- 1State Key Laboratory of Agricultural Microbiology, College of Science, Huazhong Agricultural University, Wuhan, China
- 2State Key Laboratory of Agricultural Microbiology, College of Animal Sciences and Technology, Huazhong Agricultural University, Wuhan, China
- 3The Cooperative Innovation Center for Sustainable Pig Production, Wuhan, China
- 4Hubei Provincial Engineering Laboratory for Pig Precision Feeding and Feed Safety Technology, Wuhan, China
The organ-on-a-chip (OOC) technology has been utilized in a lot of biomedical fields such as fundamental physiological and pharmacological researches. Various materials have been introduced in OOC and can be broadly classified into inorganic, organic, and hybrid materials. Although PDMS continues to be the preferred material for laboratory research, materials for OOC are constantly evolving and progressing, and have promoted the development of OOC. This mini review provides a summary of the various type of materials for OOC systems, focusing on the progress of materials and related fabrication technologies within the last 5 years. The advantages and drawbacks of these materials in particular applications are discussed. In addition, future perspectives and challenges are also discussed.
Introduction
An organ-on-a-chip (OOC) is a microfluidics-based cell culture device that contains continuously perfused chambers inhabited by living cells to simulate tissue- and organ-level physiology (Bhatia and Ingber, 2014; Ahadian et al., 2018). The development of OOC stems from the recognition that the conventional two-dimensional static cell culture methods lack the ability to mimic the environment that cells experience in vivo (Ryan et al., 2016; Duval et al., 2017). Microfluidic technology provides a way to simulate spatiotemporal chemical gradients, dynamic mechanical forces, and critical tissue interfaces by manipulation of fluids at micro levels. OOC systems that can recreate key aspects of the complex physiological microenvironment of human lung (Huh et al., 2010), heart (Maoz et al., 2017), stomach (Lee K. K. et al., 2018), intestine (Kim et al., 2016), liver (Weng et al., 2017), kidney (Sateesh et al., 2018), blood vessels (Wang et al., 2015), etc., have been developed. Moreover, multi-organs-on-a-chip or body-on-a-chip systems have been proposed (Sung et al., 2019; Zhao et al., 2019a). OOC platforms have shown application potential in a lot of biomedical fields such as fundamental physiological and pharmacological researches (Zhang and Radisic, 2017; Zhang et al., 2018a).
Materials play the major roles in the development of microfluidics and OOC technologies. In general, material considerations include non-toxic to cells, gas permeable, optically transparent for microscopic imaging, costs of the materials and the fabrication process, and the ability to model specific properties of organs (Lee et al., 2014). Although polydimethylsiloxane (PDMS) is still the most common material for laboratory research, emerging materials such as hydrogel, paper and hybrid materials are being developed and used. In this mini review, the classic and advanced materials and fabrication technologies for OOC devices are introduced and discussed, focusing on the progress within the last 5 years. The major properties, limitations, and typical applications in OOC of some representative materials are summarized in Table 1. Future perspectives and challenges in the development of materials for OOCs are briefly discussed.
Materials for OOCs
Inorganic Materials
Silicon and glass are the main inorganic materials for OOCs. The first-generation microscale cell culture analog (μCCA) devices mimicking the organ-level function of human physiology were fabricated on silicon (Sin et al., 2004; Mahler et al., 2009). Compared to opaque silicon, glass is optically transparent and optimal for real-time imaging, while reducing the absorbance of hydrophobic molecules and the adsorption of biomolecules (Lee S. et al., 2017; Kulthong et al., 2018). Nevertheless, glass chips with enclosed channels are not suitable for long-term cell culture because glass is not gas permeable. Another problem is that glass is typically processed with standard photolithography and etching, which are time-consuming and expensive. Recently, femtosecond laser ablation technique has been applied to fabricate 3D structures in glass-based OOCs (Xu et al., 2015; Schulze et al., 2017). Liquid glass, a photocurable amorphous silica nanocomposite enabling soft replication, has been developed for low-cost prototyping of glass microfluidics (Kotz et al., 2016).
Elastomer
Elastomers are polymers with elasticity, and generally having lower Young’s modulus and higher yield strain than other materials. PDMS is one of the most common materials used for the fabrication of microchips for the life science applications. It is not only gas permeable, biocompatible and optically transparent, but also particularly useful in prototyping new devices by soft lithography and micromolding technique (McDonald and Whitesides, 2002). Its elasticity allows to demold the PDMS replica with complex 3D structures (Suzuki et al., 2017). Moreover, the elasticity can be used to fabricate biomimetic cell culture scaffolds, such as the human lung-on-a-chip and gut-on-a-chip with pneumatically controlled deformation (Figure 1A) (Huh et al., 2010; Kim et al., 2012) and the microvascular models (Choi et al., 2014; Zhang W. et al., 2016). Apart from the conventional replication method, other strategies including hybrid stamp approach (Kung et al., 2015), razor-printing (Cosson et al., 2015), sacrificial template methods (Cheng et al., 2016) can also be used for PDMS. An optimized blend of PDMS-methacrylate macromers has been developed and demonstrated for 3D stereolithography (SL) with mechanical properties similar to conventional thermally cured PDMS. The 3D-printable PDMS resin would facilitate the fabrication of PDMS-based OOC platforms (Bhattacharjee et al., 2018).
