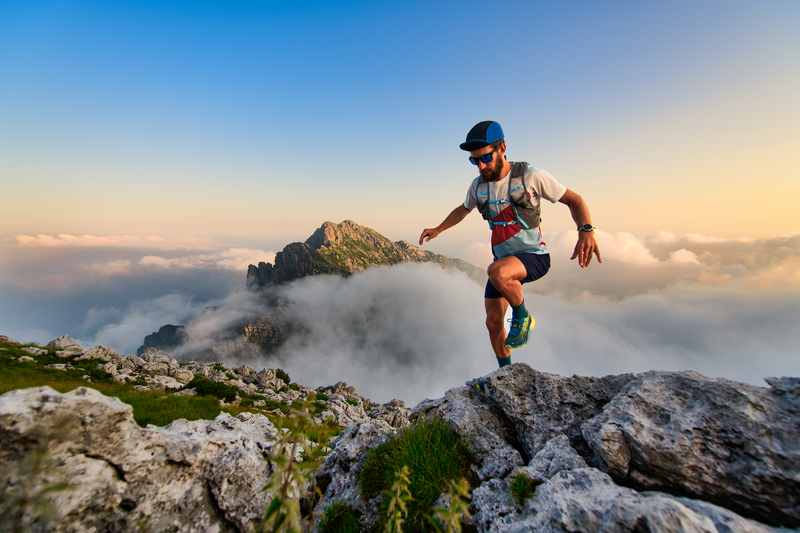
94% of researchers rate our articles as excellent or good
Learn more about the work of our research integrity team to safeguard the quality of each article we publish.
Find out more
ORIGINAL RESEARCH article
Front. Bioeng. Biotechnol. , 14 July 2020
Sec. Biomaterials
Volume 8 - 2020 | https://doi.org/10.3389/fbioe.2020.00783
This article is part of the Research Topic Synthesis, Functionalization, and Clinical Translation of Pharmaceutical Biomaterials View all 39 articles
Though radiofrequency ablation (RFA) is considered to be an effective treatment for hepatocellular carcinoma (HCC), but more than 30% of patients may suffer insufficient RFA (IRFA), which can promote more aggressive of the residual tumor. One possible method to counter this is to accurately identify the margin of the HCC. Colony-stimulating factor 1 receptor (CSF-1R) has been found to be restrictively expressed by tumor associated macrophages (TAMs) and monocytes which more prefer to locate at the boundary of HCC. Using biotinylation method, we developed a CSF-1R-conjugated nanobubble CSF-1R (NBCSF–1R) using a thin-film hydration method for margin detection of HCC. CSF-1R expression was higher in macrophages than in HCC cell lines. Furthermore, immunofluorescence showed that CSF-1R were largely located in the margin of xenograft tumor and IFRA models. In vitro, NBCSF–1R was stable and provided a clear ultrasound image even after being stored for 6 months. In co-culture, NBCSF–1R adhered to macrophages significantly better than HCC cells (p = 0.05). In in vivo contrast-enhanced ultrasound imaging, the washout half-time of the NBCSF–1R was significantly greater than that of NBCTRL and Sonovue® (p = 0.05). The signal intensity of the tumor periphery was higher than the tumor center or non-tumor region after NBCSF–1R injection. Taken together, NBCSF–1R may potentially be used as a non-invasive diagnostic modality in the margin detection of HCC, thereby improving the efficiency of RFA. This platform may also serve as a complement method to detect residual HCC after RFA; and may also be used for targeted delivery of therapeutic drugs or genes.
Hepatocellular carcinoma (HCC), is the third leading cause of cancer death in China (Chen et al., 2016). Radiofrequency ablation (RFA) which considered to be a valid local treatment method with curative intent and shows a comparable overall outcome to that of liver resection when patients with HCCs smaller than 3 cm in diameter (N’Kontchou et al., 2009; Kang et al., 2015). However, one major cause of insufficient RFA (IRFA) is the uncertain ablation margin, which may lead to local recurrence with a more aggressive phenotype and worse prognosis (Kim et al., 2010; Wang et al., 2013; Liu et al., 2015; Shady et al., 2016; Sotirchos et al., 2016; Dai et al., 2017; Zhang et al., 2019).
Some researchers found that colony-stimulating factor 1 receptor (CSF-1R) expression and tumor associated macrophage (TAM) density (CSF-1 receptor, CSF-1R or CD68) in the adjacent liver tissues are associated with patient survival after resection of HCC (Zhu et al., 2008; Jia et al., 2010; Kong et al., 2013). CSF-1R is highly expressed by monocytes (precursors of macrophages) and TAMs which support tumor cell proliferation, motility, and drug resistance (Lewis and Pollard, 2006; Pyonteck et al., 2013). CSF-1R and macrophages are the front line of defense to prevent tumor growth. The peritumoral liver tissue, which possessed of abundant CSF-1R, plays an opposite role in anti-tumor effect by providing a fertile environment for metastasis (Qian and Pollard, 2010). A high density of CSF-1R in peritumoral liver tissue, but not in tumor tissue, was associated with poor survival and a high incidence of metastasis after resection of the primary tumor (Zhu et al., 2008; Nandi et al., 2013). Leftin et al., 2019 confirmed that macrophage-targeted inhibition of CSF-1R by immunotherapy inhibits macrophage accumulation and slows mammary tumor growth in vivo. Thus, CSF-1R might be a feasible target for molecular imaging of HCC.
Ultrasound molecular imaging can provide high specificity and sensitivity imaging as it combines the advantages of ultrasound contrast agents (UCAs). UCAs can targeted with ligands such as antibodies or other proteins to detect expression of cancer-specific molecular markers (Jiang et al., 2016; Li et al., 2018; Wang et al., 2018). Unfortunately, traditional UCAs composed of microbubbles with a diameter about several micrometers, which cannot penetrate through the vasculature and have the short circulation time, which has constrained the advancement of ultrasound molecular imaging (Krupka et al., 2010; Wang et al., 2010). Nanobubbles (NBs, <1000 nm) were then introduced as a contrast agent enhancer in ultrasound imaging. However, NBs may decrease the echogenicity under clinical ultrasound (Sheeran et al., 2013). So it extremely challenging to fabricate not only small, highly echogenic particles but also can provide new, paradigm shifting applications of ultrasound agents in diagnosis and therapy (theranostics; Guvener et al., 2017; Tang et al., 2017; Liu et al., 2019).
