectral clustering, and agglomerative clustering. In contrast, the examples of clustering are mean shift (based on k-means), DBSCAN, Optics, and HDBSCAN (McInnes et al., 2017).
We decided to use HDBSCAN (Hierarchical Density-Based Spatial Clustering of Applications with Noise), which is a relatively new method (McInnes et al., 2017). The algorithm takes each publication (vector) and checks at what distance it can find similar ones. Then it compares the results, and the densest areas are detected as clusters. The density and number of elements in the cluster can differ. The researcher has to define the minimum cluster size, which should be identified experimentally. In the case of this study, the minimum cluster size was set to 5.
The sample was divided into groups that contained publications published in 5-years overlapping periods starting with 1995–2000 and ending with 2014–2019. Each publication was assigned to all the groups into which it fitted. Cluster analysis was performed in every group separately. Figure 3 presents the number of articles in each year.
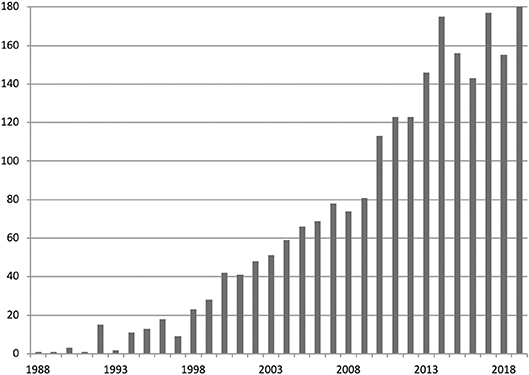
Figure 3. Number of publications in polyhydroxyalkanoate research identified in Web of Science Core Collection for years 1988–2019.
Due to the limited length of the publication, it is not possible to describe HDBSCAN algorithm in detail. Full documentation with examples and comparison to other methods can be found on the dedicated website (hdbscan.readthedocs.io).
Discovery of Trends
Clusters require further analysis to discover trends. Definition of overlapping periods allows automatic detection of trends based on tracking of publications in subsequent corpora. A trend occurs when the average number of articles exceeds a certain expert-determined level per given time. Each step of the analysis leads to the discovery of slightly different clusters in which publication tracking is possible. Thanks to tracking, an evolution of clusters can be observed, and trends can be identified. As a result of the analysis, several types of trends can be discovered:
- long-lasting trends that exist and evolve during the studied period,
- declining trends which end during the studied period,
- emerging trends which begin during the studied period,
- ephemeris trends that begin and end during the studied period.
The final step is trends verification, description, and interpretation, which has to be performed by researchers without the help of algorithms. The researcher has to decide whether trends have been identified correctly. The algorithm usually identifies more trends, as it is very sensitive. They have to be merged by researchers. High sensitivity is intentional, as a lower one could lead to an unjustified merger. Trends can be described based on the most important terms found during the TF-IDF analysis of publications that constitute the trend. The interpretation phase should help to highlight changes within the trends and try to predict their future evolution.
Trends Unveiled
The unbiased data mining allowed us to identify eight trends that have been spanning since 1988 (Figure 4). Below, the reader will find their brief description with links to the more specialist reviews and original publications. Some of them are continuing and will last into the future; the others have peaked for several years and are not anymore persistent in the published literature. Nevertheless, it does not mean that research in these areas is not conducted and probably more detailed and narrower studies, which polish the findings over the trend life span, are conducted.
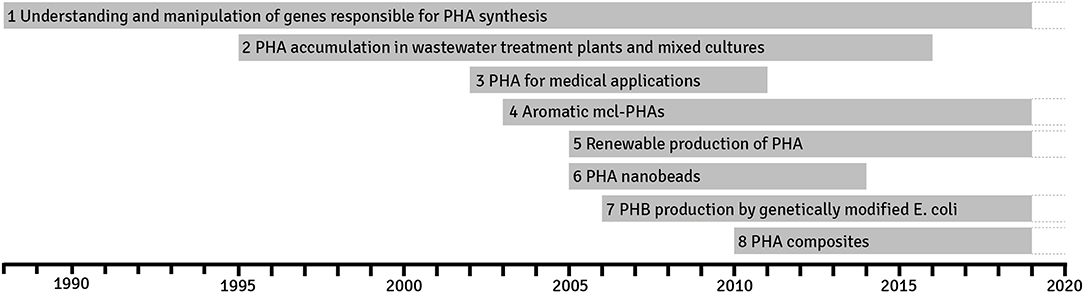
Figure 4. Trends discovered in research on polyhydroxyalkanoates in years 1988–2019 based on the most important terms found during the term frequency–inverted document frequency (TF-IDF) analysis of publications. A trend occurs when the average number of articles exceeds a certain expert-determined level per given time.
Understanding and Manipulation of Genes Responsible for Polyhydroxyalkanoate Synthesis
Years 1988–2019
This trend is the longest appearing in the research of PHAs over the years. The first works relate to understanding the biosynthesis of PHAs in microorganisms, of which more than 300 have been investigated. Primary findings were described in 1988. Slater et al. (1988) succeeded in the expression of poly-3-hydroxybutyrate (PHB) synthesis genes in Escherichia coli by introducing plasmids; at the same time, Schubert et al. (1988) also synthesized PHB in E. coli using cosmids. Since then, several biosynthetic pathways have been discovered to date. One distinct was described for short chain length PHAs, the biosynthesis enzymes encoded in the phaABC operon, whereas for medium chain length PHAs, the most abundant are: pathways of metabolite shunts through β-oxidation, de novo fatty acid synthesis, acyl chain elongation, and others that provide precursors of (R)-3-hydroxyacyls from numerous vital biochemical pathways within a given microorganism (see review, Suriyamongkol et al., 2007; Lu et al., 2009). In parallel to the discovery of microbial enzymatic apparatus leading to the synthesis of these polyesters, three key enzymatic groups, directly responsible for PHA synthesis, are also in the spotlight: PHA synthases (see in Stubbe and Tian, 2003; Nomura and Taguchi, 2007), PHA depolymerases (see in Jendrossek, 1998; Ong et al., 2017), and granule associated proteins (see in Dinjaski and Prieto, 2015; Maestro and Sanz, 2017; Gonzalez-Miro et al., 2019). With the development of molecular genetic tools, the constantly discovered genes were being transferred to other model prokaryotes (e.g., E. coli, see trend PHB production by genetically modified Escherichia coli) and eukaryotes (yeasts, see Breuer et al., 2002, or plants, see Suriyamongkol et al., 2007) in order to increase production yields and thus lower the costs of PHAs. Moreover, directed evolution of enzymes, hybrids generation, and genome manipulations [i.e., through CRISPR/Cas9-based systems (Qin et al., 2018)] not only led to the creation of new robust production strains characterized by good productivities but also allowed for the development of sophisticated polymer bead/enzyme-based platforms (see review Gonzalez-Miro et al., 2019, and also trend PHA nanobeads).
Polyhydroxyalkanoate Accumulation in Wastewater Treatment Plants and Mixed Cultures
Years 1995–2016
The increasing world population causes the production of large quantities of urban and industrial waste. Recycling waste and wastewater opens up an opportunity for reducing their quantity and the treatment costs (Cavaillé et al., 2013). Activated sludge can be an alternative to pure cultures for PHA production due to many PHA-producing bacteria present in activated sludge (Yang et al., 2013). In the wastewater treatment process, the microorganisms from activated sludge display the ability to transform a biodegradable carbon source into PHA before using them for growth (Qu and Liu, 2009). Industrial-scale PHA production is based mainly on pure-culture systems with refined feedstock and sterile cultivation conditions. Both features result in high-energy consumption, thereby strongly increasing production costs (Valentino et al., 2019).
Consequently, PHA cannot be cost-competitive to conventional petroleum-based plastics. An innovative approach has been proposed to combine PHA production with sludge minimization in municipal wastewater treatment. In the publications that make up this trend, both the impact of changing the approach to wastewater on the natural environment (Valentino et al., 2015) and the life cycle assessment (LCA) analysis of the production process (Morgan-Sagastume et al., 2016a), as well as specific examples of biochemical (Yang et al., 2013; Inoue et al., 2016) and industrial processes (Pittmann and Steinmetz, 2014; Valentino et al., 2019) resulting in biopolymers, are presented. In the publications, an economic analysis of the process on the example of selected European countries is also carried out (Pittmann and Steinmetz, 2017). The first reports of a significant relationship between PHA production and activated sludge were published in 1998. The issue of biodegradable polymer production with the use of sludge was raised in this publication, as well as the laboratory scale process was described along with performance analysis depending on the reaction conditions (Satoh et al., 1998). Later studies (after 2000) focus on parameters such as carbon and nitrogen sources, their mutual quantitative relations in the fermentation reaction, and the impact of these parameters on process efficiency (Khardenavis et al., 2005). Further analysis on the example of PHB production describes the impact of critical factors such as dissolved oxygen, pH, and food to microorganism (F/M) ratio in the batch reactor on the reaction efficiency (Qu and Liu, 2009). Researchers also carried out physiochemical properties analysis of the polymers PHB and P(HB-co-HV) to identify production conditions that directly affect final product mechanical properties (Wallen and Rohwedder, 1974; Patel et al., 2009). In works mentioned in this trend, the potential of mixed colony nitrogen-fixing bacteria cultures for producing biodegradable polymers with mechanical and chemical properties similar to those originating from pure culture counterparts is presented. In the latest reports (2019), a description of operating pilot plant designed for the production of biopolymer from wastewater can be found (Valentino et al., 2019).