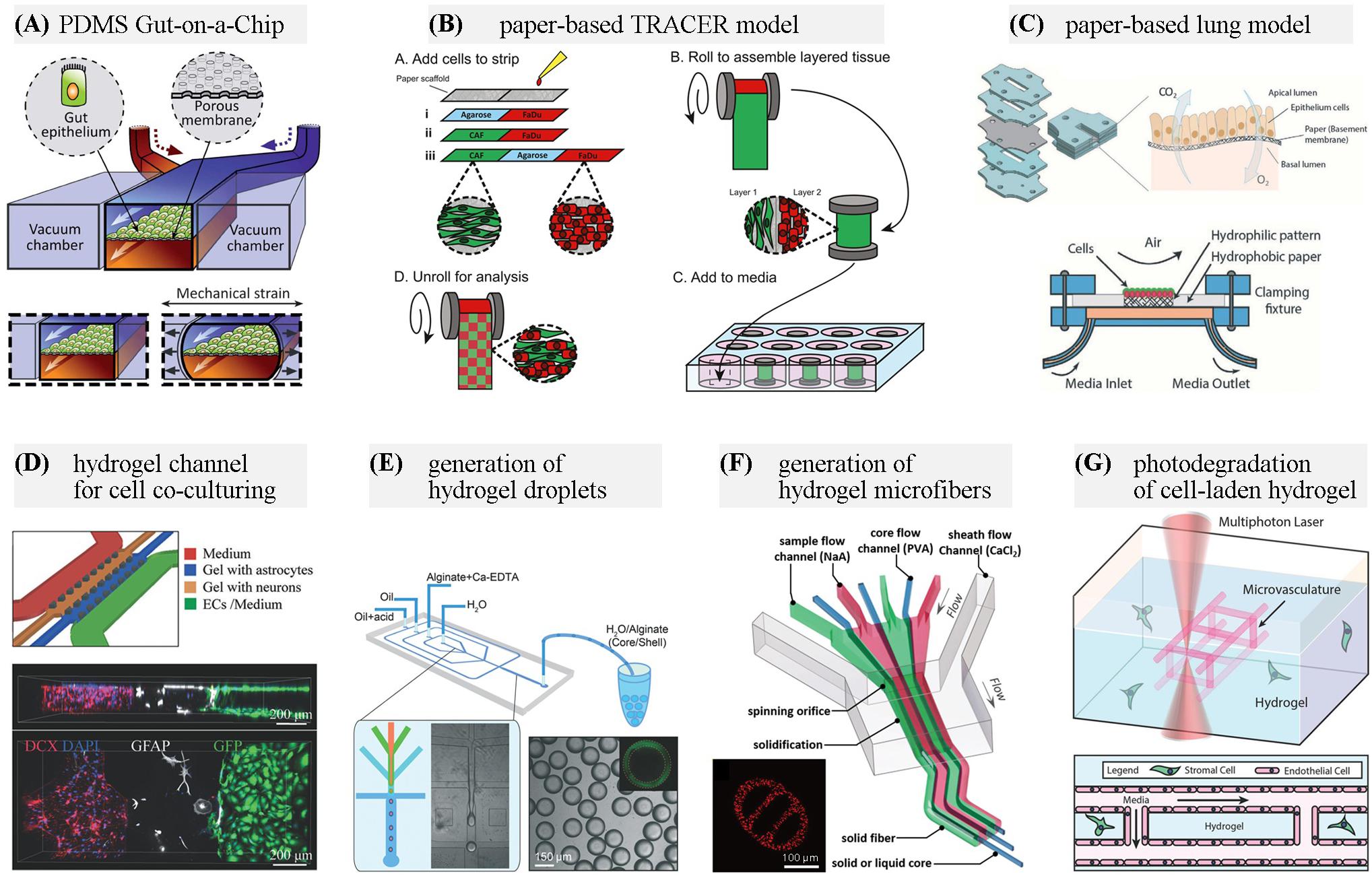
Figure 1. Representative materials used for OOC applications. (A) A PDMS gut-on-a-chip device containing two microchannels separated by a flexible porous membrane, and vacuum chambers on both sides. Adapted with permission from Kim et al. (2012), The Royal Society of Chemistry. (B) Configuration of a paper-based Tissue Roll for the Analysis of Cellular Environment and Response (TRACER) platform. Adapted with permission from Young et al. (2018), Elsevier. (C) Paper-based air-liquid-interface for in vitro human respiratory system model. Adapted with permission from Rahimi et al. (2016), The Royal Society of Chemistry. (D) A neurovascular chip with gel channels for cell co-culturing. Adapted with permission from Adriani et al. (2017), The Royal Society of Chemistry. (E) Generation of core-shell hydrogel droplets for spheroid-based liver model. Adapted with permission from Chen Q. et al. (2016), The Royal Society of Chemistry. (F) On-chip spinning of hydrogel microfibers with morphological and structural complexity, as well as a heterogeneous composition. Adapted with permission from Yu et al. (2016), John Wiley & Sons. (G) Microchannel generation within cell-laden hydrogel by user-programmed multiphoton excitation induced localized degradation. Adapted with permission from Arakawa et al. (2017), John Wiley & Sons.
Nevertheless, some characteristics of PDMS such as incompatibility with organic solvents, hydrophobicity and strong adsorption of biomolecules also limits its application in certain fields. Surface modifications of PDMS or the use of alternative materials may be feasible solutions. Some polymers with similar fabrication procedures suitable for rapid prototyping, higher rigidity, and better resistance to solvents, such as thermoset polyester (TPE), polyurethane methacrylate (PUMA) and Norland adhesive 81 (NOA81), have been assessed as complementary to PDMS (Sollier et al., 2011). However, they have not developed into the common choice in OOC devices. Styrene-(ethylene/butylene)-styrene (SEBS) copolymer (Domansky et al., 2017) and tetrafluoroethylene-propylene (FEPM) elastomer (Sano et al., 2019) that do not absorb hydrophobic molecules have been used for fabrication of OOCs for drug discovery and development.
To establish vascular networks, a biodegradable elastomer, poly(octamethylene maleate (anhydride) citrate) (POMaC) is used to construct a scaffold (AngioChip) with a build-in microchannel network. This material provides desired mechanical properties, biodegradation rate, and biocompatibility for specific applications (for example, human myocardium or liver tissue engineering) (Zhang B. et al., 2016; Zhang et al., 2018b). In a platform termed Biowire II, two parallel POMaC wires are suspended in the microwell between which cardiac tissue would self-assemble, matching the mechanical properties of the native cardiac tissue (Zhao et al., 2019b). A biodegradable elastomer with significantly low Young’s modulus has been synthesized and demonstrated utility in cardiac tissue engineering constructs (Davenport Huyer et al., 2016).
Plastic
Typical plastic materials for microfluidics include poly(methyl methacrylate) (PMMA), polycarbonate (PC), polystyrene (PS), Cyclic Olefin Polymer (COP) and Cyclic Olefin Copolymer (COC). They are generally optically transparent, more rigid than elastomers, less gas-permeable than PDMS, resistant to the permeation of small molecule, but incompatible with most organic solvents (Ren et al., 2013; Gencturk et al., 2017). Among these materials, PMMA has been widely utilized as substrate materials for OOC devices due to its rigid mechanical property, excellent optical transparency and low auto-fluorescence background (Chen X. et al., 2016; Miller and Shuler, 2016). Porous PC membranes are usually incorporated between microchannels in OOC systems to model tissue-tissue interfaces (Shah et al., 2016; Pocock et al., 2017). PS is highly biocompatible and suitable for cell growth and adhesion (Lee et al., 2019). COP and COC present excellent optical transmittance in both the visible and UV range, allowing for high quality fluorescence imaging. They are also FDA approved, showing a promising potential for future routine clinical use (Mottet et al., 2014). And recently, polylactic acid (PLA) as a sustainable, low absorption, low autofluorescence alternative to other plastics for OOC applications has been demonstrated (Ongaro et al., 2020).
Thermoplastics are suitable for thermo-processing, which is excellent for commercial production due to high production-rate and low cost, but not economical for prototypic use (Ren et al., 2013). Some novel materials such as a photocurable soft lithography compatible liquid PS prepolymer (Nargang et al., 2014) and a fast curing PMMA prepolymer that can be used as a negative photoresist and directly structured using UV or visible light (Kotz et al., 2018) have been developed for rapid prototyping. Fabrication methods for rapid prototyping of whole-thermoplastic microfluidic chips with microvalves and micropumps are being developed and could be employed for the OOC applications (Pourmand et al., 2018; Shaegh et al., 2018).
Paper
Paper microfluidics has the advantages of lightweight, easy-of-use and low cost. The cellulose matrix of paper allows for a porous structure for cell growth in a 3D format. Paper-based microfluidics with dynamic control of physiological microenvironment can be formed on multilayered paper and be used as high-throughput test platforms (Mosadegh et al., 2015; Sapp et al., 2015). And by directly incorporating a luminescent sensing film, the spatiotemporal oxygen consumption rates or pH gradients can be monitored in real-time through quantitative image analysis (Boyce et al., 2016; Kenney et al., 2018). In a device named tumor roll for analysis of cellular environment and response (TRACER), different cells are seeded in a defined area on the paper, and then the 3D tumors are assembled by rolling the biocomposite strip. By unrolling the strip, the model can be rapidly disassembled for snapshot analysis (Figure 1B) (Rodenhizer et al., 2016; Young et al., 2018). The Khademhosseini group presented the use of hydrophobic paper as a semi-permeable membrane for culturing cells at the air-liquid interface. The final paper-based device provides a cost-effective platform for human respiratory system studies under physiologically relevant conditions (Figure 1C) (Rahimi et al., 2016).