Herein, to address the above shortcomings, we designed a novel CSF-1R targeted nanobubble (NBCSF–1R) and characterized its properties in vitro and in vivo. We also investigated the specificity and efficacy of the nanobubbles (NBCTRL and NBCSF–1R) against HCC xenograft tumors and IRFA models to evaluate the feasibility of using NBCSF–1R in the clinical diagnosis of HCC margin (Scheme 1).
SCHEME 1. After radiofrequency ablation (RFA), macrophages are highly condensed at the tumor margin, highly expressing CSF-1R. By using nanobubbles targeting CSF-1R (NBCSF1R), we developed an ultrasound-guided CSF-1R specific targeted real-time monitoring of tumor margin. NBCSF1R could penetrate through the vascular and adhered to the macrophages, which can provide ultrasound molecular imaging to reveal the accurate margin of HCC.
1,2-distearoyl-sn-glycero-3-phosphocholine (DSPC) and 1,2-dipalmitoyl-sn-glycero- 3-phosphoethanolamine (DPPE) were purchased from Avanti Polar Lipids, Inc. (Alabaster, AL, United States). Polyethylene glycol (PEG4000) was purchased from Aladdin Limited Company (Shanghai, China). 1,2-distearoyl-sn-glycero-3-phosphoethanolamine-N-[biotinyl(polyethylene glycol)-2000] (DSPE-PEG2000-Biotin) was purchased from A.V.T. Pharmaceutical Co., Ltd. (Shanghai, China). 4′,6-diamidino-2-phenylindole (DAPI) and 1,1′-dioctadecyl-3,3,3′,3′-tetramethylindocarbocyanineperchlorate (DiI) were obtained from Beyotime (Haimen, China). Octafluoropropane (C3F8) was purchased from Guangzhou Walter (Guangzhou, China). Antibodies for flow cytometric analysis or Western blot of CSF-1R were purchased from Novus International, Inc. (St Charles, MO, United States). Antibodies for immunohistochemistry (IHC) or immunofluorescence (IF) for CSF-1R were obtained from Abcam (Cambridge, MA, United States). Other chemicals and reagents were of analytical grade. Phorbol 12-myristate 13-acetate (PMA) was purchased from Sigma-Aldrich (St. Louis, MO, United States).
Human monocyte THP-1 was purchased from Sun Yat-sen University Cell Bank. SMMC-7721 and HepG2 human liver cancer cell lines were kindly donated by the Radiology Department, Sun Yat-sen Memorial Hospital. The H22 cell line was obtained from Procell Life Science & Technology Co., Ltd. (Wuhan, China). Hepa1-6 mouse liver cancer cell lines were purchased from Guangzhou Genebio Biotechnology Co., Ltd. (Guangzhou, China). SMMC-7721, HepG2, and Hepa1-6 cells were cultured in Dulbecco’s modified Eagle’s medium (DMEM, GIBCO Gaithersburg, MD, United States) and supplemented with high glucose and 10% fetal bovine serum (FBS, GIBCO) at 37°C with 5% CO2. THP-1 and H22 were cultured separately in RPMI 1640 (GIBCO) and supplemented with 10% FBS at 37°C with 5% CO2. Macrophages were obtained from induction of THP-1 cells by 100 ng/ml of PMA for 24 h.
All animal procedures were performed in accordance with the Guidelines for Care and Use of Laboratory Animals of Sun Yat-sen University. Experiments were reviewed and approved (NO. SYSU-IACUC-2018-000179) by the Ethics Committee of Sun Yat-sen Memorial Hospital and Ethics Committee of Zhongshan School of Medicine (ZSSOM) on Laboratory Animal Care, Sun Yat-sen University (Guangdong, China).
Primary hepatocellular carcinomas were obtained from 30 patients at Sun Yat-sen Memorial Hospital. All samples were collected with informed consent and with the approval of the Internal Review and Ethics Boards of the indicated hospitals.
Quantitative real-time polymerase chain reaction (qRT-PCR), fluorescence-activated cell sorting (FACS), and Western blot were used to analyze the CSF-1R presentation in different cells. The following primers were used: human CSF-1R: forward (5′- > 3′) AGCGATAGGTCCCCGTGTTTT, reverse (5′- > 3′) CAACGGTGACCTTGCGATGTG, murine CSF-1R: forward (5′- > 3′) CAGGGTCCAAGGTCCAGTAGG, reverse (5′- > 3′) TGGTTGTAGAGCCGGGTGAAA. Macrophages and SMMC-7721 cells were seeded into six-well plates at 5 × 105 cells/well in 2 mL of medium for 12 h. Cells were collected and each sample was divided into two tubes. One tube was incubated with anti-CSF-1R antibodies for 30 min and then rinsed with phosphate-buffered saline (PBS) one time. Then, the samples were incubated with PE-conjugated anti-mouse IgG for 20 min and rinsed with PBS. The fluorescence intensity in the macrophages and SMMC-7721 cells was calculated by Flow Cytometry (Beckman Coulter, Fullerton, CA, United States).
Immunohistochemistry (IHC) analysis of human liver cancer tissue and peritumor tissue adjacent to tumor (about 10 mm) was performed. Procedures for IHC analysis of CSF-1R (anti-CSF-1R antibody, 1:200 dilution, Novus International, Inc., United States) were performed. Procedures for IHC analysis of CSF-1R (anti-CSF-1R antibody, ab215441, 1:100 dilution, Abcam, Cambridge, MA, United States) were performed in two random fields in tumor tissue and peritumor tissue for each slide. The quantification of stained cells was analyzed by Image-Pro Plus. The slides were observed by using a light microscope (ECLIPSE 80i, Nikon, Japan).
Nanobubbles were prepared according to our previous studies (Jiang et al., 2016; Zhou et al., 2019). Briefly, a homogenous mixture containing DSPE-PEG2000-biotin, DSPE-PEG2000, DSPC, and DPPE at a mole ratio of 2.5:2.5:30:10 was mixed in 15 mL chloroform. The mixture was stirred for 1 h, then vacuum dried for 2 h at 60°C using a rotary evaporator (EYELA, Tokyo, Japan). The resulting film was rehydrated with PBS and agitated for 2 h. The size of the resulting liposomes was reduced by sonication, and then C3F8 gas was injected to replace the air over the fluid to generate NBs.