As a summary, one can indicate that a more economical and much cheaper way of producing biopolymers does not necessarily mean a deterioration of their properties, which further indicates the high desirability of projects involving the study and optimization of the production process of bioplastics from sludge (Patel et al., 2009).
Polyhydroxyalkanoate for Medical Applications
Years 2002–2011
PHAs attract the attention of researchers and medical doctors mainly due to their biocompatibility. This feature allows for the creation of medical devices and implants that are completely safe for use in mammals, including humans. PHAs such as P(3HB) and its breakdown products, 3-hydroxy acids, have been found in many organisms—from bacteria to higher mammals. Furthermore, (R)-3-hydroxybutyric acid is a natural blood component at concentrations between 0.3 and 1.3 mM. This means that PHAs are excellent biocompatible materials and can, therefore, be successfully used for the construction of cell scaffolds, biodegradable sutures, wound dressings, and drug delivery systems (Zinn et al., 2001; Chen and Wu, 2005). In vitro and in vivo studies have shown that among the different PHAs, P(3HB-co-3HHx) retains the chondrocyte phenotype, due to the support of chondrocyte-specific extracellular matrix (ECM), type II collagen, and the promotion of sulfated glycosaminoglycan (sGAG) production (Deng et al., 2003). The unquestionable advantage of a polymer such as P(3HB) is its piezoelectric properties, which are similar to those of bones (van der Walle et al., 2001). Therefore, PHAs have been tested for the use of bone loss engineering, and the results showed no chronic inflammation even after 1 year of use (Porter et al., 2013). It was also noted that PHA polymers are widely used in the construction of nerve tissue regeneration devices, dressing materials, cardiovascular patches, venous–arterial valves, orthopedic pins, adhesion barriers, tendon repair materials, bone marrow scaffolds, cardiovascular stents, and tissue engineering (Chen and Wu, 2005; Valappil et al., 2006; Hazer, 2010; Shrivastav et al., 2013). Due to the wide interest of the biomedical industry in PHA polymers, the chemocompatibility of these polymers was also evaluated by incubating P(3HB) and P(3HB-co-3HV) films with mammalian blood during which it was shown that P(3HB) and P(3HB-co-3HV) in contact with blood did not cause negative reactions (Shrivastav et al., 2013).
The first material made of PHA to receive a positive opinion from the US Food and Drug Administration (FDA) was TephaFLEX®, which is an absorbable surgical floss made of P(4HB). There are other commercially available products made of PHA, i.e., GalaFLEX®, which is made of P(4HB) and is designed for reconstructive surgery, plastic surgery, and soft tissue reinforcement, as well as MonoMax Suture for soft tissue regeneration, BioFiber for tendon repair, and Phasix Mesh for hernia regeneration (Williams et al., 2016). Due to their biocompatibility and the hydrophobicity of PHA, they can be used as drug delivery systems in the form of microcapsules, microspheres, and nanoparticles (Shrivastav et al., 2013). In the course of studies on the release of antibiotics such as gentamicin and sulperazone from sticks constructed with P(3HB-co-3HV), continuous release of these drugs over 2 weeks was observed, and it was proved that the content of longer PHA monomers is conducive to prolonging drug release time (Gursel et al., 2002). It has also been shown that lower crystallinity of PHA results in more controlled release of drugs to surrounding tissues. An example was a mixture of P(3HHx-co-3HO) with tamulosin, which was characterized by better penetration of the active substance into the skin, compared to a mixture of the drug with a short-chain, highly crystalline P(3HB) (Wang et al., 2003b). For further details, the reader is encouraged to see the following reviews: Chen et al. (2017); Lizarraga et al. (2018); Elmowafy et al. (2019), and Grigore et al. (2019).
Processing and Modifications of Polyhydroxyalkanoate Polymers
Years 2003–2019
A natural way to add desirable functionalities to PHA polymers is through monomer alterations. More than 150 monomers have been identified (Steinbüchel et al., 1995) that offer a broad range of physicochemical properties (Dinjaski and Prieto, 2015). Among these, aliphatic monomers are in the spotlight. However, these monomers offer only certain properties to scl-PHAs and mcl-PHAs. The other most researched group of PHA monomers is that of aromatic chemistry. We have identified this subtrend in our in silico analysis (2003–2009), where most of the publications regarding aromatic monomers were produced. However, the first report that disclosed a biosynthesized PHA bearing an aromatic group as a side chain was presented by Fritzsche et al. (1990) in 1990. In later reports, one can find various aromatic constituents being introduced into PHA chains through biosynthesis achieved mainly with use of Pseudomonas strains (Ishii-Hyakutake et al., 2018), recombinant E. coli and Ralstonia eutropha (Mizuno et al., 2018; Yang et al., 2018). Aromatic PHAs show different mechanical characteristics depending on the type of aromatic monomers incorporated in their structure (Ishii-Hyakutake et al., 2018). In numerous literature reports, the aromatic PHAs have been studied widely. Properties such as degradability (Olivera et al., 2001), surface structure (Takagi et al., 2004), solubility (Mizuno et al., 2017), and thermal behavior (Antoun et al., 1991) have been assessed, and their dependency on the polymer's structure was identified (Ishii-Hyakutake et al., 2018).
Another way to improve the properties of PHAs is to create composites with other materials by physical blending with nanoparticles and nanofillers, produce PHA-based multiphase materials, and perform chemical modifications (Table 2). Modifying PHA is justified with concern regarding features such as their durability, shelf lifetime, replacement, and maintenance costs (Visakh and Roy, 2015). Majority of works on improving and modifying PHAs aims to increase their competitiveness concerning traditional plastics both in terms of physicochemical properties and production costs (Pandey et al., 2005). Incorporating nanoparticles into PHA polymer expands the potential application area thanks to the significant enhancement of materials properties. The most commonly used nanofillers are silylated kaolinite (Zhang et al., 2009), carbon nanotubes (Misra et al., 2010), bioactive glass (Misra et al., 2007), nanoclay (Bordes et al., 2009), cellulose nanocrystals (Yu et al., 2011), calcium phosphates (Cichoń et al., 2019), and modified hydroxides (Dagnon et al., 2009).
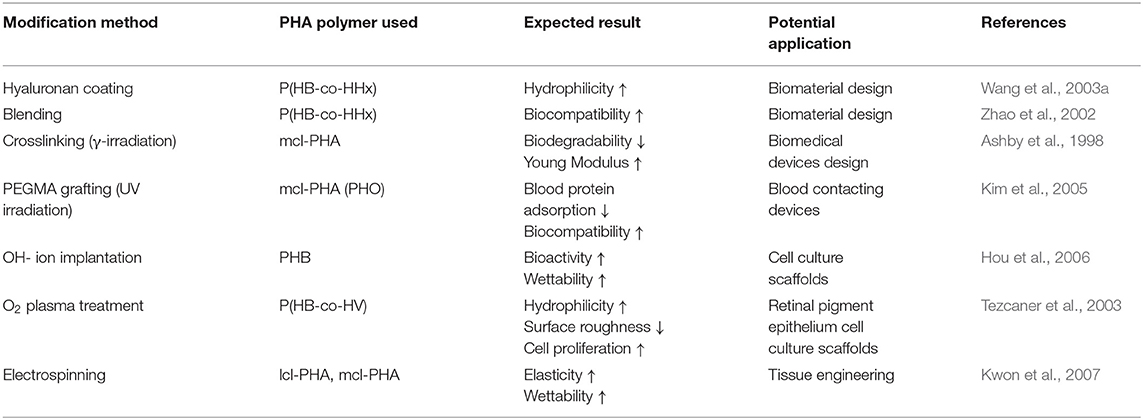
Table 2. Most common methods used for mechanical modification of polyhydroxyalkanoate (PHA) polymers.
To give PHAs new and unique properties, several multiphase materials have been developed, mainly by mixing PHB or P(HB-co-HV) with other products such as plasticizers, fillers, or other polymers (Visakh and Roy, 2015). The structure of a PHA can be modified to obtain a polymer with desired properties for niche applications by chemical processes such as carboxylation, hydroxylation, epoxidation, chlorination, and grafting reaction (Figure 5) (Hazer and Steinbüchel, 2007; Bassas-Galià et al., 2015; Antwi Peprah et al., 2018).
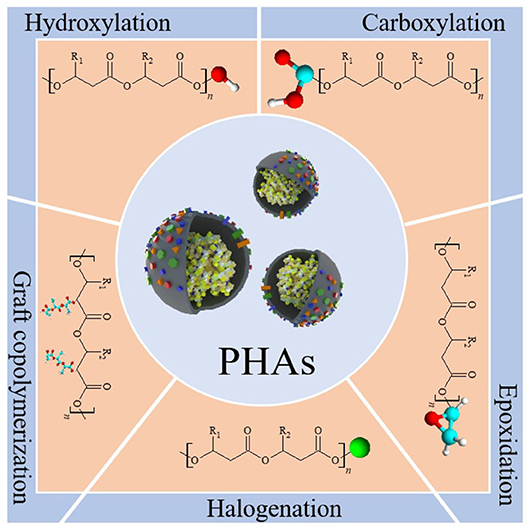
Figure 5. Modification of a polyhydroxyalkanoate (PHA) structure through selected chemical reactions. Carboxylation by addition of carboxylic functional group to the polymeric macromer which can serve as functional binding sites for bioactive moieties. Hydroxylation resulting in shortened hydroxy-terminated PHA chains important in block copolymerization reactions. Epoxidation is useful in diverse reactions such as cross-linking, copolymerization, and bioactive moieties attachment thanks to the high reactivity of the epoxide group. Halogenation which relies on the addition of halogen atoms such as chlorine, bromine, or fluorine to the olefinic bonds of unsaturated PHA, being an excellent method in diversifying functions and applications of the polymer. Graft copolymerization results in the formation of a modified segmented copolymer. Chemical-, radiation-, or plasma discharge-induced reaction evokes improvement of selected polymer properties, i.e., increased wettability or mechanical strength.