Having many similarities with paper, nitrocellulose membranes (Guo et al., 2018), threads (Yang et al., 2014), and cloths (Wu and Zhang, 2015) have also been investigated as a scaffold for cell culture. They have potential as superior alternatives to paper due to the stronger, higher controllable rates for fluid mixing and lower environmental impact (Bagherbaigi et al., 2014).
Hydrogel
Hydrogels are polymeric materials distinguished by high water content (Seliktar, 2012). They can mimic salient elements of native extracellular matrices (ECMs) due to their high biocompatibility and tunable properties, such as elasticity, porosity, permeability, stiffness and degradability. These properties of hydrogels are largely dependent on the types, gelation methods, and fabrication technologies. Hydrogels can be broadly classified into natural, synthetic, and hybrid according to their source (Caliari and Burdick, 2016; Jiang et al., 2016; Liu et al., 2019). Typical natural hydrogels include collagen, alginate, gelatin, agarose, and fibrin. They are generally highly biocompatible and containing cell-binding sites for cell attachment, spreading, growth, and differentiation. Collagen is the most common ECM component in the body and one of the most widely used hydrogels for bioengineered tissue microenvironments (Antoine et al., 2014). Gelatin has a similar composition to collagen. Gelatin methacryloyl (GelMA) hydrogels closely resemble some essential properties of native ECM and can be microfabricated using different methodologies (Yue et al., 2015). In recent studies, ECM hydrogels derived from decellularized tissues have been used to provide a supportive microenvironment capable of long-term culture of islets or directing cell growth (Giobbe et al., 2019; Jiang et al., 2019). Nevertheless, natural hydrogels suffer from some drawbacks such as relatively poor mechanical properties, limited long-term stability, and batch-to-batch variability. Typical synthetic hydrogels include polyethylene glycol (PEG) and its derivatives [e.g., PEG-diacrylate (PEG-DA)], polylactic acid (PLA), poly(lactic-co-glycolic acid) (PLGA), and poly(ε-caprolactone) (PCL). Synthetic and hybrid hydrogels are more tunable to provide desired mechanical properties and degradation rates.
Since the materials for chip fabrication mentioned above, such as PDMS and plastics, are unfavorable for cell attachment, hydrogels are often coated on the channel surfaces or integrated into OOC devices (Annabi et al., 2013; Zhang X. et al., 2016). By incorporating a collagen scaffold that mimics the human intestinal villi, the microfluidic gut-on-a-chip could provide cells with both 3D tissue structure and fluidic shear to induce further improvement in gut functions (Kim et al., 2014; Shim et al., 2017). A hydrogel microlayer consisting of type I collagen and Matrigel has been used in a lung airway-on-a-chip, replacing the semipermeable, PDMS membrane, allowing for the co-culture of epithelial cells and smooth muscle cells (Humayun et al., 2018). Hydrogels can also act as diffusion barriers which separate cells while permitting the soluble factors, such as nutrients, proteins, and signaling molecules exchange (Figure 1D) (Adriani et al., 2017). Compared with other artificial membranes used in OOC models, hydrogel barriers allow close cell association by making direct cell–cell contact between multiple cell types possible (Tibbe et al., 2018). Thanks to the progress in 3D (bio)printing technology, cell-laden hydrogels scaffolds can be rapidly created with spatial heterogeneity in predefined patterns (Miri et al., 2018; Moroni et al., 2018). Methods for the fabrication of hydrogel-based microfluidic chips are being developed. By stereolithographic high-resolution printing of PEG-DA, microfluidic chips with biofunctionalized complex 3D perfusion networks can be rapidly fabricated (Zhang and Larsen, 2017). Combining casting and bonding processes, Nie et al. (2018) fabricated a hydrogel-based vessel-on-a-chip of gelatin and GelMA.
Another frequently employed strategy for cell-based assays using hydrogels is to generate cell-encapsulated hydrogel droplets or hydrogel microfibers, especially through microfluidic approaches. On-chip production, storage, sorting and high-resolution imaging of hydrogel droplet has been achieved (Aubry et al., 2015). The generated microgels, including multicellular microspheres and microcapsules, create microenvironments for cell growth and proliferation (Figure 1E) (Headen et al., 2014; Alessandri et al., 2016; Chen Q. et al., 2016). By adjusting flow conditions in the microfluidic devices, various microfibers with morphological and compositional diversity can be generated as platforms for cell coculture (Figure 1F) (Yu et al., 2016; Xu et al., 2017; Liu et al., 2018; Xie et al., 2018).
The development of “smart” responsive hydrogels adapting to external stimuli has found its applications in OOC. Light-responsive hydrogels are of particular interest because of their capability of contact-free remote manipulation and the inherent space-time control capabilities of light stimulation (Li et al., 2019). Softening or stiffening hydrogels can be achieved by sequential photodegradation and photoinitiated crosslinking reactions, which is useful to design dynamic cell microenvironments (Rosales et al., 2017). Based on photodegradable hydrogels, 3D vascular networks within hydrogels can be altered dynamically, permitting user-defined 4D control even in the presence of live cells (Figure 1G) (Arakawa et al., 2017). In addition to applications related to cell culture, nanocomposite hydrogels crosslinking with metal or metal-oxide nanoparticles, and hydrogels of conducting polymers, such as poly(3,4-ethylenedioxythiophene): poly(styrene sulfonate) (PEDOT:PSS) based hydrogels, have biocompatibility, desired electrical and mechanical properties, and can be used to make sensors integrated in OOC platforms (Gaharwar et al., 2014; Park et al., 2015; Lu et al., 2019).
Organic-Inorganic Hybrid Materials
Organic–inorganic hybrid materials offer the advantages of the organic content and the inorganic matrix. By combining inorganic clay nanoparticles with polymer matrix, clay-polymer nanocomposites has the ability to marry important biomaterial parameters such as porosity or self-organization with mechanical strength and toughness. Enhancements in cell adhesion, proliferation, and differentiation in response to clay nanoparticles have been observed in investigation into clay-cell interactions, suggesting the potential for the generation of multifunctional scaffolds for tissue engineering (Dawson and Oreffo, 2013). A UV-curable hybrid ceramic polymer Ormocomp is inherently biocompatible supporting cell adhesion without any additional coating and has been utilized as scaffolds for cell culture (Scheiwe et al., 2015; Järvinen et al., 2020). Ormocomp has excellent transparency for VIS and near UV down to 350 nm. In a recent study, round concave cross-sectional shaped microchannels of Ormocomp were fabricated via single step lithography to improve the sensitivity of fluorescence imaging (Bonabi et al., 2017). Novel organic-inorganic hybrid materials can potentially be used in the fabrication of OOC devices (Mechref et al., 2016a, b).