The bubbles were purified by centrifugation and collected according to our previous research. Then, NBs were resuspended in PBS and stored at 4°C. For the development of fluorescent NBs, DiI-encapsulated NBs were prepared through the same method, with the addition of DiI in the initial mixture of phospholipids in chloroform. DiI-encapsulated NBCSF–1R were observed by inverted fluorescence microscope (Olympus IX73, Japan) and Western Blot. Excitation wavelength of Dil is 549 nm and the emission wavelength is 565 nm.
In order to determine the success of CSF-1R onto NBs surface, SDS-PAGE and Western blot were used. An 8% SDS–polyacrylamide gel was loaded with NBCTRL, NBCSF–1R, and CSF-1RmAb (Novus International, Inc., United States) and electrophoresed under reducing condition for 2 h at 60 mV and for an additional 180 min at 300 mA. The gel was then transferred to a membrane and blocked using 5% skim milk. After blocking, the membranes were Horseradish peroxidase (HRP)-conjugated goat anti-rabbit IgG (1:2000 dilution; Santa Cruz Biotechnology, Santa Cruz, CA, United States) was used as the secondary antibody. Protein signals were detected using a chemiluminescence system (New Life Science Products, Boston, MA, United States).
In vitro CSF-1RmAbs was biotinylated using the EZLink NHS-Biotin Kit (Muralidhara et al., 2019; Wang et al., 2019). Biotinylated CSF-1RmAb was bound to the NBs (NBCTRL) by linking the biotin groups of CSF-1RmAb and DSPE-PEG2000-biotin on the NBs with Streptavidin. Briefly, nanobubbles was mixed with biotinylated CSF-1RmAb using a DSPE-PEG2000-biotin:CSF-1RmAb: Streptavidin molar ratio of 30:1:15, then incubated at 4°C for 8 h (NBCSF–1R). To remove the excess free CSF-1RmAb, the upper layer of the suspension was centrifuged three times (1000 rpm, 5 min) and stored at 4°C. To determine the success of conjugation, the CSF-1RmAb was labeled with fluorescein isothiocyanate (FITC) and co-localization of the CSF-1RmAb with CSF-1R were confirmed by fluorescence microscope.
The mean diameter and Zeta potential of NBCTRL, and NBCSF–1R were measured using a Malvern Zetasizer Nano (Malvern Instruments, Ltd., United Kingdom). Their morphology was detected by scanning electron microscopy (SEM, SU8020, Hitachi, Japan). The concentration of NBs was measured with a Coulter counter (Multisizer 4e, United States) according to Liu et al. (2019).
The long-term stability test of NBCSF–1R were confirmed by using a Vevo 2100 small animal imaging device with a frequency of 20 MHz, in a static state. NBCSF–1R was diluted from 100 to 10,000 times. The contrast imaging was then observed for each sample. To determine the long-term stability of NBCSF–1R, the above experiments were repeated in samples that had been stored for 1, 3, or 6 months at 4°C. As a control, Sonovue® was suspended at the same concentration.
Macrophages were induced from THP-1 cells. Approximately 5 × 106 cells were cultured with 100 ng/ml PMA for 24 h at 37°C with 5% CO2. SMMC-7721 cells and macrophages were separately inoculated into 96-well plates at 3000 cells/well for 12 h. The same volume of fresh media with various concentrations NBCSF–1R were incubated with the cells for an additional 24 h, the concentration of NBCSF–1R ranging from 2 × 103 to 2 × 108 bubbles/ml. Then, 10 μL CCK-8 reagent in 100 μL fresh medium replaced, and incubated for an additional 2 h. The plates were gently shook for 5 min, and Infinite F200 multimode plate reader (Spectra Max M5, Molecular Devices) was used to test the absorbance of each well at 450 nm. All experiments were conducted in triplicate.
SMMC-7721 and macrophages were seeded into confocal dishes at 1 × 105 cells/dish and grown for 24 h at 37°C with 5% CO2. The cells were then rinsed gently with PBS three times at room temperature, 4% paraformaldehyde was added for 5 min, then cells were gently rinsed again with PBS three times. Then, 1 ml of PBS containing 0.5% Triton X-100 was added for 5 min and rinsed with PBS three times. The remaining steps were performed in the dark: added 100 μL of diluted phalloidin solution (5 μL of phalloidin solution to 200 μL of PBS containing 0.1% BSA) to cover the cells in the center of the confocal dish; incubated for 30 min; added 200 μL DiI labeled NBCSF–1R or NBCTRL to the center of the confocal dish and incubated for 2 h at 37°C with 5% CO2; added 200 μl of 100 ng/ml DAPI solution and incubated for 5 min; gently rinsed 5 times with PBS to remove the unbound CSF-1R. The cells were observed under a laser confocal microscope to observe the fluorescence distribution of the cytoskeleton and the NBCSF–1R, and the specific targeting of the NBCSF–1R to the CSF-1 was observed.
To generate tumors, approximately 1 × 107 SMMC-7721 cells in 100 μL of single-cell suspension was injected into 5–6-week-old male BALB/c nude mice (n = 30, five animals/group) in the right hind legs, subcutaneously (s.c.). The mean maximum tumor size at ultrasound was about 10 mm. In this experiment, the mice were divided into six groups (n = 30). Group 1 = NBCTRL, Group 2 = NBCSF–1R, Group 3 = Sonovue, Group 4 = NBCTRL + IRFA, Group 5 = NBCSF–1R + IRFA, Group 6 = Sonovue® + IRFA. During imaging, Mice were kept warm using a heated stage and a heat lamp, and anesthesia at 2% isoflurane in oxygen at 2 L/min during imaging. Mechanically, the contrast enhanced imaging can only generated while enveloped bubbles undergo compression and expansion. In this experiment, negative blank (PBS) was not included as PBS was unable to generate ultrasound intensity. Three groups received radiofrequency ablation to simulate IRFA models, which was performed using a bipolar RFA device (Radionics, INC, Burlington, MA, United States), radiofrequency energy about 30 watts for 30 s. One group of the xenograft tumor models and one group of the residual cancer models received NBCSF–1R. Mice were anesthetized with isoflurane by full anesthesia machine and placed on a warm pad. Approximately, 4 × 107 NBCSF–1R was injected through caudal veins. The ultrasound contrast parameters were: (Visual Sonics, Vevo 2100) Transducer: MS-250; Frequency: 20 MHz; Imaging Mode: Non-linear Contrast Mode; Dynamic Range: 30 dB; Overall Contrast Gain: 45 dB; Output Power: 4%. NBCTRL and Sonovue® were injected through caudal veins similarly. VevoCQ software was used to export the image of ultrasound molecular imaging (USMI) signal, and then observe the differential targeted enhancement distribution in the region of interest (green contour).