A large group of PHA-based materials was obtained from hydroxylated PHAs and have been reviewed in numerous scientific reports (Cheng et al., 2013). An important factor applicable in PHA modification is high reactivity of epoxide group which can participate in numerous reactions like cross-linking (Visakh and Roy, 2015). PHA modification through epoxidation was reported in several cases, i.e., by Bear et al. (1997) and Park et al. (1999). In a different study, Lee et al. (1999) presented a cross-linking reaction of epoxidized PHA using hexamethylene diamine (HMDA). Another excellent method in diversifying the polymer functions and applications is its modification through halogenation (Arkin et al., 2000; Arkin and Hazer, 2002). In their study, Arkin and Hazer modified the PHA-Cl into ammonium salts, thiosulfate moieties, and phenyl derivatives (Arkin et al., 2000). A process of P(HB-co-HHx) direct fluorination was described by Samsuddin et al. (2013) PHA chemical modification by sulfanyl halogenation and its potential application in electrophotographic imaging were also reported and have been patented in 2008 (Mihara et al., 2006).
Enzymatic PHA modification is a mild, specific, and environment-friendly method widely discussed in the literature. The biopolymer can be modified on an enzymatic route by enzymatic degradation synthesis or by using the degradation products itself (Sato et al., 2008; Gumel et al., 2013; Kwiecień et al., 2015). Enzymatic catalyzed surface erosion of PHA can significantly enhance the surface roughness, thereby cell adhesion and proliferation. The raw PHA surface lacks a bioactive ligand to couple with molecules in targeting devices or biosensors (Visakh and Roy, 2015). Therefore, surface erosion and roughening are essential to immobilize bioactive molecules such as insulin, collagen, or fibronectin to expand the polymer's biomedical applications. The demand for customized biodegradable PHAs with unique features and the difficulty in their conventional production by biosynthesis have shifted the current interest in polymer modification and functionalization via chemical, physical, and enzymatic processes (Visakh and Roy, 2015). By precise control over properties such as wettability, elasticity, biocompatibility, biodegradability, or Young's modulus value, one can get a set of smart materials with properties so diverse that they will not only be able to replace traditional polymers but can also contribute to developing new technologies and therapeutic techniques whose use was limited by the lack of material with desired functionality.
Renewable Production of Polyhydroxyalkanoate
Years 2005–2019
PHAs are a promising alternative to petroleum-based polymers; however, their production is still expensive and at industrial scale often competes with the food chain supply. Therefore, a trend emerged in the research of PHAs in 2005 that lasts up to date where research is focused on the search for sustainable production of PHAs from renewable resources. Two parallel platforms are constantly bettered, one that searches for natural wild-type strains capable of efficient conversion of substrates to PHAs and the other that seeks to modify microorganisms genetically. The process optimizations are achieved through not only genetic manipulations but also via different fermentation process developments. These include novel strategies for batch, fed-batch, and continuous fermentation optimization with the aid of mathematical modeling of single or mixed microbial cultures. Different strategies for limiting inorganic nutrients are also applied. However, the most crucial issue addressed by research is the application of sustainable carbon sources for the production of renewable PHAs. Therefore, many processes are developed to be integrated into biorefineries as they envisage the use of primary or secondary substrates of existing biotechnologies.
The primary carbon sources for the production of PHA can be various types of vegetable oils such as soybean, coconut, palm, rapeseed, or rubber, which are characterized by low production costs (Kahar et al., 2004; Lee et al., 2008; Kynadi and Suchithra, 2017). The fatty acids also are seen as primary resources used for PHA production—they are obtained from vegetable oils. They include, among others, palmitic, stearic, oleic, linoleic, as well as α-linolenic, caproic, caprylic, and myristic acids (Kynadi and Suchithra, 2017). Employing these substrates for PHA production, one can obtain polymers such as P(3HB-co-3HV), P(3HB-co-3HHx), P(3HB), or mcl-PHAs (Kahar et al., 2004; Ng et al., 2011).
The substrates of the second generation include food, industrial, forestry, and timber waste as well as municipal sewage. Various food waste and food by-products can be used as raw materials for the biotechnological production of PHAs. Mixed food waste is characterized by high complexity and variety of physical properties, particle size, and composition, so pretreatment of such raw material is a necessary step (Nikodinovic-Runic et al., 2013). The second stage of the process of PHA production from food bio-wastes is to obtain a sufficient amount of bacterial biomass capable of production and maximum accumulation of PHA inside the cells. One of the approaches is the use of pure bacterial cultures, their multiplication to an appropriate amount of active biomass on media with easily assimilated carbon source, and their acclimatization before the third stage of the process (PHA synthesis) (Cardozo et al., 2016; Schmidt et al., 2016). The last step usually takes place in a different reactor than the second stage. Still, with a strategy using pure bacterial culture, the approach of PHA synthesis in the same reactor as biomass multiplication is also used, where the polymer synthesis is driven by inorganic nutrient limitation (Rodriguez-Perez et al., 2018).
Wastes with more unified characteristics are also used in the production of PHA. These are, for example, industrial wastes mainly from food production and processing plants (sugar factories, distilleries, slaughterhouses, dairies and cold stores, and oil mills). Others are plant waste from crops, which are generated by agriculture and represent a considerable biomass resource. Agricultural residues include stems, leaves, and seed pods, while process waste also includes peelings, husks, seeds, marc, roots, bark, and sawdust. All these wastes are rich in cellulose, starch, and other carbohydrates. Examples of processes for PHA production based on bacterial monocultures from these substrates are collected in Table 3. The substrates either are fermented directly (glycerol, fatty acids) or require appropriate preprocessing (hydrolysate production). For details, please see the following reviews: Nikodinovic-Runic et al. (2013); Koller (2017); Koller et al. (2017); Kourmentza et al. (2017); Blunt et al. (2018), and Favaro et al. (2019).
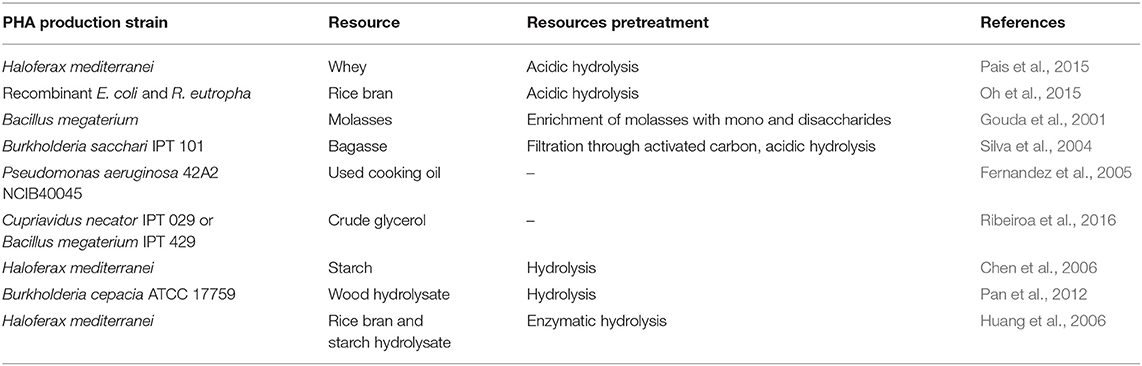
Table 3. Examples of secondary raw materials and processes for polyhydroxyalkanoate (PHA) production.
In recent years, there was an increasing number of reports published that provide LCA for PHA-based products and process (Pietrini et al., 2007; Cristóbal et al., 2015; Morgan-Sagastume et al., 2016b; Nitkiewicz et al., 2020). Mainly these studies point toward the implementation of waste streams (resources) for the production of biopolymers. Further, they highlight also that the selection of materials and, eventually, the final product also plays a crucial role in both environmental and economic outcomes of the whole process. Furthermore, the LCA analyses conclude that PHA polymers can find their right place in a biorefinery context, where their production can be carried out in parallel to other biotechnologies, while sourcing substrates from coexisting blocks' waste streams. In this way, there would be not only complemented with zero-waste policies but also upcycling of the generated wastes within biorefineries to high-value products.
Polyhydroxyalkanoate Nanobeads
Years 2005–2014
Inspired by the creation of PHA granules in vivo by microorganisms, a trend emerged that encompassed technologies based on spherical nanobeads. First, reports reached for the science behind simple nano/microbeads creation where these vehicles were prepared by emulsification. Thanks to PHA being hydrophobic in nature, it was proposed to mix by physical blending bioactive compounds to produce nano/micro-preparations for antimicrobial and anticancer treatments. Moreover, technologies have been adapted from polymer chemistry in order to functionalize PHA polymers by graft and/or block polymerization with other polymers [e.g., with polyethylene glycol (PEG), poly(ethylene imine) (PEI), or Jeffamine] or covalently bind drugs to the polymeric chains and further create nano/micro-carriers. Recently, hollow or highly open porous microspheres based on PHA biocompatible polymers were constructed as cell carriers for targeted therapies. Tapping into molecular biology and material design, a platform was created that enabled the creation of functionalized nano/microbeads in vitro and in vivo by enzymatic apparatus of microorganisms. Several works were performed where a genetically modified PHA synthase was used to prepare functionalized PHA beads in a test tube from PHA monomers that either carried a bioactive drug inside a granule or the granule itself was decorated with engineered protein. Finally, a very sophisticated approach was proposed, where microorganism itself through genetic manipulations became able to synthetize a native PHA granule decorated with proteins of interest through their conjugation to granule-associated proteins (phasins or PHA synthetases), enabling the use of such PHA beads in the creation of vaccines, new methods in imaging, bioseparations, or protein purification. More details on the above technologies can be found in the following reviews: Li and Loh (2017); Michalak et al. (2017); Wei et al. (2018), and Gonzalez-Miro et al. (2019).