Summary and Outlook
The OOC technology has been utilized in biomedical fields and has displayed great potential to speed up and simplify fundamental physiological and pathophysiological researches. The choice of chip materials is the first and crucial step for a successful OOC application. PDMS and plastics have been utilized as substrate materials for the majority of OOC platforms. Hydrogel materials are particularly suitable for mimicking native ECMs, and are often combined with other substrate materials to form hybrid chips. Many materials suitable for 3D (bio)printing technologies have been developed, providing a convenient method for prototyping complex chip structures. In particular, novel multi-material bioprinting technologies facilitate the fabrication of cell-laden constructs that highly similar to the biological tissues. These advances in materials and fabrication technologies have promoted the development of OOCs.
However, limitations and challenges exist. The hydrogel simulated microenvironments still differ from the native ECM microenvironments in stiffness, permeability and biochemical components. Moreover, the native microenvironment is diverse and may dynamically change during the stages of growth. It is important to design materials that can mimic the real ECM microenvironments as well as simple but precise methods to regulate the properties. In addition, the design of most OOC devices typically requires the assembly of hybrid materials. Novel materials together with fabrication methods covering both biological and engineering aspects can be a great challenge and an active area of research.
Author Contributions
CD and XY conceived and designed the manuscript. CD and XC wrote the original draft. QK and XY revised the manuscript. All the authors contributed to the article and approved the submitted version.
Funding
This research was financially supported by the National Natural Science Foundation of China (31730090), Hubei Provincial Natural Science Foundation of China (2018CFA020), and the Fundamental Research Funds for the Central Universities (2662018JC008 and 2662019YJ010).
Conflict of Interest
The authors declare that the research was conducted in the absence of any commercial or financial relationships that could be construed as a potential conflict of interest.
References
Adriani, G., Ma, D., Pavesi, A., Kamm, R. D., and Goh, E. L. K. (2017). A 3D neurovascular microfluidic model consisting of neurons, astrocytes and cerebral endothelial cells as a blood–brain barrier. Lab Chip 17, 448–459. doi: 10.1039/C6LC00638H
Ahadian, S., Civitarese, R., Bannerman, D., Mohammadi, M. H., Lu, R., Wang, E., et al. (2018). Organ-on-a-chip platforms: a convergence of advanced materials, cells, and microscale technologies. Adv. Healthc. Mater. 7:1700506. doi: 10.1002/adhm.201700506
Alessandri, K., Feyeux, M., Gurchenkov, B., Delgado, C., Trushko, A., Krause, K.-H., et al. (2016). A 3D printed microfluidic device for production of functionalized hydrogel microcapsules for culture and differentiation of human Neuronal Stem Cells (hNSC). Lab Chip 16, 1593–1604. doi: 10.1039/C6LC00133E
Annabi, N., Selimović, Š, Acevedo Cox, J. P., Ribas, J., Afshar Bakooshli, M., Heintze, D., et al. (2013). Hydrogel-coated microfluidic channels for cardiomyocyte culture. Lab Chip 13, 3569–3577. doi: 10.1039/C3LC50252J
Antoine, E. E., Vlachos, P. P., and Rylander, M. N. (2014). Review of collagen I hydrogels for bioengineered tissue microenvironments: characterization of mechanics, structure, and transport. Tissue Eng. Part B Rev. 20, 683–696.
Arakawa, C. K., Badeau, B. A., Zheng, Y., and DeForest, C. A. (2017). Multicellular vascularized engineered tissues through user-programmable biomaterial photodegradation. Adv. Mater. 29:1703156. doi: 10.1002/adma.201703156
Aubry, G., Zhan, M., and Lu, H. (2015). Hydrogel-droplet microfluidic platform for high-resolution imaging and sorting of early larval Caenorhabditis elegans. Lab Chip 15, 1424–1431. doi: 10.1039/C4LC01384K
Bagherbaigi, S., Córcoles, E. P., and Wicaksono, D. H. B. (2014). Cotton fabric as an immobilization matrix for low-cost and quick colorimetric enzyme-linked immunosorbent assay (ELISA). Anal. Methods 6, 7175–7180. doi: 10.1039/C4AY01071J
Bhatia, S. N., and Ingber, D. E. (2014). Microfluidic organs-on-chips. Nat. Biotechnol. 32, 760–772. doi: 10.1038/nbt.2989
Bhattacharjee, N., Parra-Cabrera, C., Kim, Y. T., Kuo, A. P., and Folch, A. (2018). Desktop-Stereolithography 3D-printing of a poly(dimethylsiloxane)-based material with sylgard-184 properties. Adv. Mater. 30:1800001. doi: 10.1002/adma.201800001
Bhise, N. S., Manoharan, V., Massa, S., Tamayol, A., Ghaderi, M., Miscuglio, M., et al. (2016). A liver-on-a-chip platform with bioprinted hepatic spheroids. Biofabrication 8:014101.
Bonabi, A., Cito, S., Tammela, P., Jokinen, V., and Sikanen, T. (2017). Fabrication of concave micromirrors for single cell imaging via controlled over-exposure of organically modified ceramics in single step lithography. Biomicrofluidics 11:034118. doi: 10.1063/1.4985653
Boyce, M. W., Kenney, R. M., Truong, A. S., and Lockett, M. R. (2016). Quantifying oxygen in paper-based cell cultures with luminescent thin film sensors. Anal. Bioanal. Chem. 408, 2985–2992. doi: 10.1007/s00216-015-9189-x
Caliari, S. R., and Burdick, J. A. (2016). A practical guide to hydrogels for cell culture. Nat. Methods 13, 405–414. doi: 10.1038/nmeth.3839
Chan, H. F., Zhang, Y., and Leong, K. W. (2016). Efficient one-step production of microencapsulated hepatocyte spheroids with enhanced functions. Small 12, 2720–2730. doi: 10.1002/smll.201502932
Chen, Q., Utech, S., Chen, D., Prodanovic, R., Lin, J.-M., and Weitz, D. A. (2016). Controlled assembly of heterotypic cells in a core–shell scaffold: organ in a droplet. Lab Chip 16, 1346–1349. doi: 10.1039/C6LC00231E
Chen, X., Shen, J., and Zhou, M. (2016). Rapid fabrication of a four-layer PMMA-based microfluidic chip using CO2-laser micromachining and thermal bonding. J. Micromech. Microeng. 26:107001. doi: 10.1088/0960-1317/26/10/107001
Cheng, S.-B., Xie, M., Xu, J.-Q., Wang, J., Lv, S.-W., Guo, S., et al. (2016). High-efficiency capture of individual and cluster of circulating tumor cells by a microchip embedded with three-dimensional Poly(dimethylsiloxane) Scaffold. Anal. Chem. 88, 6773–6780. doi: 10.1021/acs.analchem.6b01130
Choi, J. S., Piao, Y., and Seo, T. S. (2014). Circumferential alignment of vascular smooth muscle cells in a circular microfluidic channel. Biomaterials 35, 63–70. doi: 10.1016/j.biomaterials.2013.09.106
Cosson, S., Aeberli, L. G., Brandenberg, N., and Lutolf, M. P. (2015). Ultra-rapid prototyping of flexible, multi-layered microfluidic devices via razor writing. Lab Chip 15, 72–76. doi: 10.1039/C4LC00848K
Cruz-Acuña, R., Quirós, M., Farkas, A. E., Dedhia, P. H., Huang, S., Siuda, D., et al. (2017). Synthetic hydrogels for human intestinal organoid generation and colonic wound repair. Nat. Cell Biol. 19, 1326–1335. doi: 10.1038/ncb3632
Davenport Huyer, L., Zhang, B., Korolj, A., Montgomery, M., Drecun, S., Conant, G., et al. (2016). Highly elastic and moldable polyester biomaterial for cardiac tissue engineering applications. ACS Biomater. Sci. Eng. 2, 780–788. doi: 10.1021/acsbiomaterials.5b00525
Dawson, J. I., and Oreffo, R. O. C. (2013). Clay: new opportunities for tissue regeneration and biomaterial design. Adv. Mater. 25, 4069–4086. doi: 10.1002/adma.201301034
Domansky, K., Sliz, J. D., Wen, N., Hinojosa, C., Thompson, G., Fraser, J. P., et al. (2017). SEBS elastomers for fabrication of microfluidic devices with reduced drug absorption by injection molding and extrusion. Microfluid. Nanofluid. 21:107.