For data analysis, Statistical Package for the Social Sciences (SPSS) version-21 (SPSS, Inc., Chicago, IL, United States) was used. GraphPad Prism version 5.00 (GraphPad Software, Inc., San Diego, CA, United States) was used to generate figures. p < 0.05 was considered statistically significant. Data from the experiments was expressed as mean ± SD for technical replicates and the mean ± SEM for biological replicates. ANOVA was performed to compare differences between multiple groups and Differences in continuous variables were analyzed by Student’s t-test to compare two groups. A non-parametric test of two paired samples was analyzed by Wilcoxon Signed Rank Test.
To verify the expression of CSF-1R in vitro, qRT-PCR, Flow Cytometry, and Western blot were carried out. As seen in Figure 1A, qRT-PCR analysis revealed that the expression of CSF-1R mRNA is significantly higher in macrophages as compared to H22, SMMC-7721, HepG2, Hepa1-6, and THP-1 (p = 0.05). We then proceed to select a mouse originated cell line SMMC-7721 for consideration of in vivo experiments. Western blot analysis also confirmed that the protein level of CSF-1R is overexpressed in macrophages and THP-1, while minimally expressed in SMMC-7721 cells (Figure 1B). Comparison of CSF-1R intensity showed a significantly greater extent of expression within macrophages (macrophage: intensity of 143.75 ± 4.2 a.u.; THP-1: 103.02 ± 3.4 a.u.; SMMC-7721: 78.36 ± 3.4 a.u.; p < 0.001; Figure 1C). Quantification analysis using FACS indicated that 97.57% of macrophages are CSF-1R positive compared to 9.32% of SMMC-7721 cells (Figure 1D).
Figure 1. (A) qRT-PCR was used to determine the expression of colony-stimulating factor 1 receptor (CSF-1R) in different cell lines. (B) Western blot was used to access the protein level of CSF-1R in macrophages, THP-1, and SMMC-7721. (C) Showed the CSF-1R expression intensity in macrophages, THP-1, and SMMC-7721, macrophages showed the highest intensity than THP-1 and SMMC-7721, (***indicates p < 0.01). (D) The expression level of CSF-1R in macrophages and SMMC-7721 were detected by fluorescence-activated cell sorting (FACS) blue indicated the control group and, red indiacted the experiment group. (E) IHC verified the expression distribution of CSF-1R in human liver cancer tissues (the red line indicated the boundary of hepatocellular carcinoma (HCC). (P, peritumoral, T, tumor). (F) Semi-quantitatively analyzed the expression of CSF-1R by image-pro plus (IPP) software (***indicates p < 0.01).
Immunohistochemistry analysis was carried out to confirm the expression of CSF-1R in HCC patients. As seen in Figure 1E, CSF-1R deposits were detected in the peritumoral tissues of carcinoma in situ in human HCC (Figure 1E). The counts of positive CSF-1R differed significantly between the normal tissue and the margin (p < 0.05; Figure 1F).
Figure 2A shows the schematic illustration of NBCSF–1R fabrication through Streptavidin/biotin interaction. Western blot showed that the band intensity of CSF-1R attached on NBCSF–1R was similar with CSF-1R input, while NBCTRL showed no indication of CSF-1R band (Figure 2B), indicating that CSF-1R successfully conjugated with the NBCSF–1R specifically (p < 0.005; Figure 2C).
Figure 2. (A) The process of synthesis of nanobubble CSF-1R (NBCSF–1R) through the streptavidin/biotin chemical effect, CSF-1RmAb was biotinylated and then conjunction to the 1,2-distearoyl-sn-glycero-3-phosphoethanolamine-N-[biotinyl(polyethylene glycol)-2000] (DSPE-PEG2000)-Biotin. (B) Western blot showed that the NBCSF–1R band was at approximately the same position as CSF-1RmAb, and the NBCTRL showed no expression of CSF-1R. (C) Showed the Western blot intensity of NBCSF–1R, CSF-1R mAb, NBCTRL (**indicates p < 0.05, ***indicates p < 0.001).
Figure 3A depicted the two NBs synthesized, the non-targeted NBCTRL and the targeted NBCSF–1R. The morphologies of NBCTRL and NBCSF–1R were observed by SEM. As shown in Figures 3B,C, NBCTRL and NBCSF–1R were spherical, uniformed in size and had distinct shell structures. The physical properties of NBCTRL and NBCSF–1R are summarized in Figure 3D. Dynamic laser scattering (DLS) analysis indicated that the average hydrodynamic size of NBCTRL and NBCSF–1R was (408.0 ± 17.5) nm and (428.0 ± 12.47) nm, respectively. Zeta potential values showed that NBCTRL was with charge of −4.03 ± 0.23 mV, and NBCSF–1R was −4.42 ± 0.51 mV. The concentrations of NBCTRL and NBCSF–1R were (5.99 ± 0.08) × 108 bubbles/mL (n = 5) and (4.24 ± 0.07) × 108 bubbles/mL (n = 5), respectively.
Figure 3. (A) Showed the structure of the NBCTRL and NBCSF–1R, (B) scanning electron microscopy (SEM) image of NBCTRL with a scale bar of 500 nm (C) SEM image of NBCSF–1R with a scale bar of 500 nm. (D) Summary of the average size, and zeta potential of NBCTRL and NBCSF–1R as measured by dynamic laser scattering. Concentration of NBs were measured by Coulter counter. Data represent mean ± SD (n = 5).
To illustrate the in vitro binding efficacy and co-localization of NBCSF–1R with CSF-1R, we synthesized DiI-labeled NBCTRL while CSF-1RmAb were labeled with FITC. After co-incubation, the cells were observed under microscope. The green light of the FITC-labeled antibody (Figure 4A) and the red light of the DiI-labeled nanobubbles (Figure 4B) merged perfectly (Figure 4C), indicating that CSF-1RmAb were successfully attached to the NBs, and could specifically target CSF-1R.