Poly-(R)-3-Hydroxybutyrate Production by Genetically Modified Escherichia coli
Years 2006–2019
PHAs are polymerized by synthases, which use various hydroxyacyl-CoAs as substrates. The PHB production from E. coli has been attracting attention, even though E. coli does not produce PHB naturally. However, its recombinant engineering allowed for the synthesis of this biopolymer. In publications making up this trend, various E. coli strain modifications are examined to improve and optimize both the fermentation process and the final product itself. In the majority of the studies, stress is put on the modification of bacteria for processing cheap and readily available carbon sources such as substrates deriving from biomass (Nikel et al., 2006; Park et al., 2012; Yang et al., 2014; Favaro et al., 2019).
The first reports of E. coli targeted recombination date back to 1999 when the first attempt of modification of metabolic network on PHB biosynthesis in recombinant E. coli was performed. The genes responsible for PHB biosynthesis in R. eutropha were cloned in E. coli and subsequently sequenced and characterized (Shi et al., 1999). An important source of carbon and nitrogen for bioplastic producing bacteria can be waste and agricultural by-products such as whey and steep corn liquor. It has been presented that PHB can be efficiently produced by the recombinant strain grown aerobically on a laboratory scale bioreactor on a medium supplemented with the agroindustrial by-products (Nikel et al., 2006). A recombinant E. coli strain containing the PHA biosynthetic genes from an Azotobacter species was specially prepared for producing PHB from milk whey (Nikel et al., 2006). Genetic engineering of E. coli can be used for PHA accumulation at low cost and high productivity. The biosynthesis of PHAs containing 2HB monomer from glucose by metabolically engineered E. coli strains has been reported (Park et al., 2012). In 2013, the whole process of PHB production from bacterial strain was modeled and simulated. A detailed analysis of each nutrient was performed using response surface methodology (Heshiki, 2013). Modification of metabolic pathways can be used not only to improve the production of known biopolymers but also to program microorganisms to perform a variety of functions such as producing new materials with unique properties (Rahman, 2014). E. coli was also metabolically engineered to synthesize copolymers such as [P(3HB-co-3HV)] from glucose (Yang et al., 2014). Most of the studies in this trend focus on the applications of modified E. coli bacteria to reduce the cost of PHA production and the possibility of using cheap carbon and nitrogen sources in the fermentation process (Mezzolla et al., 2017; Favaro et al., 2019).
Polyhydroxyalkanoate Composites—Organic and Nonorganic Blends
Years 2010–2019
High price, limited processing capabilities, and poor mechanical properties of PHAs restrain their use for everyday products such as food packaging, disposable cutlery, or device enclosures (Cunha et al., 2016). Additionally, tailored biopolymer composites can play an important role in medical applications such as drug delivery systems, wound healing products, or surgical implant devices (Hufenus et al., 2012). Blending is a simple and effective approach for obtaining new polymeric materials with improved properties, and the drawbacks of the primary components can be eliminated. Typically, the mechanical properties of polymer blends can be easily tuned by varying the compositions of the blend and preparation conditions. Additionally, blends with biodegradable additives can enhance some of the PHA features, such as biodegradability and biocompatibility (Li et al., 2016). In studies included in this trend, several PHA blends were described.
PHA–PLA blends are the most common and the cheapest products for application replacing traditional, petroleum-based plastics (Long, 2009). Predominantly, reports described physical blending of scl-PHAs with PLA polymers which to some extent enhanced the single polymer properties. However, drawbacks such as high melting temperature and brittleness of these blends could be overcome by supplementing them with either mcl-PHAs or other bio-additives (Abdelwahab et al., 2012). This effect can also be achieved by blending Polycaprolactone (PCL) with scl-PHAs, which is an excellent option to improve the mechanical performance of both homopolymers (Visakh and Roy, 2015). Other biopolymers used to create blends with PHAs were cellulose (Zhang et al., 1997), starch (Visakh and Roy, 2015), and chitosan (Ikejima et al., 1999). These polymers have been shown to enhance the PHA properties by lowering the crystallinity of scl-PHAs and enhancing their biodegradability.
Creating blends with PHAs has gained popularity in current world trends. They serve both to reduce the cost of PHA production so that they can compete with petroleum-derived plastics and to improve their physical, chemical, or biological properties to expand the area of potential applications. Blending of PHA with other biodegradable polymers is particularly prevalent when it comes to the creation of novel materials suitable for specific applications.
Concluding Remarks and Future Perspective
The approach proposed in the study, which was based on the analysis of the full texts of articles, allowed to overcome the limitations of the traditional method based only on a systematic review of literature. The sample selected for analysis was divided into groups containing articles published in 5-years overlapping periods beginning in 1988 and ending in 2019. The analysis allowed to identify eight main areas in PHA research that governed the 31 years of discoveries. It should be noted that the indicated research groups do not exhaust all the conducted studies in the analyzed period. However, they present the research with the highest empowerment in identifiable scientific articles.
The most important outcome of the data-mining process was the identification of these research areas that are still trending in the scientific community and are of high probability to continue. Firstly, scientists try to understand genetics and biochemistry behind PHA synthesis. This leads to the identification of key enzymes responsible for PHA synthesis, thus to the creation of better production strains. Moreover, insight into PHA accumulation from a genetic perspective opens new routes for obtaining multipurpose bioactive granules. Secondly, it is visible from identified trends that a lot of work is being conducted on processing and modifications of PHA, leading to smart composites. These processes and products will lead directly to providing solutions to substitute petrochemical polymers and provide markets with a range of smart materials. Such an approach is also backed up by studies leading to the sustainable production of these biopolymers from renewable and cheap substrates, which directly can reduce their so far high production costs.
It needs to be emphasized that the indicated trends are to serve as a supportive tool for seeking further research directions, which should allow scientists to revise their research areas, improve the research process, and avoid duplication in studies, thereby increasing the efficiency of scientific work. Furthermore, it is vital to remember the given definition of a “trend.” Trends were discovered with restricting criterion of at least 10 papers published in each 5-years period. The criterion was adopted to allow the presentation of the most popular trends. That does not mean that other trends are less important. Moreover, those less popular trends may become leading ones in the future. As cited here, some works, both original or reviews, were published post the trend apogee. They presented either a summary of a specific research area or further narrowed and specialized research continuing from a particular trend. It is also not a foregone conclusion that some of the presented trends will reemerge or new ones will manifest from this broad research on microbial polyesters.
Data Availability Statement
The raw data supporting the conclusions of this article will be made available by the authors, without undue reservation.
Author Contributions
SW designed and performed the in silico study, grouped literature, arranged, and technically analyzed it. MG and TW searched and provided raw data. SW, MW, and MS wrote methodology and part of introduction and edited the manuscript. MG, TW, and AS performed thematic description of trends, wrote part of introduction, the result, and discussion sections. MW provided funding for in silico analysis part. MG gave idea for the study and provided funding.
Funding
This project has been financed by the Ministry of Science and Higher Education of Poland within the Regional Initiative of Excellence Program for 2019–2022, Project No. 021/RID/2018/19. Total financing: PLN 11,897,131.40. This project was also funded by the Polish National Centre for Research and Development, grant TechMatStrateg no. TECHMATSTRATEG2/407507/1/NCBR/2019.
Conflict of Interest
The authors declare that the research was conducted in the absence of any commercial or financial relationships that could be construed as a potential conflict of interest.