Duval, K., Grover, H., Han, L.-H., Mou, Y., Pegoraro, A. F., Fredberg, J., et al. (2017). Modeling physiological events in 2D vs. 3D cell culture. Physiology 32, 266–277. doi: 10.1152/physiol.00036.2016
Gaharwar, A. K., Peppas, N. A., and Khademhosseini, A. (2014). Nanocomposite hydrogels for biomedical applications. Biotechnol. Bioeng. 111, 441–453. doi: 10.1002/bit.25160
Gencturk, E., Mutlu, S., and Ulgen, K. O. (2017). Advances in microfluidic devices made from thermoplastics used in cell biology and analyses. Biomicrofluidics 11:051502. doi: 10.1063/1.4998604
Giobbe, G. G., Crowley, C., Luni, C., Campinoti, S., Khedr, M., Kretzschmar, K., et al. (2019). Extracellular matrix hydrogel derived from decellularized tissues enables endodermal organoid culture. Nat. Commun. 10:5658.
Guo, Y., Li, Z., Su, W., Wang, L., Zhu, Y., and Qin, J. (2018). A biomimetic human gut-on-a-chip for modeling drug metabolism in intestine. Artif. Organs 42, 1196–1205. doi: 10.1111/aor.13163
Headen, D. M., Aubry, G., Lu, H., and García, A. J. (2014). Microfluidic-based generation of size-controlled, biofunctionalized synthetic polymer microgels for cell encapsulation. Adv. Mater. 26, 3003–3008. doi: 10.1002/adma.201304880
Huh, D., Matthews, B. D., Mammoto, A., Montoya-Zavala, M., Hsin, H. Y., and Ingber, D. E. (2010). Reconstituting organ-level lung functions on a chip. Science 328, 1662–1668. doi: 10.1126/science.1188302
Humayun, M., Chow, C.-W., and Young, E. W. K. (2018). Microfluidic lung airway-on-a-chip with arrayable suspended gels for studying epithelial and smooth muscle cell interactions. Lab Chip 18, 1298–1309. doi: 10.1039/C7LC01357D
Järvinen, P., Bonabi, A., Jokinen, V., and Sikanen, T. (2020). Simultaneous culturing of cell monolayers and spheroids on a single microfluidic device for bridging the gap between 2d and 3d cell assays in drug research. Adv. Funct. Mater. 30:2000479. doi: 10.1002/adfm.202000479
Jeong, S.-Y., Lee, J.-H., Shin, Y., Chung, S., and Kuh, H.-J. (2016). Co-culture of tumor spheroids and fibroblasts in a collagen matrix-incorporated microfluidic chip mimics reciprocal activation in solid tumor microenvironment. PLoS One 11:e0159013. doi: 10.1371/journal.pone.0159013
Jiang, K., Chaimov, D., Patel, S. N., Liang, J. P., Wiggins, S. C., Samojlik, M. M., et al. (2019). 3-D physiomimetic extracellular matrix hydrogels provide a supportive microenvironment for rodent and human islet culture. Biomaterials 198, 37–48. doi: 10.1016/j.biomaterials.2018.08.057
Jiang, W., Li, M., Chen, Z., and Leong, K. W. (2016). Cell-laden microfluidic microgels for tissue regeneration. Lab Chip 16, 4482–4506. doi: 10.1039/c6lc01193d
Kang, J., Lee, D. W., Hwang, H. J., Yeon, S.-E., Lee, M.-Y., and Kuh, H.-J. (2016). Mini-pillar array for hydrogel-supported 3D culture and high-content histologic analysis of human tumor spheroids. Lab Chip 16, 2265–2276. doi: 10.1039/C6LC00526H
Kenney, R. M., Boyce, M. W., Whitman, N. A., Kromhout, B. P., and Lockett, M. R. (2018). A ph-sensing optode for mapping spatiotemporal gradients in 3D paper-based cell cultures. Anal. Chem. 90, 2376–2383. doi: 10.1021/acs.analchem.7b05015
Kim, H. J., Huh, D., Hamilton, G., and Ingber, D. E. (2012). Human gut-on-a-chip inhabited by microbial flora that experiences intestinal peristalsis-like motions and flow. Lab Chip 12, 2165–2174. doi: 10.1039/c2lc40074j
Kim, H. J., Li, H., Collins, J. J., and Ingber, D. E. (2016). Contributions of microbiome and mechanical deformation to intestinal bacterial overgrowth and inflammation in a human gut-on-a-chip. Proc. Natl. Acad. Sci. U.S.A. 113, E7–E15. doi: 10.1073/pnas.1522193112
Kim, S. H., Chi, M., Yi, B., Kim, S. H., Oh, S., Kim, Y., et al. (2014). Three-dimensional intestinal villi epithelium enhances protection of human intestinal cells from bacterial infection by inducing mucin expression. Integr. Biol. 6, 1122–1131. doi: 10.1039/c4ib00157e
Kotz, F., Arnold, K., Wagner, S., Bauer, W., Keller, N., Nargang, T. M., et al. (2018). Liquid PMMA: a high resolution polymethylmethacrylate negative photoresist as enabling material for direct printing of microfluidic chips. Adv. Eng. Mater. 20:1700699. doi: 10.1002/adem.201700699
Kotz, F., Plewa, K., Bauer, W., Schneider, N., Keller, N., Nargang, T., et al. (2016). Liquid glass: a facile soft replication method for structuring glass. Adv. Mater. 28, 4646–4650. doi: 10.1002/adma.201506089
Kulthong, K., Duivenvoorde, L., Mizera, B. Z., Rijkers, D., Dam, G. T., Oegema, G., et al. (2018). Implementation of a dynamic intestinal gut-on-a-chip barrier model for transport studies of lipophilic dioxin congeners. RSC Adv. 8, 32440–32453. doi: 10.1039/C8RA05430D
Kung, Y.-C., Huang, K.-W., Fan, Y.-J., and Chiou, P.-Y. (2015). Fabrication of 3D high aspect ratio PDMS microfluidic networks with a hybrid stamp. Lab Chip 15, 1861–1868. doi: 10.1039/C4LC01211A
Lee, H., Chung, M., and Jeon, N. L. (2014). Microvasculature: an essential component for organ-on-chip systems. MRS Bulletin 39, 51–59. doi: 10.1557/mrs.2013.286
Lee, K. K., McCauley, H. A., Broda, T. R., Kofron, M. J., Wells, J. M., and Hong, C. I. (2018). Human stomach-on-a-chip with luminal flow and peristaltic-like motility. Lab Chip 18, 3079–3085. doi: 10.1039/c8lc00910d
Lee, S., Jin, S.-P., Kim, Y. K., Sung, G. Y., Chung, J. H., and Sung, J. H. (2017). Construction of 3D multicellular microfluidic chip for an in vitro skin model. Biomed. Microdevices 19:22.