Figure 4. Fluorescence microscopy image of target NBs. (A) CSF-1RmAb (FITC showed green fluorescence), (B) DiI-dyed (nanobubbles showed red fluorescence), and (C) their co-localization (merge) under fluorescence microscope, with a sale bar of 200 μm.
After the induction of THP-1 cells into macrophages by 100 ng/ml PMA, the cells changed from suspension state to adherent state, and some of the cells became spindle-like, which confirmed that successful induction of THP-1 cells into macrophages. The cytotoxicity of NBCTRL and NBCSF–1R was evaluated using SMMC-7721 and macrophages incubated with NBCTRL at five concentrations between 108 and 103/mL for 24 h (Figure 5A). Both SMMC-7721 and macrophages incubated with NBCTRL did not show significant changes in cell viability in all concentration after 24 h of incubation. The cell viability of both SMMC-7721 cells and macrophages remained (85% after incubation with either type of NBCTRL, indicating they were minimally cytotoxic. These results show that NBCTRL and NBCSF–1R have good biocompatibility and cause minimal harm to the tested cells.
Figure 5. (A) Cell viability test for NBCSF–1R determined through CCK-8. In vitro cytotoxicity assays using macrophage and (high CSF-1 expression) and SMMC-7721 cells (low CSF-1 expression) incubated with NBCSF–1R for 24 h; there was no significant difference in the viability of macrophages and SMMC-7721. (B) In vitro ultrasound images of NBCSF–1R stored for 0, 30, 90, 120, 180 days, and the Sonovue® stored for 0 days as a control. Ultrasound frequency, 20 MHz.
The echogenic properties of NBCSF–1R were investigated in agarose gel phantom in comparison to Sonovue® in vitro, using a Vevo 2100 small animal imaging device with a frequency of 20 MHz. The signal enhancements of NBCSF–1R stored at 4°C for different periods of time (0, 30, 90, 120, and 180 days) were investigated. As indicated in Figure 5B, echogram result of NBs at Day-180 indicated no significant difference between NBs and Sonovue® at Day-0 indicating that the NBCSF–1R was stable.
The capability of NBCSF–1R was also assessed in vitro using a Vevo 2100 small animal imaging device with a frequency of 20 MHz at various concentrations. Different concentrations of NBCSF–1R nanoparticles (approximately 1 × 104 ∼6.0 × 106/bubbles of same volume, 100 μL) were evaluated in this experiment. The signal intensity decreased with the decreasing concentrations of NBCSF–1R (Figure 6A). However, even when the NBCSF–1R were diluted 2000 times, the signal intensity remained relatively strong.
Figure 6. (A) In vitro ultrasound images of various concentrations of NBCSF–1R dilute for different times, imaging was still viable even when diluted 2000 times. Ultrasound frequency, 20 MHz. (B) In vitro, the same quantity of NBCSF–1R and NBCTRL were added to SMMC-7721 and macrophages and then observed using confocal laser scanning microscopy (CLSM). There were few NBCTRL adhered to SMMC-7721 and macrophages, and also few NBCTRL bounded to macrophages. There were a lot NBCSF–1R bounded to macrophages, and showed the specificity of targeting. With a scale bar of 100 μm.
To determine the binding ability of NBCTRL and NBCSF–1R in SMMC-7721 cells and macrophages, we carried out confocal laser scanning microscopy (CLSM) assay. The cytoskeletons with FITC phalloidin were green and the NBs labeled with DiI were red. As seen in Figure 6B, the red fluorescence intensity of macrophages treated with NBCSF–1R was much higher than SMMC-7721 cells treated with NBCSF–1R, NBCTRL and macrophages treated with NBCTRL, while minimal attachment of NBCSF–1R and NBCTRL were seen in SMMC-7721. This result indicates that more NBCSF–1R adhered to macrophages, and demonstrated its excellent targeting ability.
In vivo, NBCSF–1R, NBCTRL, and Sonovue® were tested in xenograft tumors and IRFA models which had been inoculated with SMMC-7721 cells (n = 30, 5 mice for each group). After examination, none of the mice exhibited apparent signs of distress in each group, under the same ultrasound conditions. Contrast-enhanced images of the tumors continuously exposed to ultrasound were taken at minutes 0, 5, 15, and 30 (Figures 7A,B). The peak intensity and washout half-time were compared between NBCSF–1R, NBCTRL, and Sonovue® in these models (Figures 7C,D). The peak intensity of NBCS–F1R, NBCTRL, and Sonovue® (Figure 7C) was 11.55 ± 1.397 a.u, 8.826 ± 1.348 a.u, 12.20 ± 1.974 a.u in the xenograft tumors, and 12.67 ± 3.126 a.u, 13.74 ± 2.878 a.u, 11.53 ± 4.401 a.u in the IRFA models (Figure 7C). There was no significant difference between the groups (Figures 7A,B). The washout half-time of NBCSF–1R, NBCTRL, and Sonovue® in the xenograft tumors was 29.17 ± 1.08 min, 15.87 ± 1.05 min, 3.35 ± 0.16 min (Figure 7D), and 26.84 ± 0.44 min, 6.71 ± 0.07 min, 2.89 ± 0.44 min in IRFA models (Figure 7D). Therefore, in the xenograft tumors and IRFA models, the washout half-time (Figure 7D, p = 0.05) was significantly different between NBCSF–1R, NBCTRL, and Sonovue®. As shown in Figures 7A,B, even after 30 min, the NBCSF–1R contrast agent can still enhanced efficiently in xenograft tumors and IRFA models, which implied that it has a longer circulation time in vivo.
Figure 7. (A) In vivo tumor imaging, images were taken at the indicated time points (0, immediate, 5, 15, and 30 min) after NBCSF–1R, NBCTRL, and Sonovue® were injected into tail vein of the transplanted tumors. (B) In vivo tumor imaging, images were taken at the indicated time points (0, immediate, 5, 15, and 30 min) after NBCSF–1R, NBCTRL, and Sonovue® were injected into tail vein of insufficient radiofrequency ablation (IRFA) models. Perfusion imaging was also taken to show the nanobubble diffusion. (C) The peak intensity of NBCS–F1R, NBCTRL, and Sonovue® was showed and there was no significant difference in each group. (D) The washout half-time in six group was show, and the NBCSF–1R can provide longer imaging time in the xenograft tumor and IRFA models (**indicates p < 0.05, ***indicates p < 0.001).