References
Abdelwahab, M. A., Flynn, A., Chiou, B., Sen, I. S., Orts, W., and Chiellini, E. (2012). Thermal, mechanical and morphological characterization of plasticized PLA-PHB blends. Polym. Degrad. Stab. 97, 1822–1828. doi: 10.1016/j.polymdegradstab.2012.05.036
Ananiadou, S., Rea, B., Okazaki, N., Procter, R., and Thomas, J. (2009). Supporting systematic reviews using text mining. Soc. Sci. Comput. Rev. 27, 509–523. doi: 10.1177/0894439309332293
Antoun, S., Grizzi, I., Lenz, R. W., and Fuller, R. C. (1991). Production of a chiral polyester byPseudomonas oleovorans grown with 5-phenyl-2,4-pentadienoic acid. Chirality 3, 492–494. doi: 10.1002/chir.530030615
Antwi Peprah, B., Ramsay, J. A., and Ramsay, B. A. (2018). Stabilization and characterization of carboxylated medium-chain-length poly(3-hydroxyalkanoate) nanosuspensions. Int. J. Biol. Macromol. 108, 902–908. doi: 10.1016/j.ijbiomac.2017.10.181
Arkin, A. H., and Hazer, B. (2002). Chemical modification of chlorinated microbial polyesters. Biomacromolecules 3, 1327–1335. doi: 10.1021/bm020079v
Arkin, A. H., Hazer, B., and Borcakli, M. (2000). Chlorination of poly(3-hydroxy alkanoates) containing unsaturated side chains. Macromolecules 33, 3219–3223. doi: 10.1021/ma991535j
Ashby, R. D., Cromwick, A. M., and Foglia, T. A. (1998). Radiation crosslinking of a bacterial medium-chain-length poly(hydroxyalkanoate) elastomer from tallow. Int. J. Biol. Macromol. 23, 61–72. doi: 10.1016/S0141-8130(98)00034-8
Bassas-Galià, M., Gonzalez, A., Micaux, F., Gaillard, V., Piantini, U., Schintke, S., et al. (2015). Chemical Modification of Polyhydroxyalkanoates (PHAs) for the Preparation of Hybrid Biomaterials. Chim. Int. J. Chem. 69, 627–630. doi: 10.2533/chimia.2015.627
Bear, M. M., Leboucher-Durand, M. A., Langlois, V., Lenz, R. W., Goodwin, S., and Guérin, P. (1997). Bacterial poly-3-hydroxyalkenoates with epoxy groups in the side chains. React. Funct. Polym. 34, 65–77. doi: 10.1016/S1381-5148(97)00024-2
Blake, C. (2010). Beyond genes, proteins, and abstracts: identifying scientific claims from full-text biomedical articles. J. Biomed. Inform. 43, 173–189. doi: 10.1016/j.jbi.2009.11.001
Blunt, W., Levin, D., and Cicek, N. (2018). Bioreactor Operating Strategies for Improved Polyhydroxyalkanoate (PHA) Productivity. Polymers (Basel). 10:1197. doi: 10.3390/polym10111197
Bordes, P., Pollet, E., Averous, L., and Avérous, L. (2009). Nano-biocomposites: biodegradable polyester/nanoclay systems. Prog. Polym. Sci. 34, 125–155. doi: 10.1016/j.progpolymsci.2008.10.002
Breuer, U., Terentiev, Y., Kunze, G., and Babel, W. (2002). Yeasts as producers of polyhydroxyalkanoates: genetic engineering of saccharomyces cerevisiae. Macromol. Biosci. 2, 380–386. doi: 10.1002/1616-5195(200211)2:8<380::AID-MABI380>3.0.CO;2-X
Cardozo, J. R. G., Martínez, A. L. M., Pérez, M. Y., and Londoño, G. A. C. (2016). Production and characterization of polyhydroxyalkanoates and native microorganisms synthesized from fatty waste. Int. J. Polymer Sci. 2016, 1–12. doi: 10.1155/2016/6541718
Cavaillé, L., Grousseau, E., Pocquet, M., Lepeuple, A. S., Uribelarrea, J. L., Hernandez-Raquet, G., et al. (2013). Polyhydroxybutyrate production by direct use of waste activated sludge in phosphorus-limited fed-batch culture. Bioresour. Technol. 149, 301–309. doi: 10.1016/j.biortech.2013.09.044
Chen, C. W., Don, T., and Yen, H. (2006). Enzymatic extruded starch as a carbon source for the production of poly (3-hydroxybutyrate-co-3-hydroxyvalerate) by Haloferax mediterranei. 41, 2289–2296. doi: 10.1016/j.procbio.2006.05.026
Chen, G.-Q., and Wu, Q. (2005). The application of polyhydroxyalkanoates as tissue engineering materials. Biomaterials 26, 6565–6578. doi: 10.1016/j.biomaterials.2005.04.036
Chen, Y., Hung, S.-T., Chou, E., and Wu, H.-S. (2017). Review of polyhydroxyalkanoates materials and other biopolymers for medical applications. Mini. Rev. Org. Chem. 15, 105–121. doi: 10.2174/1570193X14666170721153036
Cheng, H. N., Gross, R. A., and Smith, P. B. (eds.). (2013). Green Polymer Chemistry: Biocatalysis and Materials II. Washington, DC: American Chemical Society. doi: 10.1021/bk-2013-1144
Cichoń, E., Harazna, K., Skibiński, S., Witko, T., and Zima, A. (2019). Novel bioresorbable tricalcium phosphate / polyhydroxyoctanoate (TCP / PHO) composites as scaffolds for bone tissue engineering applications Journal of the Mechanical Behavior of Biomedical Materials Novel bioresorbable tricalcium phosphate / polyhydrox. J. Mech. Behav. Biomed. Mater. 98, 235–245. doi: 10.1016/j.jmbbm.2019.06.028
Cong, Y., Chan, Y. B., and Ragan, M. A. (2016). A novel alignment-free method for detection of lateral genetic transfer based on TF-IDF. Sci. Rep. 6:303038. doi: 10.1038/srep30308
Cristóbal, J., Matos, C. T., Aurambout, J. P., Manfredi, S., and Kavalov, B. (2015). Environmental sustainability assessment of bioeconomy value chains. Biomass Bioenergy 89, 159–171. doi: 10.1016/j.biombioe.2016.02.002
Cunha, M., Marie-Alix, B., and Pereira, P. (2016). Polyvinyl alcohol-modified pithecellobium clypearia benth herbal residue fiberpolypropylene composites. Polym. Compos. 37, 915–924. doi: 10.1002/pc.23250
Dagnon, K. L., Chen, H. H., Innocentini-Mei, L. H., and D'Souza, N. A. (2009). Poly[(3-hydroxybutyrate)-co-(3-hydroxyvalerate)]/layered double hydroxide nanocomposites. Polym. Int. 58, 133–141. doi: 10.1002/pi.2503
Deng, Y., Lin, X. S., Zheng, Z., Deng, J. G., Chen, J. C., Ma, H., et al. (2003). Poly(hydroxybutyrate-co-hydroxyhexanoate) promoted production of extracellular matrix of articular cartilage chondrocytes in vitro. Biomaterials 24, 4273–4281. doi: 10.1016/S0142-9612(03)00367-3
Dinjaski, N., and Prieto, M. A. (2015). Smart polyhydroxyalkanoate nanobeads by protein based functionalization. Nanomed. Nanotechnol. Biol. Med. 11, 885–899. doi: 10.1016/j.nano.2015.01.018
Elmowafy, E., Abdal-Hay, A., Skouras, A., Tiboni, M., Casettari, L., and Guarino, V. (2019). Polyhydroxyalkanoate (PHA): applications in drug delivery and tissue engineering. Expert Rev. Med. Devices 16, 467–482. doi: 10.1080/17434440.2019.1615439
Favaro, L., Basaglia, M., and Casella, S. (2019). Improving polyhydroxyalkanoate production from inexpensive carbon sources by genetic approaches: a review. Biofuels. Bioprod. Biorefining 13, 208–227. doi: 10.1002/bbb.1944
Fernandez, D., Rodriguez, E., Bassas, M., Vioas, M., Solanas, A. M., Llorens, J., et al. (2005). Agro-industrial oily wastes as substrates for PHA production by the new strain Pseudomonas aeruginosa NCIB 40045: effect of culture conditions. Biochem. Eng. J. 26, 159–167. doi: 10.1016/j.bej.2005.04.022
Fritzsche, K., Lenz, R. W., and Fuller, R. C. (1990). An unusual bacterial polyester with a phenyl pendant group. Makromol. Chem 191, 1957–1965. doi: 10.1002/macp.1990.021910821
Gonzalez-Miro, M., Chen, S., Gonzaga, Z. J., Evert, B., Wibowo, D., and Rehm, B. H. A. (2019). Polyester as antigen carrier toward particulate vaccines. Biomacromolecules 20, 3213–3232. doi: 10.1021/acs.biomac.9b00509
Gouda, M. K., Swellam, A. E., and Omar, S. H. (2001). Production of PHB by a Bacillus megaterium strain using sugarcane molasses and corn steep liquor as sole carbon and nitrogen sources. Microbiol. Res. 156, 201–207. doi: 10.1078/0944-5013-00104
Grigore, M. E., Grigorescu, R. M., Iancu, L., Ion, R. M., Zaharia, C., and Andrei, E. R. (2019). Methods of synthesis, properties and biomedical applications of polyhydroxyalkanoates: a review. J. Biomater. Sci. Polym. Ed. 30, 695–712. doi: 10.1080/09205063.2019.1605866
Gumel, A. M., Annuar, S. M., and Heidelberg, T. (2013). Single-step lipase-catalyzed functionalization of medium-chain-length polyhydroxyalkanoates. J. Chem. Technol. Biotechnol. 88, 1328–1335. doi: 10.1002/jctb.3980
Gursel, I., Yagmurlu, F., Korkusuz, F., and Hasirci, V. (2002). In vitro antibiotic release from poly(3-hydroxybutyrate-co-3-hydroxyvalerate) rods. J. Microencapsul. 19, 153–164. doi: 10.1080/02652040110065413
Hazer, B. (2010). Amphiphilic Poly(3-hydroxy alkanoate)s: potential candidates for medical applications. Int. J. Polym. Sci. 2010, 1–8. doi: 10.1155/2010/423460
Hazer, B., and Steinbüchel, A. (2007). Increased diversification of polyhydroxyalkanoates by modification reactions for industrial and medical applications. Appl Microbiol Biotechnol 74, 1–12. doi: 10.1007/s00253-006-0732-8
Heshiki, Y. (2013). Optimization of Polyhydroxybutyrate Production in Recombinant Escherichia coli Through Metabolic Modeling and Simulation. All Graduate Plan B and other Reports. Logan, UT: Utah State University, 291. Available online at: https://digitalcommons.usu.edu/gradreports/291