Lee, S., Lim, J., Yu, J., Ahn, J., Lee, Y., and Jeon, N. L. (2019). Engineering tumor vasculature on an injection-molded plastic array 3D culture (IMPACT) platform. Lab Chip 19, 2071–2080. doi: 10.1039/C9LC00148D
Lee, S. J., Wang, H.-J., Kim, T.-H., Choi, J. S., Kulkarni, G., Jackson, J. D., et al. (2018). In situ tissue regeneration of renal tissue induced by collagen hydrogel injection. Stem Cells Transl. Med. 7, 241–250. doi: 10.1002/sctm.16-0361
Li, L., Scheiger, J. M., and Levkin, P. A. (2019). Design and applications of photoresponsive hydrogels. Adv. Mater. 31:1807333. doi: 10.1002/adma.201807333
Li, R. A., Keung, W., Cashman, T. J., Backeris, P. C., Johnson, B. V., Bardot, E. S., et al. (2018). Bioengineering an electro-mechanically functional miniature ventricular heart chamber from human pluripotent stem cells. Biomaterials 163, 116–127. doi: 10.1016/j.biomaterials.2018.02.024
Li, X., George, S. M., Vernetti, L., Gough, A. H., and Taylor, D. L. (2018). A glass-based, continuously zonated and vascularized human liver acinus microphysiological system (vLAMPS) designed for experimental modeling of diseases and ADME/TOX. Lab Chip 18, 2614–2631. doi: 10.1039/C8LC00418H
Liu, H., Wang, Y., Cui, K., Guo, Y., Zhang, X., and Qin, J. (2019). Advances in hydrogels in organoids and organs-on-a-chip. Adv. Mater. 31:e1902042. doi: 10.1002/adma.201902042
Liu, Y., Xu, P., Liang, Z., Xie, R., Ding, M., Liu, H., et al. (2018). Hydrogel microfibers with perfusable folded channels for tissue constructs with folded morphology. RSC Adv. 8, 23475–23480. doi: 10.1039/C8RA04192J
Lu, B., Yuk, H., Lin, S., Jian, N., Qu, K., Xu, J., et al. (2019). Pure PEDOT:PSS hydrogels. Nat. Commun. 10:1043.
Ma, Z., Wang, J., Loskill, P., Huebsch, N., Koo, S., Svedlund, F. L., et al. (2015). Self-organizing human cardiac microchambers mediated by geometric confinement. Nat. Commun. 6, 7413. doi: 10.1038/ncomms8413
Mahler, G. J., Esch, M. B., Glahn, R. P., and Shuler, M. L. (2009). Characterization of a gastrointestinal tract microscale cell culture analog used to predict drug toxicity. Biotechnol. Bioeng. 104, 193–205. doi: 10.1002/bit.22366
Maoz, B. M., Herland, A., Henry, O. Y. F., Leineweber, W. D., Yadid, M., Doyle, J., et al. (2017). Organs-on-Chips with combined multi-electrode array and transepithelial electrical resistance measurement capabilities. Lab Chip 17, 2294–2302. doi: 10.1039/C7LC00412E
McDonald, J. C., and Whitesides, G. M. (2002). Poly(dimethylsiloxane) as a material for fabricating microfluidic devices. Acc. Chem. Res. 35, 491–499. doi: 10.1021/ar010110q
Mechref, E., Jabbour, J., Calas-Etienne, S., Amro, K., Mehdi, A., Tauk, R., et al. (2016a). New organic–inorganic hybrid material based on a poly(amic acid) oligomer: a promising opportunity to obtain microfluidic devices by a photolithographic process. RSC Adv. 6, 90666–90673. doi: 10.1039/C6RA10584J
Mechref, E., Jabbour, J., Calas-Etienne, S., Amro, K., Mehdi, A., Tauk, R., et al. (2016b). Synthesis and characterization of a photosensitive organic–inorganic, hybrid positive resin type material: application to the manufacture of microfluidic devices by laser writing. RSC Adv. 6, 3951–3959. doi: 10.1039/C5RA21393B
Miller, P. G., and Shuler, M. L. (2016). Design and demonstration of a pumpless 14 compartment microphysiological system. Biotechnol. Bioeng. 113, 2213–2227. doi: 10.1002/bit.25989
Miri, A. K., Nieto, D., Iglesias, L., Goodarzi Hosseinabadi, H., Maharjan, S., Ruiz-Esparza, G. U., et al. (2018). Microfluidics-enabled multimaterial maskless stereolithographic bioprinting. Adv. Mater. 30:e1800242. doi: 10.1002/adma.201800242
Moroni, L., Burdick, J. A., Highley, C., Lee, S. J., Morimoto, Y., Takeuchi, S., et al. (2018). Biofabrication strategies for 3D in vitro models and regenerative medicine. Nat. Rev. Mater. 3, 21–37. doi: 10.1038/s41578-018-0006-y
Mosadegh, B., Lockett, M. R., Minn, K. T., Simon, K. A., Gilbert, K., Hillier, S., et al. (2015). A paper-based invasion assay: assessing chemotaxis of cancer cells in gradients of oxygen. Biomaterials 52, 262–271. doi: 10.1016/j.biomaterials.2015.02.012
Mottet, G., Perez-Toralla, K., Tulukcuoglu, E., Bidard, F.-C., Pierga, J.-Y., Draskovic, I., et al. (2014). A three dimensional thermoplastic microfluidic chip for robust cell capture and high resolution imaging. Biomicrofluidics 8, 024109. doi: 10.1063/1.4871035
Nargang, T. M., Brockmann, L., Nikolov, P. M., Schild, D., Helmer, D., Keller, N., et al. (2014). Liquid polystyrene: a room-temperature photocurable soft lithography compatible pour-and-cure-type polystyrene. Lab Chip 14, 2698–2708. doi: 10.1039/c4lc00045e
Ng, S. S., Saeb-Parsy, K., Blackford, S. J. I., Segal, J. M., Serra, M. P., Horcas-Lopez, M., et al. (2018). Human iPS derived progenitors bioengineered into liver organoids using an inverted colloidal crystal poly (ethylene glycol) scaffold. Biomaterials 182, 299–311. doi: 10.1016/j.biomaterials.2018.07.043
Nie, J., Gao, Q., Wang, Y., Zeng, J., Zhao, H., Sun, Y., et al. (2018). Vessel-on-a-chip with hydrogel-based microfluidics. Small 14:1802368. doi: 10.1002/smll.201802368
Ning, L., Xu, Y., Chen, X., and Schreyer, D. J. (2016). Influence of mechanical properties of alginate-based substrates on the performance of Schwann cells in culture. J. Biomater. Sci. Polym. Ed. 27, 898–915. doi: 10.1080/09205063.2016.1170415
Ongaro, A. E., Di Giuseppe, D., Kermanizadeh, A., Miguelez Crespo, A., Mencattini, A., Ghibelli, L., et al. (2020). Polylactic is a sustainable, low absorption, low autofluorescence alternative to other plastics for microfluidic and organ-on-chip applications. Anal. Chem. 92, 6693–6701. doi: 10.1021/acs.analchem.0c00651
Park, S., Kang, Y. J., and Majd, S. (2015). A review of patterned organic bioelectronic materials and their biomedical applications. Adv. Mater. 27, 7583–7619. doi: 10.1002/adma.201501809
Pocock, K., Delon, L., Bala, V., Rao, S., Priest, C., Prestidge, C., et al. (2017). Intestine-on-a-chip microfluidic model for efficient in vitro screening of oral chemotherapeutic uptake. ACS Biomater. Sci. Eng. 3, 951–959. doi: 10.1021/acsbiomaterials.7b00023
Pourmand, A., Shaegh, S. A. M., Ghavifekr, H. B., Najafi Aghdam, E., Dokmeci, M. R., Khademhosseini, A., et al. (2018). Fabrication of whole-thermoplastic normally closed microvalve, micro check valve, and micropump. Sens. Actuators B Chem. 262, 625–636. doi: 10.1016/j.snb.2017.12.132