In the xenograft tumors, the echo signal intensity of NBCSF–1R, NBCTRL, and Sonovue® in the peritumoral tissues and tumor center are shown (Figure 8). The results indicated that the intensity of the peritumoral echo signal of NBCSF–1R was significantly higher than that of the central tissue (Figures 8A,B, p = 0.05) at the peak time, 5, and 15 min.
Figure 8. (A) The echo intensity fitting curve of the same area around or in the center of transplant tumor with NBCSF–1R, NBCTRL, and Sonovue®. The intensity of the peritumoral echo signal of the NBCSF–1R was significantly higher than that of the central tissue at the peak time, 5 and 15 min (p = 0.05). Data was shown in panel (B).
Colony-stimulating factor 1 receptor deposits were detected at the boundary of the tumor (Figure 9A), and were also detected at boundaries of the residual tumor after IRFA (Figure 9C). However, there were few deposits detected at the center of the tumor tissue (Figure 9B) or the residual tumor tissue (Figure 9D). The fluorescence intensity at the peritumor was higher than the tumor center. Therefore, similar to the human HCC spatial infiltration profiles, CSF-1R expressed in murine HCC were also abundant at the outer margins of the tumors. These results support the potential of using CSF-1R as a cancer imaging biomarker of macrophages.
Figure 9. The distribution of CSF-1R in xenograft tumor observed by fluorescence immunoassay, higher fluorescence intensity was observed at the peritumor, lower fluorescence intensity was observed at the tumor center. [(A) boundary of the tumor, (B) central of tumor, (C) boundary of the residual tumor after IRFA, and (D) residual tumor after IRFA. With a scale bar of 200 μm. The yellow line showed the tumor margin].
Researches have shown that RFA can lead to acute serologic elevation of active cytokines such as IL-6, nMDSC, and mMDSC, and a sustained high infiltration level of macrophages in the residual tumor (Shi et al., 2019; Sugimoto et al., 2019). In this study, CSF-1R was found highly expressed at the tumor boundary in patients with HCC, and also highly expressed in macrophages, but not tumor cells; making CSF-1R a feasible target. Frozen sections of the tumors revealed that macrophages were mostly located at the boundary of the xenograft tumors and residual tissue after performing IRFA.
Nanobubble CSF-1R had an average size of about 428 nm and were ultrasound-visible even at 20 MHz both in vitro and in vivo; imaging was still viable even when diluted 2000 times. Notably, NBs were administered at a low concentration compared with our previous research and other studies (Wischhusen et al., 2018), a technique which can be employed to reduce the level of background signal and modulate facilitate the comparison of heterogeneous tumor models (Wang et al., 2010, 2016).
To gain the insight functions of NBCSF–1R, we explored the specificity and efficiency of targeting of NBs in SMMC-7721 cells and macrophages. The results confirmed that the CSF-1R antibody could bound onto NBs efficiently; and the resulting NBCSF–1R were stable and target specific. In an in vitro cell binding experiment, these NBCSF–1R were identified to aggregate selectively surrounding macrophages but not SMMC-7721 cells, implying that the attachment of NBs to CSF-1R-positive macrophages contributes to interactions between antigen and antibody. Moreover, unconjugated NBs did not bind to macrophages, suggesting that the CSF-1R antibodies conjugating on the surface of the NBs were able to specifically recognize and improve adhesion to macrophages with high CSF-1R expression. In vivo, non-invasive imaging modality can be applied in extra-vascular region once NBs penetrate deep into the tumor neovasculature with a feature of a maximum pore size of approximately 380—780; this is because a basement membrane and smooth muscle absent and the intercellular space expands in cancer vasculature (Maeda, 2015).
Reduction in the size of the MBs not only decreases its echogenicity under clinical ultrasound but also cause instability (Sheeran et al., 2013). In our in vivo imaging experiments, however, showed that the peak intensity of NBCSF–1R, NBCTRL, and Sonovue® had no statistical difference in the xenograft tumor models and IRFA models (Figure 7C). This is probably due to the fact that lipid contrast agents can produce preferable harmonic signal intensity (Postema and Schmitz, 2007), and nanoparticles could be accumulate within tumor tissue through the enhanced permeability and retention (EPR) effect and then were transformed into micro-sized echogenic bubbles (Min et al., 2016). These microbubbles at targeted tumor tissues could serve as new echogenic particles for cancer-targeting ultrasound imaging.
With the application of acoustic radiation forces (ARF) to upregulate contrast agent binding (Zhao et al., 2004), molecular ultrasound imaging is constantly improving. Frinking et al. (2012) manifested enhanced adhesion of targeted MB in vivo upon ARF performed in experimental models of cancer. In comparison with normal vessels, they found an increased binding of VEGFR2-targeted MB (BR55) in the vasculature of experiment (Frinking et al., 2012). In this study, in the xenograft tumor model and IRFA model, the washout half-time ratio of NBCSF–1R to NBs was two times higher, and about nine times higher compared to Sonovue®. Furthermore, being a nanoparticle, NBCTRL and NBCSF–1R could accumulate at the targeted tumor tissue via the EPR effect, and NBCSF–1R can abound onto higher CSF-1 expression cells effectively. The adherent NBCSF–1R maintained visible for a long time, contributing to a longer persistence of enhanced contrast compared to NBCTRL and Sonovue®. This result further verifies that the duration of contrast enhancement may be applied as an indicator for the investigation of targeted NBs enhanced imaging. The molecular imaging would also be helpful in finding the residual tumor after IRFA. With long-term stability, NBCSF–1R could be used to evaluate the boundary of the tumor when performing RFA.
In this study, a uniform nano-sized lipid NBs was prepared, and could successfully combined the NBs with biotinylated anti-CSF-1R. The NBCSF–1R which was small and stable as well as high specificity for the molecule that is overexpressed in macrophages. We demonstrated the high specificity of our NBCSF–1R on targeting CSF-1R overexpressing macrophages and HCC tumor margin. In vitro and in vivo studies demonstrated that NBCSF–1R exhibited effective ultrasound imaging capabilities in evaluating the RFA response, which can be used to detect the residual HCC after RFA, opening a possibility of clinical translation of a non-invasive diagnosis method for IRFA.