Hou, T., Zhang, J. Z., Kong, L. J., Zhang, X. F., Hu, P., Zhang, D. M., et al. (2006). Morphologies of fibroblast cells cultured on surfaces of PHB films implanted by hydroxyl ions. J. Biomater. Sci. Polym. Ed. 17, 735–746. doi: 10.1163/156856206777656508
Huang, T., Duan, K., Huang, S., and Will Chen, C. (2006). Production of polyhydroxyalkanoates from inexpensive extruded rice bran and starch by Haloferax mediterranei. J. Ind. Microbiol. Biotechnol. 33, 701–706. doi: 10.1007/s10295-006-0098-z
Hufenus, R., Reifler, F. A., Maniura-Weber, K., Spierings, A., and Zinn, M. (2012). Biodegradable bicomponent fibers from renewable sources: melt-spinning of poly(lactic acid) and poly[(3-hydroxybutyrate)-co-(3-hydroxyvalerate)]. Macromol. Mater. Eng. 297, 75–84. doi: 10.1002/mame.201100063
Ikejima, T., Yagi, K., and Inoue, Y. (1999). Thermal properties and crystallization behavior of poly(3-hydroxybutyric acid) in blends with chitin and chitosan. Macromol. Chem. Phys. 200, 413–421. doi: 10.1002/(SICI)1521-3935(19990201)200:2<413::AID-MACP413>3.0.CO;2-Q
Inoue, D., Suzuki, Y., Uchida, T., Morohoshi, J., and Sei, K. (2016). Polyhydroxyalkanoate production potential of heterotrophic bacteria in activated sludge. J. Biosci. Bioeng. 121, 47–51. doi: 10.1016/j.jbiosc.2015.04.022
Ishii-Hyakutake, M., Mizuno, S., and Tsuge, T. (2018). Biosynthesis and characteristics of aromatic polyhydroxyalkanoates. Polymers (Basel). 10, 1–24. doi: 10.3390/polym10111267
Jendrossek, D. (1998). Microbial degradation of polyesters: a review on extracellular poly(hydroxy alkanoic acid) depolymerases. Polym. Degrad. Stab. 59, 317–325. doi: 10.1016/S0141-3910(97)00190-0
Jones, E., Oliphant, T., and Peterson, P. (2001). SciPy: Open Source Scientific Tools for Python. Available online at: http://www.scipy.org
Kahar, P., Tsuge, T., Taguchi, K., and Doi, Y. (2004). High yield production of polyhydroxyalkanoates from soybean oil by Ralstonia eutropha and its recombinant strain. Polymer Degrad. Stab. 83, 79–86. doi: 10.1016/S0141-3910(03)00227-1
Khardenavis, A., Guha, P. K., Kumar, M. S., Mudliar, S. N., and Chakrabarti, T. (2005). Activated sludge is a potential source for production of biodegradable plastics from wastewater. Environ. Technol. 26, 545–552. doi: 10.1080/09593332608618536
Kim, H. W., Chung, C. W., and Rhee, Y. H. (2005). UV-induced graft copolymerization of monoacrylate-poly(ethylene glycol) onto poly(3-hydroxyoctanoate) to reduce protein adsorption and platelet adhesion. Int. J. Biol. Macromol. 35, 47–53. doi: 10.1016/j.ijbiomac.2004.11.007
Koller, M. (2017). Production of Polyhydroxyalkanoate (PHA) Biopolyesters by Extremophiles? MOJ Polym. Sci. 1, 69–85. doi: 10.15406/mojps.2017.01.00011
Koller, M., Maršálek, L., de Sousa Dias, M. M., and Braunegg, G. (2017). Producing microbial polyhydroxyalkanoate (PHA) biopolyesters in a sustainable manner. N. Biotechnol. 37, 24–38. doi: 10.1016/j.nbt.2016.05.001
Kourmentza, C., Plácido, J., Venetsaneas, N., Burniol-Figols, A., Varrone, C., Gavala, H. N., et al. (2017). Recent Advances and Challenges towards Sustainable Polyhydroxyalkanoate (PHA) Production. Bioengineering 4:55. doi: 10.3390/bioengineering4020055
Kwiecień, I., Radecka, I., Kowalczuk, M., and Adamus, G. (2015). Transesterification of PHA to oligomers covalently bonded with (bio)active compounds containing either carboxyl or hydroxyl functionalities. PLoS ONE 10:e0120149. doi: 10.1371/journal.pone.0120149
Kwon, O. H., Lee, I. S., Ko, Y.-G., Meng, W., Jung, K.-H., Kang, I.-K., et al. (2007). Electrospinning of microbial polyester for cell culture. Biomed. Mater. 2, S52–S58. doi: 10.1088/1748-6041/2/1/S08
Kynadi, A. S., and Suchithra, T. V. (2017). Industrial Crops & Products Formulation and optimization of a novel media comprising rubber seed oil for PHA production. Ind. Crop. Prod. 105, 156–163. doi: 10.1016/j.indcrop.2017.04.062
Lee, M. Y., Cha, S. Y., and Park, W. H. (1999). Crosslinking of microbial copolyesters with pendant epoxide groups by diamine. Polymer (Guildf). 40, 3787–3793. doi: 10.1016/S0032-3861(98)00612-0
Lee, W. H., Loo, C. Y., Nomura, C. T., and Sudesh, K. (2008). Biosynthesis of polyhydroxyalkanoate copolymers from mixtures of plant oils and 3-hydroxyvalerate precursors. Bioresour. Technol. 99, 6844–6851. doi: 10.1016/j.biortech.2008.01.051
Li, Z., and Loh, X. J. (2017). Recent advances of using polyhydroxyalkanoate-based nanovehicles as therapeutic delivery carriers. Wiley Interdiscip. Rev. Nanomed. Nanobiotechnol. 9:e1429. doi: 10.1002/wnan.1429
Li, Z., Yang, J., and Loh, X. J. (2016). Polyhydroxyalkanoates: opening doors for a sustainable future. NPG Asia Mater. 8, e265–e220. doi: 10.1038/am.2016.48
Lizarraga, L., Thomas, C., Juan Ignacio, C.-M., and Roy, I. (2018). “Tissue engineering: polyhydroxyalkanoate-based materials and composites,” in Encyclopedia of Polymer Applications (Boca Raton, FL: CRC Press Taylor& Francis Group). doi: 10.1201/9781351019422-140000458
Long, Y. (2009). Biodegradable Polymer Blends and Composites from Renewable Resources. 1st ed., New Jersey, NY: John Wiley and Sons, Inc.
Lopez, P. (2009). “GROBID: combining automatic bibliographic data recognition and term extraction for scholarship publications,” in Proceedings of the 13th European Conference on Digital Library (ECDL), (Corfu). doi: 10.1007/978-3-642-04346-8_62
Lu, J., Tappel, R. C., and Nomura, C. T. (2009). Mini-review: biosynthesis of poly(hydroxyalkanoates). Polym. Rev. 49, 226–248. doi: 10.1080/15583720903048243
Maestro, B., and Sanz, J. M. (2017). Polyhydroxyalkanoate-associated phasins as phylogenetically heterogeneous, multipurpose proteins. Microb. Biotechnol. 10, 1323–1337. doi: 10.1111/1751-7915.12718
McInnes, L., Healy, J., and Astels, S. (2017). hdbscan: hierarchical density based clustering. J. Open Source Softw. 2:205. doi: 10.21105/joss.00205
Mezzolla, V., D'Urso, O., and Polotronieri, P. (2017). Optimization of polyhydroxyalkanoate production by recombinant E. coli supplemented with different plant by-products. Biotechnol. An Indian J. 13, 1–17. Available online at: https://www.tsijournals.com/articles/optimization-of-polyhydroxyalkanoate-production-by-recombinant-e-coli-supplemented-with-different-plant-byproducts.html
Michalak, M., Kurcok, P., and Hakkarainen, M. (2017). Polyhydroxyalkanoate-based drug delivery systems. Polym. Int. 66, 617–622. doi: 10.1002/pi.5282
Mihalcea, R., Corley, C., and Strapparava, C. (2006). “Corpus-based and knowledge-based measures of text semantic similarity.” in AAAI'06 Proceedings of the 21st national conference on Artificial intelligence, ed A. Cohn, Vol. 1 (Boston, MA: AAAI Press), 775–780.
Mihara, C., Yano, T., Kozaki, S., Honma, T, Kenmoku, T., Fukui, T., et al. (2006). Polyhydroxyalkanoate Containing Amide Group, Sulfonic Group, and Sulfonate Ester Group, Method for Producing the Same, and Charge Control Agent, Toner, Image Forming Method, and Image Forming Apparatus. EP1579277B1. Available online at: https://patents.google.com/patent/EP1579277B1/nl
Misra, S. K., Ohashi, F., Valappil, S. P., Knowles, J. C., Roy, I., Silva, S. R. P., et al. (2010). Characterization of carbon nanotube (MWCNT) containing P(3HB)/bioactive glass composites for tissue engineering applications. Acta Biomater. 6, 735–742. doi: 10.1016/j.actbio.2009.09.023
Misra, S. K., Watts, P. C. P., Valappil, S. P., Silva, S. R. P., Roy, I., and Boccaccini, A. R. (2007). Poly(3- hydroxybutyrate)/Bioglass ® composite films containing carbon nanotubes. Nanotechnology 18:075701. doi: 10.1088/0957-4484/18/7/075701
Mizuno, S., Enda, Y., Saika, A., Hiroe, A., and Tsuge, T. (2018). Biosynthesis of polyhydroxyalkanoates containing 2-hydroxy-4-methylvalerate and 2-hydroxy-3-phenylpropionate units from a related or unrelated carbon source. J. Biosci. Bioeng. 125, 295–300. doi: 10.1016/j.jbiosc.2017.10.010
Mizuno, S., Hiroe, A., Fukui, T., Abe, H., and Tsuge, T. (2017). Fractionation and thermal characteristics of biosynthesized polyhydoxyalkanoates bearing aromatic groups as side chains. Polym. J. 49, 557–565. doi: 10.1038/pj.2017.20
Morgan-Sagastume, F., Heimersson, S., Laera, G., Werker, A., and Svanström, M. (2016a). Techno-environmental assessment of integrating polyhydroxyalkanoate (PHA) production with services of municipal wastewater treatment. J. Clean. Prod. 137, 1368–1381. doi: 10.1016/j.jclepro.2016.08.008
Morgan-Sagastume, F., Heimersson, S., Laera, G., Werker, A., and Svanström, M. (2016b). Techno-environmental assessment of integrating polyhydroxyalkanoate (PHA) production with services of municipal wastewater treatment. J. Clean. Prod. 137, 1368–1381.