Rahimi, R., Htwe, S. S., Ochoa, M., Donaldson, A., Zieger, M., Sood, R., et al. (2016). A paper-based in vitro model for on-chip investigation of the human respiratory system. Lab Chip 16, 4319–4325. doi: 10.1039/C6LC00866F
Ren, K., Zhou, J., and Wu, H. (2013). Materials for microfluidic chip fabrication. Acc. Chem. Res. 46, 2396–2406. doi: 10.1021/ar300314s
Rodenhizer, D., Gaude, E., Cojocari, D., Mahadevan, R., Frezza, C., Wouters, B. G., et al. (2016). A three-dimensional engineered tumour for spatial snapshot analysis of cell metabolism and phenotype in hypoxic gradients. Nat. Mater. 15, 227–234. doi: 10.1038/nmat4482
Rosales, A. M., Vega, S. L., DelRio, F. W., Burdick, J. A., and Anseth, K. S. (2017). Hydrogels with reversible mechanics to probe dynamic cell microenvironments. Angew. Chem. Int. Ed. 56, 12132–12136. doi: 10.1002/anie.201705684
Ryan, S.-L., Baird, A.-M., Vaz, G., Urquhart, A. J., Senge, H., Richard, D. J., et al. (2016). Drug discovery approaches utilizing three-dimensional cell culture. ASSAY Drug Dev. Technol. 14, 19–28. doi: 10.1089/adt.2015.670
Sano, E., Mori, C., Matsuoka, N., Ozaki, Y., Yagi, K., Wada, A., et al. (2019). Tetrafluoroethylene-propylene elastomer for fabrication of microfluidic organs-on-chips resistant to drug absorption. Micromachines 10:793. doi: 10.3390/mi10110793
Sapp, M. C., Fares, H. J., Estrada, A. C., and Grande-Allen, K. J. (2015). Multilayer three-dimensional filter paper constructs for the culture and analysis of aortic valvular interstitial cells. Acta Biomater. 13, 199–206. doi: 10.1016/j.actbio.2014.11.039
Sateesh, J., Guha, K., Dutta, A., Sengupta, P., and Srinivasa Rao, K. (2018). Design and analysis of microfluidic kidney-on-chip model: fluid shear stress based study with temperature effect. Microsyst. Technol. 25, 2553–2560. doi: 10.1007/s00542-018-4261-z
Scheiwe, A. C., Frank, S. C., Autenrieth, T. J., Bastmeyer, M., and Wegener, M. (2015). Subcellular stretch-induced cytoskeletal response of single fibroblasts within 3D designer scaffolds. Biomaterials 44, 186–194. doi: 10.1016/j.biomaterials.2014.12.018
Schulze, T., Mattern, K., Früh, E., Hecht, L., Rustenbeck, I., and Dietzel, A. (2017). A 3D microfluidic perfusion system made from glass for multiparametric analysis of stimulus-secretioncoupling in pancreatic islets. Biomed. Microdevices 19:47. doi: 10.1007/s10544-017-0186-z
Seliktar, D. (2012). Designing cell-compatible hydrogels for biomedical applications. Science 336, 1124–1128. doi: 10.1126/science.1214804
Shaegh, S. A. M., Pourmand, A., Nabavinia, M., Avci, H., Tamayol, A., Mostafalu, P., et al. (2018). Rapid prototyping of whole-thermoplastic microfluidics with built-in microvalves using laser ablation and thermal fusion bonding. Sens. Actuators B Chem. 255, 100–109. doi: 10.1016/j.snb.2017.07.138
Shah, P., Fritz, J. V., Glaab, E., Desai, M. S., Greenhalgh, K., Frachet, A., et al. (2016). A microfluidics-based in vitro model of the gastrointestinal human-microbe interface. Nat. Commun. 7:11535. doi: 10.1038/ncomms11535
Shim, K. Y., Lee, D., Han, J., Nguyen, N. T., Park, S., and Sung, J. H. (2017). Microfluidic gut-on-a-chip with three-dimensional villi structure. Biomed. Microdevices 19:37. doi: 10.1007/s10544-017-0179-y
Sin, A., Chin, K. C., Jamil, M. F., Kostov, Y., Rao, G., and Shuler, M. L. (2004). The design and fabrication of three-chamber microscale cell culture analog devices with integrated dissolved oxygen sensors. Biotechnol. Prog. 20, 338–345. doi: 10.1021/bp034077d
Sollier, E., Murray, C., Maoddi, P., and Di Carlo, D. (2011). Rapid prototyping polymers for microfluidic devices and high pressure injections. Lab Chip 11, 3752–3765. doi: 10.1039/C1LC20514E
Sung, J. H., Wang, Y. I., Narasimhan Sriram, N., Jackson, M., Long, C., Hickman, J. J., et al. (2019). Recent advances in body-on-a-chip systems. Anal. Chem. 91, 330–351. doi: 10.1021/acs.analchem.8b05293
Suzuki, H., Mitsuno, K., Shiroguchi, K., Tsugane, M., Okano, T., Dohi, T., et al. (2017). One-step micromolding of complex 3D microchambers for single-cell analysis. Lab Chip 17, 647–652. doi: 10.1039/c6lc01313a
Tibbe, M. P., Leferink, A. M., van den Berg, A., Eijkel, J. C. T., and Segerink, L. I. (2018). Microfluidic gel patterning method by use of a temporary membrane for organ-on-chip applications. Adv. Mater. Technol. 3:1700200. doi: 10.1002/admt.201700200
Wang, X. Y., Pei, Y., Xie, M., Jin, Z. H., Xiao, Y. S., Wang, Y., et al. (2015). An artificial blood vessel implanted three-dimensional microsystem for modeling transvascular migration of tumor cells. Lab Chip 15, 1178–1187. doi: 10.1039/c4lc00973h
Weng, Y. S., Chang, S. F., Shih, M. C., Tseng, S. H., and Lai, C. H. (2017). Scaffold-free liver-on-a-chip with multiscale organotypic cultures. Adv. Mater. 29:1701545. doi: 10.1002/adma.201701545
Wu, P., and Zhang, C. (2015). Low-cost, high-throughput fabrication of cloth-based microfluidic devices using a photolithographical patterning technique. Lab Chip 15, 1598–1608. doi: 10.1039/c4lc01135j
Xie, R., Xu, P., Liu, Y., Li, L., Luo, G., Ding, M., et al. (2018). Necklace-like microfibers with variable knots and perfusable channels fabricated by an oil-free microfluidic spinning process. Adv. Mater. 30:1705082. doi: 10.1002/adma.201705082
Xu, J., Wu, D., Ip, J. Y., Midorikawa, K., and Sugioka, K. (2015). Vertical sidewall electrodes monolithically integrated into 3D glass microfluidic chips using water-assisted femtosecond-laser fabrication for in situ control of electrotaxis. RSC Adv. 5, 24072–24080. doi: 10.1039/c5ra00256g
Xu, P., Xie, R., Liu, Y., Luo, G., Ding, M., and Liang, Q. (2017). Bioinspired microfibers with embedded perfusable helical channels. Adv. Mater. 29:1701664. doi: 10.1002/adma.201701664
Yamada, M., Hori, A., Sugaya, S., Yajima, Y., Utoh, R., Yamato, M., et al. (2015). Cell-sized condensed collagen microparticles for preparing microengineered composite spheroids of primary hepatocytes. Lab Chip 15, 3941–3951. doi: 10.1039/C5LC00785B
Yang, L., Shridhar, S. V., Gerwitz, M., and Soman, P. (2016). An in vitro vascular chip using 3D printing-enabled hydrogel casting. Biofabrication 8, 035015. doi: 10.1088/1758-5090/8/3/035015
Yang, Y.-A., Lin, C.-H., and Wei, Y.-C. (2014). Thread-based microfluidic system for detection of rapid blood urea nitrogen in whole blood. Microfluid. Nanofluid. 16, 887–894.