All datasets generated for this study are included in the article/supplementary material.
The animal study was reviewed and approved by Ethics Committee of Sun Yat-sen Memorial Hospital and Ethics Committee of Zhongshan School of Medicine (ZSSOM) on Laboratory Animal Care, Sun Yat-sen University.
HL and BZ performed animal imaging analysis. YK performed statistical analysis. PS and BL designed and oversaw all the experiments and wrote the manuscript. All authors contributed to the article and approved the submitted version.
This study was supported by the National Natural Science Foundation of China (81701715, 81671704, and 81873899); the Science Foundation of Guangdong Province (2017A030310200, 2016A030313306, 2017A030313727, and 2018A030313097); and the People’s Livelihood and Technology Planning Project of Guangzhou City of China (201803010035).
The authors declare that the research was conducted in the absence of any commercial or financial relationships that could be construed as a potential conflict of interest.
We thank all the researchers and staff of the Guangdong Provincial Key Laboratory of Malignant Tumor Epigenetics and Gene Regulation for their contribution to this study, especially the flow cytometric analysis laboratory.
Chen, W., Zheng, R., Baade, P. D., Zhang, S., Zeng, H., Bray, F., et al. (2016). Cancer statistics in China, 2015. CA Cancer J. Clin. 66, 115–132. doi: 10.3322/caac.21338
Dai, H., Jia, G., Wang, H., Yang, J., Jiang, H., and Chu, M. (2017). Epidermal growth factor receptor transactivation is involved in the induction of human hepatoma SMMC7721 cell proliferation by insufficient radiofrequency ablation. Oncol. Lett. 14, 2463–2467. doi: 10.3892/ol.2017.6463
Frinking, P. J., Tardy, I., Theraulaz, M., Arditi, M., Powers, J., Pochon, S., et al. (2012). Effects of acoustic radiation force on the binding efficiency of BR55, a VEGFR2-specific ultrasound contrast agent. Ultrasound Med. Biol. 38, 1460–1469. doi: 10.1016/j.ultrasmedbio.2012.03.018
Guvener, N., Appold, L., de Lorenzi, F., Golombek, S. K., Rizzo, L. Y., Lammers, T., et al. (2017). Recent advances in ultrasound-based diagnosis and therapy with micro- and nanometer-sized formulations. Methods 130, 4–13. doi: 10.1016/j.ymeth.2017.05.018
Jia, J. B., Wang, W. Q., Sun, H. C., Zhu, X. D., Liu, L., and Zhuang, P. Y. (2010). High expression of macrophage colony-stimulating factor-1 receptor in peritumoral liver tissue is associated with poor outcome in hepatocellular carcinoma after curative resection. Oncologist 15, 732–743. doi: 10.1634/theoncologist.2009-0170
Jiang, Q., Hao, S., Xiao, X., Yao, J., Ou, B., Zhao, Z., et al. (2016). Production and characterization of a novel long-acting Herceptin-targeted nanobubble contrast agent specific for Her-2-positive breast cancers. Breast Cancer Tokyo 23, 445–455. doi: 10.1007/s12282-014-0581-8
Kang, T. W., Kim, J. M., Rhim, H., Lee, M. W., Kim, Y. S., and Lim, H. K. (2015). Small hepatocellular carcinoma: radiofrequency ablation versus nonanatomic resection–propensity score analyses of long-term outcomes. Radiology 275, 908–919. doi: 10.1148/radiol.15141483
Kim, Y. S., Lee, W. J., Rhim, H., Lim, H. K., Choi, D., and Lee, J. Y. (2010). The minimal ablative margin of radiofrequency ablation of hepatocellular carcinoma (>2 and <5 cm) needed to prevent local tumor progression: 3D quantitative assessment using CT image fusion. AJR Am. J. Roentgenol. 195, 758–765. doi: 10.2214/AJR.09.2954
Kong, L. Q., Zhu, X. D., Xu, H. X., Zhang, J. B., Lu, L., and Wang, W. Q. (2013). The clinical significance of the CD163+ and CD68+ macrophages in patients with hepatocellular carcinoma. PLoS One 8:e59771. doi: 10.1371/journal.pone.0059771
Krupka, T. M., Solorio, L., Wilson, R. E., Wu, H., Azar, N., and Exner, A. A. (2010). Formulation and characterization of echogenic lipid-Pluronic nanobubbles. Mol. Pharm. 7, 49–59. doi: 10.1021/mp9001816
Leftin, A., Ben-Chetrit, N., Joyce, J. A., and Koutcher, J. A. (2019). Imaging endogenous macrophage iron deposits reveals a metabolic biomarker of polarized tumor macrophage infiltration and response to CSF1R breast cancer immunotherapy. Sci. Rep. 9:857. doi: 10.1038/s41598-018-37408-7
Lewis, C. E., and Pollard, J. W. (2006). Distinct role of macrophages in different tumor microenvironments. Cancer Res. 66, 605–612. doi: 10.1158/0008-5472.CAN-05-4005
Li, X., Wang, D., Ran, H., Hao, L., Cao, Y., Ao, M., et al. (2018). A preliminary study of photoacoustic/ultrasound dual-mode imaging in melanoma using MAGE-targeted gold nanoparticles. Biochem. Biophys. Res. Commun. 502, 255–261. doi: 10.1016/j.bbrc.2018.05.155
Liu, R., Tang, J., Xu, Y., and Dai, Z. (2019). bioluminescence imaging of inflammation in vivo based on bioluminescence and fluorescence resonance energy transfer using nanobubble ultrasound contrast agent. ACS Nano 13, 5124–5132. doi: 10.1021/acsnano.8b08359
Liu, Z., Dai, H., Jia, G., Li, Y., Liu, X., and Ren, W. (2015). Insufficient radiofrequency ablation promotes human hepatoma SMMC7721 cell proliferation by stimulating vascular endothelial growth factor overexpression. Oncol. Lett. 9, 1893–1896. doi: 10.3892/ol.2015.2966