Ng, K., Wong, Y., Tsuge, T., and Sudesh, K. (2011). Biosynthesis and characterization of poly (3-hydroxybutyrate- co−3-hydroxyvalerate) and poly (3-hydroxybutyrate- co−3-hydroxyhexanoate) copolymers using jatropha oil as the main carbon source. Process Biochem. 46, 1572–1578. doi: 10.1016/j.procbio.2011.04.012
Nikel, P. I., De Almeida, A., Melillo, E. C., Galvagno, M. A., and Pettinari, M. J. (2006). New recombinant Escherichia coli strain tailored for the production of poly(3-hydroxybutyrate) from agroindustrial by-products. Appl. Environ. Microbiol. 72, 3949–3954. doi: 10.1128/AEM.00044-06
Nikodinovic-Runic, J., Guzik, M., Kenny, S. T., Babu, R., Werker, A., and O'Connor, K. E. (2013). “Carbon-rich wastes as feedstocks for biodegradable polymer (polyhydroxyalkanoate) production using bacteria,” in Advances in Applied Microbiology, eds S. Sariaslani and G. M. Gadd (Academic Press Inc.), 139–200. doi: 10.1016/B978-0-12-407673-0.00004-7
Nitkiewicz, T., Wojnarowska, M., Soltysik, M., Kaczmarski, A., Witko, T, Ingrao, C., et al. (2020). How sustainable are biopolymers? Findings from a life cycle assessment of polyhydroxyalkanoate production from rapeseed-oil derivatives. Sci. Total Environ. 749:141279. doi: 10.1016/j.scitotenv.2020.141279
Nomura, C. T., and Taguchi, S. (2007). {PHA} synthase engineering toward superbiocatalysts for custom-made biopolymers. Appl. Microbiol. Biotechnol. 73, 969–979. doi: 10.1007/s00253-006-0566-4
Oh, Y. H., Lee, S. H., Jang, Y.-A., Choi, J. W., Hong, K. S., Yu, J. H., et al. (2015). Development of rice bran treatment process and its use for the synthesis of polyhydroxyalkanoates from rice bran hydrolysate solution. Bioresour. Technol. 181, 283–290. doi: 10.1016/j.biortech.2015.01.075
Olivera, E. R., Carnicero, D., Jodra, R., Minambres, B., Garcia, B., Abraham, G. A., et al. (2001). Genetically engineered Pseudomonas: a factory of new bioplastics with broad applications. Environ. Microbiol. 3, 612–618. doi: 10.1046/j.1462-2920.2001.00224.x
Ong, S. Y., Chee, Y., Sudesh, K., and Yee, J. (2017). Degradation of polyhydroxyalkanoate (PHA): a review. J. Sib. Fed. Univ. Biol. 10, 211–225. doi: 10.17516/1997-1389-0024
Pais, J., Serafim, L. S., Freitas, F., and Reis, M. A. M. (2015). Conversion of cheese whey into poly (3-hydroxybutyrate- co−3- hydroxyvalerate) by Haloferax mediterranei. N. Biotechnol. 33, 224–230. doi: 10.1016/j.nbt.2015.06.001
Pan, W., Perrotta, J. A., Stipanovic, A. J., Nomura, C. T., and Nakas, J. P. (2012). Production of polyhydroxyalkanoates by Burkholderia cepacia ATCC 17759 using a detoxified sugar maple hemicellulosic hydrolysate. J. Ind. Microbiol. Biotechnol. 39, 459–469. doi: 10.1007/s10295-011-1040-6
Pandey, J. K., Raghunatha Reddy, K., Pratheep Kumar, A., and Singh, R. P. (2005). An overview on the degradability of polymer nanocomposites. Polym. Degrad. Stab. 88, 234–250. doi: 10.1016/j.polymdegradstab.2004.09.013
Park, S. J., Lee, T. W., Lim, S.-C. C., Kim, T. W., Lee, H., Kim, M. K., et al. (2012). Biosynthesis of polyhydroxyalkanoates containing 2-hydroxybutyrate from unrelated carbon source by metabolically engineered Escherichia coli. Appl. Microbiol. Biotechnol. 93, 273–283. doi: 10.1007/s00253-011-3530-x
Park, W. H., Lenz, R. W., and Goodwin, S. (1999). Epoxidation of bacterial polyesters with unsaturated side chains: IV. Thermal degradation of initial and epoxidized polymers. Polym. Degrad. Stab. 63, 287–291. doi: 10.1016/S0141-3910(98)00107-4
Patel, M., Gapes, D. J., Newman, R. H., and Dare, P. H. (2009). Physico-chemical properties of polyhydroxyalkanoate produced by mixed-culture nitrogen-fixing bacteria. Appl. Microbiol. Biotechnol. 82, 545–555. doi: 10.1007/s00253-008-1836-0
Pedregosa, F., Michel, V., Grisel, O., Blondel, M., Prettenhofer, P., Weiss, R., et al. (2011). Scikit-learn: Machine Learning in Python. Available online at: http://scikit-learn.sourceforge.net (accessed December 5, 2019).
Pietrini, M., Roes, L., Patel, M. K., and Chiellini, E. (2007). Comparative life cycle studies on poly(3-hydroxybutyrate)-based composites as potential replacement for conventional petrochemical plastics. Biomacromolecules 8, 2210–2218. doi: 10.1021/bm0700892
Pittmann, T., and Steinmetz, H. (2014). Polyhydroxyalkanoate production as a side stream process on a municipal waste water treatment plant. Bioresour. Technol. 167, 297–302. doi: 10.1016/j.biortech.2014.06.037
Pittmann, T., and Steinmetz, H. (2017). Polyhydroxyalkanoate production on waste water treatment plants: process scheme, operating conditions and potential analysis for german and european municipal waste water treatment plants. Bioengineering 4:54. doi: 10.3390/bioengineering4020054
Porter, M. M., Lee, S., Tanadchangsaeng, N., Jaremko, M. J., Yu, J., Meyers, M., et al. (2013). Mechanics of biological systems and materials, volume 6. Mech. Biol. Syst. Mater. 5, 63–71. doi: 10.1007/978-1-4614-4427-5_10
Qin, Q., Ling, C., Zhao, Y., Yang, T., Yin, J., Guo, Y., et al. (2018). CRISPR/Cas9 editing genome of extremophile Halomonas spp. Metab. Eng. 47, 219–229. doi: 10.1016/j.ymben.2018.03.018
Qu, B., and Liu, J. X. (2009). Determination of optimum operating conditions for production of polyhydroxybutyrate by activated sludge submitted to dynamic feeding regime. Chinese Sci. Bull. 54, 142–149. doi: 10.1007/s11434-008-0566-0
Rahman, A. (2014). Sustainable production of novel biomaterials in Escherichia coli (All Graduate Theses and Dissertations), Utah State University, Logan, UT, United States, 3874. Available online at: https://digitalcommons.usu.edu/etd/3874
Ribeiroa, P. L. L., de Souza Silvab, G., and Druzian, J. I. (2016). Evaluation of the effects of crude glycerol on the production and properties of novel polyhydroxyalkanoate copolymers containing high 11-hydroxyoctadecanoate by Cupriavidus necator IPT 029 and Bacillus megaterium IPT 429. Polymer Adv. Tech. 27, 542–549. doi: 10.1002/pat.3725
Rodriguez-Perez, S., Serrano, A., Pantión, A. A., and Alonso-Fariñas, B. (2018). Challenges of scaling-up PHA production from waste streams. A review. J. Environ. Manage. 205, 215–230. doi: 10.1016/j.jenvman.2017.09.083
Salton, G., and Yang, C. S. (1973). On the Specification of Term Values in Automatic Indexing. Ithaca, NY: Cornell University. doi: 10.1108/eb026562
Samsuddin, F. M., Benjamin, R. L., Sriram, Y., Shant, A., and Dennis, W. S. (2013). Green Polymer Chemistry: Biocatalysis and Materials II, Vol. 1144. American Chemical Society, 291–301.