Young, M., Rodenhizer, D., Dean, T., D’Arcangelo, E., Xu, B., Ailles, L., et al. (2018). A TRACER 3D Co-Culture tumour model for head and neck cancer. Biomaterials 164, 54–69. doi: 10.1016/j.biomaterials.2018.01.038
Yu, Y., Wei, W., Wang, Y., Xu, C., Guo, Y., and Qin, J. (2016). Simple spinning of heterogeneous hollow microfibers on chip. Adv. Mater. 28, 6649–6655. doi: 10.1002/adma.201601504
Yue, K., Trujillo-de Santiago, G., Alvarez, M. M., Tamayol, A., Annabi, N., and Khademhosseini, A. (2015). Synthesis, properties, and biomedical applications of gelatin methacryloyl (GelMA) hydrogels. Biomaterials 73, 254–271. doi: 10.1016/j.biomaterials.2015.08.045
Zhang, B., Korolj, A., Lai, B. F. L., and Radisic, M. (2018a). Advances in organ-on-a-chip engineering. Nat. Rev. Mater. 3, 257–278.
Zhang, B., Lai, B. F. L., Xie, R., Davenport Huyer, L., Montgomery, M., and Radisic, M. (2018b). Microfabrication of AngioChip, a biodegradable polymer scaffold with microfluidic vasculature. Nat. Protoc. 13, 1793–1813.
Zhang, B., Montgomery, M., Chamberlain, M. D., Ogawa, S., Korolj, A., Pahnke, A., et al. (2016). Biodegradable scaffold with built-in vasculature for organ-on-a-chip engineering and direct surgical anastomosis. Nat. Mater. 15, 669–678. doi: 10.1038/nmat4570
Zhang, B., and Radisic, M. (2017). Organ-on-a-chip devices advance to market. Lab Chip 17, 2395–2420. doi: 10.1039/c6lc01554a
Zhang, R., and Larsen, N. B. (2017). Stereolithographic hydrogel printing of 3D culture chips with biofunctionalized complex 3D perfusion networks. Lab Chip 17, 4273–4282. doi: 10.1039/c7lc00926g
Zhang, W., Zhang, Y. S., Bakht, S. M., Aleman, J., Shin, S. R., Yue, K., et al. (2016). Elastomeric free-form blood vessels for interconnecting organs on chip systems. Lab Chip 16, 1579–1586. doi: 10.1039/c6lc00001k
Zhang, X., Li, L., and Luo, C. (2016). Gel integration for microfluidic applications. Lab Chip 16, 1757–1776. doi: 10.1039/C6LC00247A
Zhang, Y. S., Arneri, A., Bersini, S., Shin, S.-R., Zhu, K., Goli-Malekabadi, Z., et al. (2016). Bioprinting 3D microfibrous scaffolds for engineering endothelialized myocardium and heart-on-a-chip. Biomaterials 110, 45–59. doi: 10.1016/j.biomaterials.2016.09.003
Zhao, X., Lang, Q., Yildirimer, L., Lin, Z. Y., Cui, W., Annabi, N., et al. (2016). Photocrosslinkable gelatin hydrogel for epidermal tissue engineering. Adv. Healthc. Mater. 5, 108–118. doi: 10.1002/adhm.201500005
Zhao, Y., Kankala, R. K., Wang, S. B., and Chen, A. Z. (2019a). Multi-organs-on-chips: towards long-term biomedical investigations. Molecules 24:675. doi: 10.3390/molecules24040675
Zhao, Y., Rafatian, N., Feric, N. T., Cox, B. J., Aschar-Sobbi, R., Wang, E. Y., et al. (2019b). A platform for generation of chamber-specific cardiac tissues and disease modeling. Cell 176, 913. doi: 10.1016/j.cell.2018.11.042
Zheng, Y., Chen, J., Craven, M., Choi, N. W., Totorica, S., Diaz-Santana, A., et al. (2012). In vitro microvessels for the study of angiogenesis and thrombosis. Proc. Natl. Acad. Sci. U.S.A. 109, 9342–9347. doi: 10.1073/pnas.1201240109
Keywords: organ-on-a-chip, microfluidics, elastomer, hydrogel, microfabrication
Citation: Ding C, Chen X, Kang Q and Yan X (2020) Biomedical Application of Functional Materials in Organ-on-a-Chip. Front. Bioeng. Biotechnol. 8:823. doi: 10.3389/fbioe.2020.00823
Received: 26 April 2020; Accepted: 29 June 2020;
Published: 22 July 2020.
Edited by:
Jianxun Ding, Changchun Institute of Applied Chemistry (CAS), ChinaReviewed by:
Peter Ertl, Vienna University of Technology, AustriaMario Rothbauer, Vienna University of Technology, Austria
Copyright © 2020 Ding, Chen, Kang and Yan. This is an open-access article distributed under the terms of the Creative Commons Attribution License (CC BY). The use, distribution or reproduction in other forums is permitted, provided the original author(s) and the copyright owner(s) are credited and that the original publication in this journal is cited, in accordance with accepted academic practice. No use, distribution or reproduction is permitted which does not comply with these terms.
*Correspondence: Xianghua Yan, eGh5YW5AbWFpbC5oemF1LmVkdS5jbg==