Sato, S., Ono, Y., Mochiyama, Y., Sivaniah, E., Kikkawa, Y., Sudesh, K., et al. (2008). Polyhydroxyalkanoate film formation and synthase activity during in vitro and in situ polymerization on hydrophobic surfaces. Biomacromolecules 9, 2811–2818. doi: 10.1021/bm800566s
Satoh, H., Iwamoto, Y., Mino, T., and Matsuo, T. (1998). Activated sludge as a possible source of biodegradable plastic. Water Sci. Technol. 38, 103–109. doi: 10.2166/wst.1998.0115
Schmidt, M., Lutz, J., Kelin, L., Zanfonato, K., Schmidell, W., Maria, G., et al. (2016). Poly (3-hydroxybutyrate- co−3-hydroxyvalerate) production in a system with external cell recycle and limited nitrogen feeding during the production phase. Biochem. Eng. J. 112, 130–135. doi: 10.1016/j.bej.2016.04.013
Schubert, P., Steinbuchel, A., and Schlegel, H. (1988). Cloning of the Alcaligenes eutrophus genes for synthesis of poly-beta-hydroxybutyric acid (PHB) and synthesis of PHB in Escherichia coli. J. Bacteriol. 170, 5837–5847. doi: 10.1128/JB.170.12.5837-5847.1988
Shi, H., Nikawa, J., and Shimizu, K. (1999). Effect of modifying metabolic network on poly-3-hydroxybutyrate biosynthesis in recombinant Escherichia coli. J. Biosci. Bioeng. 87, 666–677. doi: 10.1016/S1389-1723(99)80132-7
Shrivastav, A., Kim, H.-Y., and Kim, Y.-R. (2013). Advances in the applications of polyhydroxyalkanoate nanoparticles for novel drug delivery system. Biomed Res. Int. 2013, 1–12. doi: 10.1155/2013/581684
Silva, L. F., Taciro, M. K., Ramos, M. E. M., Carter, J. M., Pradella, J. G. C., and Gomez, J. G. C. (2004). Poly-3-hydroxybutyrate (P3HB) production by bacteria from xylose, glucose and sugarcane bagasse hydrolysate. J. Ind. Microbiol. Biotechnol. 31, 245–254. doi: 10.1007/s10295-004-0136-7
Slater, S., Voige, W., and Dennis, D. (1988). Cloning and expression in Escherichia coli of the Alcaligenes eutrophus H16 poly-β-hydroxybutyrate biosynthetic pathway. J. Bacteriol. 170, 4431–4436. doi: 10.1128/JB.170.10.4431-4436.1988
Steinbüchel, A., Valentin, H. E., Steinbuchel, A., and Valentin, H. E. (1995). Diversity of bacterial polyhydroxyalkanoic acids. FEMS Microbiol. Lett. 128, 219–228. doi: 10.1016/0378-1097(95)00125-O
Stubbe, J., and Tian, J. (2003). Polyhydroxyalkanoate (PHA) hemeostasis: the role of PHA synthase. Nat. Prod. Rep. 20, 445–457. doi: 10.1039/b209687k
Suriyamongkol, P., Weselake, R., Narine, S., Moloney, M., and Shah, S. (2007). Biotechnological approaches for the production of polyhydroxyalkanoates in microorganisms and plants: a review. Biotechnol. Adv. 25, 148–175. doi: 10.1016/j.biotechadv.2006.11.007
Takagi, Y., Yasuda, R., Maehara, A., and Yamane, T. (2004). Microbial synthesis and characterization of polyhydroxyalkanoates with fluorinated phenoxy side groups from Pseudomonas putida. Eur. Polym. J. 40, 1551–1557. doi: 10.1016/j.eurpolymj.2004.01.030
Tezcaner, A., Bugra, K., and Hasirci, V. (2003). Retinal pigment epithelium cell culture on surface modified poly(hydroxybutyrate-co-hydroxyvalerate) thin films. Biomaterials 24, 4573–4583. doi: 10.1016/S0142-9612(03)00302-8
Tranfield, D., Denyer, D., and Smart, P. (2003). Towards a methodology for developing evidence-informed management knowledge by means of systematic review*. Br. J. Manag. 14, 207–222. doi: 10.1111/1467-8551.00375
Valappil, S. P., Misra, S. K., Boccaccini, A. R., and Roy, I. (2006). Biomedical applications of polyhydroxyalkanoates, an overview of animal testing and in vivo responses. Expert Rev. Med. Devices 3, 853–868. doi: 10.1586/17434440.3.6.853
Valentino, F., Moretto, G., Lorini, L., Bolzonella, D., Pavan, P., and Majone, M. (2019). Pilot-scale polyhydroxyalkanoate production from combined treatment of organic fraction of municipal solid waste and sewage sludge. Ind. Eng. Chem. Res. 58, 12149–12158. doi: 10.1021/acs.iecr.9b01831
Valentino, F., Morgan-Sagastume, F., Fraraccio, S., Corsi, G., Zanaroli, G., Werker, A., et al. (2015). Sludge minimization in municipal wastewater treatment by polyhydroxyalkanoate (PHA) production. Environ. Sci. Pollut. Res. 22, 7281–7294. doi: 10.1007/s11356-014-3268-y
van der Walle, G. A. M., de Koning, G. J. M., Weusthuis, R. A., and Eggink, G. (2001). Properties, modifications and applications of biopolyesters. Adv. Biochem. Eng. Biotechnol. 71, 264–291. doi: 10.1007/3-540-40021-4_9
Visakh, P., and Roy, I. (2015). Polyhydroxyalkanoate (PHA) Based Blends, Composites and Nanocomposites. doi: 10.1002/9781118831328
Wallen, L. L., and Rohwedder, W. K. (1974). Poly-.β.-hydroxyalkanoate from Activated Sludge. Environ. Sci. Technol. 8, 576–579. doi: 10.1021/es60091a007
Wang, Y. W., Wu, Q., and Chen, G. Q. (2003a). Reduced mouse fibroblast cell growth by increased hydrophilicity of microbial polyhydroxyalkanoates via hyaluronan coating. Biomaterials 24, 4621–4629. doi: 10.1016/S0142-9612(03)00356-9
Wang, Z., Itoh, Y., Hosaka, Y., Kobayashi, I., Nakano, Y., Maeda, I., et al. (2003b). Mechanism of enhancement effect of dendrimer on transdermal drug permeation through polyhydroxyalkanoate matrix. J. Biosci. Bioeng. 96, 537–540. doi: 10.1016/S1389-1723(04)70146-2
Wei, D.-X., Dao, J.-W., and Chen, G.-Q. (2018). A micro-ark for cells: highly open porous polyhydroxyalkanoate microspheres as injectable scaffolds for tissue regeneration. Adv. Mater. 30:1802273. doi: 10.1002/adma.201802273
Williams, S. F., Martin, D. P., and Moses, A. C. (2016). The history of GalaFLEX P4HB scaffold. Aesthetic Surg. J. 36, S33–S42. doi: 10.1093/asj/sjw141
Yang, C., Zhang, W., Liu, R., Zhang, C., Gong, T., Li, Q., et al. (2013). Analysis of polyhydroxyalkanoate (PHA) synthase gene and PHA-producing bacteria in activated sludge that produces PHA containing 3-hydroxydodecanoate. FEMS Microbiol. Lett. 346, 56–64. doi: 10.1111/1574-6968.12201
Yang, J. E., Choi, Y. J., Lee, S. H. J. S. Y., Kang, K.-H. H., Lee, H., Oh, Y. H., et al. (2014). Metabolic engineering of Escherichia coli for biosynthesis of poly(3-hydroxybutyrate-co-3-hydroxyvalerate) from glucose. Appl. Microbiol. Biotechnol. 98, 95–104. doi: 10.1007/s00253-013-5285-z
Yang, J. E., Park, S. J., Kim, W. J., Kim, H. J., Kim, B. J., Lee, H., et al. (2018). One-step fermentative production of aromatic polyesters from glucose by metabolically engineered Escherichia coli strains. Nat. Commun. 9:79. doi: 10.1038/s41467-017-02498-w
Yu, H. Y., Qin, Z. Y., and Zhou, Z. (2011). Cellulose nanocrystals as green fillers to improve crystallization and hydrophilic property of poly(3-hydroxybutyrate-co-3-hydroxyvalerate). Prog. Nat. Sci. Mater. Int. 21, 478–484. doi: 10.1016/S1002-0071(12)60086-0
Zhang, L., Deng, X., and Huang, Z. (1997). Miscibility, thermal behaviour and morphological structure of poly(3-hydroxybutyrate) and ethyl cellulose binary blends. Polymer (Guildf). 38, 5379–5387. doi: 10.1016/S0032-3861(97)84642-3
Zhang, Q., Liu, Q., Mark, J. E., and Noda, I. (2009). A novel biodegradable nanocomposite based on poly (3-hydroxybutyrate-co-3-hydroxyhexanoate) and silylated kaolinite/silica core-shell nanoparticles. Appl. Clay Sci. 46, 51–56. doi: 10.1016/j.clay.2009.07.008
Zhao, K., Yang, X., Chen, G. Q., and Chen, J. C. (2002). Effect of lipase treatment on the biocompatibility of microbial polyhydroxyalkanoates. J. Mater. Sci. Mater. Med. 13, 849–854. doi: 10.1023/A:1016596228316
Keywords: polyhydroxyalkanoate, data mining, trends, composites, medicine, renewable production, polyhydroxyalkanoate (PHA) synthesis, biotechnology
Citation: Guzik M, Witko T, Steinbüchel A, Wojnarowska M, Sołtysik M and Wawak S (2020) What Has Been Trending in the Research of Polyhydroxyalkanoates? A Systematic Review. Front. Bioeng. Biotechnol. 8:959. doi: 10.3389/fbioe.2020.00959
Received: 15 June 2020; Accepted: 24 July 2020;
Published: 10 September 2020.
Edited by:
Justyna Mozejko-Ciesielska, University of Warmia and Mazury in Olsztyn, PolandReviewed by:
Stanislav Obruca, Brno University of Technology, CzechiaChristopher John Brigham, Wentworth Institute of Technology, United States
Copyright © 2020 Guzik, Witko, Steinbüchel, Wojnarowska, Sołtysik and Wawak. This is an open-access article distributed under the terms of the Creative Commons Attribution License (CC BY). The use, distribution or reproduction in other forums is permitted, provided the original author(s) and the copyright owner(s) are credited and that the original publication in this journal is cited, in accordance with accepted academic practice. No use, distribution or reproduction is permitted which does not comply with these terms.
*Correspondence: Maciej Guzik, bmNndXppayYjeDAwMDQwO2N5ZnJvbmV0LnBs