Maeda, H. (2015). Toward a full understanding of the EPR effect in primary and metastatic tumors as well as issues related to its heterogeneity. Adv. Drug Deliv. Rev. 91, 3–6. doi: 10.1016/j.addr.2015.01.002
Min, H. S., Son, S., You, D. G., Lee, T. W., Lee, J., Lee, S., et al. (2016). Chemical gas-generating nanoparticles for tumor-targeted ultrasound imaging and ultrasound-triggered drug delivery. Biomaterials 108, 57–70. doi: 10.1016/j.biomaterials.2016.08.049
Muralidhara, S., Malu, K., Gaines, P., and Budhlall, B. M. (2019). Quantum dot encapsulated nanocolloidal bioconjugates function as bioprobes for in vitro intracellular imaging. Colloids Surf. B Biointerfaces 182, 110348. doi: 10.1016/j.colsurfb.2019.110348
Nandi, S., Cioce, M., Yeung, Y. G., Nieves, E., Tesfa, L., and Lin, H. (2013). Receptor-type protein-tyrosine phosphatase zeta is a functional receptor for interleukin-34. J. Biol. Chem. 288, 21972–21986. doi: 10.1074/jbc.M112.442731
N’Kontchou, G., Mahamoudi, A., Aout, M., Ganne-Carrie, N., Grando, V., and Coderc, E. (2009). Radiofrequency ablation of hepatocellular carcinoma: long-term results and prognostic factors in 235 Western patients with cirrhosis. Hepatology 50, 1475–1483. doi: 10.1002/hep.23181
Postema, M., and Schmitz, G. (2007). Ultrasonic bubbles in medicine: influence of the shell. Ultrason. Sonochem. 14, 438–444. doi: 10.1016/j.ultsonch.2006.09.013
Pyonteck, S. M., Akkari, L., Schuhmacher, A. J., Bowman, R. L., Sevenich, L., Quail, D. F., et al. (2013). CSF-1R inhibition alters macrophage polarization and blocks glioma progression. Nat. Med. 19, 1264–1272. doi: 10.1038/nm.3337
Qian, B. Z., and Pollard, J. W. (2010). Macrophage diversity enhances tumor progression and metastasis. Cell 141, 39–51. doi: 10.1016/j.cell.2010.03.014
Shady, W., Petre, E. N., Gonen, M., Erinjeri, J. P., Brown, K. T., Covey, A. M., et al. (2016). Percutaneous radiofrequency ablation of colorectal cancer liver metastases: factors affecting outcomes–A 10-year experience at a single center. Radiology 278, 601–611. doi: 10.1148/radiol.2015142489
Sheeran, P. S., Streeter, J. E., Mullin, L. B., Matsunaga, T. O., and Dayton, P. A. (2013). Toward ultrasound molecular imaging with phase-change contrast agents: an in vitro proof of principle. Ultrasound Med. Biol 39, 893–902. doi: 10.1016/j.ultrasmedbio.2012.11.017
Shi, L., Wang, J., Ding, N., Zhang, Y., and Liao, W. (2019). Inflammation induced by incomplete radiofrequency ablation accelerates tumor progression and hinders PD-1 immunotherapy. Nat. Commun. 10:5421.
Sotirchos, V. S., Petrovic, L. M., Gonen, M., Klimstra, D. S., Do, R. K., and Petre, E. N. (2016). Colorectal cancer liver metastases: biopsy of the ablation zone and margins can be used to predict oncologic outcome. Radiology 280, 949–959. doi: 10.1148/radiol.2016151005
Sugimoto, K., Kakimi, K., Takeuchi, H., Fujieda, N., and Itoi, T. (2019). Irreversible electroporation versus radiofrequency ablation: comparison of systemic immune responses in patients with hepatocellular carcinoma. J. Vasc. Int. Radiol. 30, 845.e6–853.e6.
Tang, H., Zheng, Y., and Chen, Y. (2017). Materials chemistry of nanoultrasonic biomedicine. Adv. Mater. 29:1604105. doi: 10.1002/adma.201604105
Wang, L., Chen, S., Zhu, Y., Zhang, M., Tang, S., Li, J., et al. (2018). Triple-modal imaging-guided chemo-photothermal synergistic therapy for breast cancer with magnetically targeted phase-shifted nanoparticles. ACS Appl. Mater. Interfaces 10, 42102–42114. doi: 10.1021/acsami.8b16323
Wang, P., Wang, X., Luo, Q., Li, Y., Lin, X., Fan, L., et al. (2019). Fabrication of red blood cell-based multimodal theranostic probes for second near-infrared window fluorescence imaging-guided tumor surgery and photodynamic therapy. Theranostics 9, 369–380. doi: 10.7150/thno.29817
Wang, S., Herbst, E. B., Mauldin, F. J., Diakova, G. B., Klibanov, A. L., and Hossack, J. A. (2016). Ultra-low-dose ultrasound molecular imaging for the detection of angiogenesis in a mouse murine tumor model: how little can we see? Invest. Radiol. 51, 758–766. doi: 10.1097/RLI.0000000000000310
Wang, X., Sofocleous, C. T., Erinjeri, J. P., Petre, E. N., Gonen, M., and Do, K. G. (2013). Margin size is an independent predictor of local tumor progression after ablation of colon cancer liver metastases. Cardiovasc. Intervent. Radiol. 36, 166–175. doi: 10.1007/s00270-012-0377-1
Wang, Y., Li, X., Zhou, Y., Huang, P., and Xu, Y. (2010). Preparation of nanobubbles for ultrasound imaging and intracelluar drug delivery. Int. J. Pharm. 384, 148–153. doi: 10.1016/j.ijpharm.2009.09.027
Wischhusen, J., Wilson, K. E., Delcros, J. G., Molina-Pena, R., Gibert, B., Jiang, S., et al. (2018). Ultrasound molecular imaging as a non-invasive companion diagnostic for netrin-1 interference therapy in breast cancer. Theranostics 8, 5126–5142. doi: 10.7150/thno.27221
Zhang, N., Li, H., Qin, C., Ma, D., Zhao, Y., Zhu, W., et al. (2019). Insufficient radiofrequency ablation promotes the metastasis of residual hepatocellular carcinoma cells via upregulating flotillin proteins. J. Cancer Res. Clin. Oncol. 145, 895–907. doi: 10.1007/s00432-019-02852-z
Zhao, S., Borden, M., Bloch, S. H., Kruse, D., Ferrara, K. W., and Dayton, P. A. (2004). Radiation-force assisted targeting facilitates ultrasonic molecular imaging. Mol. Imaging 3, 135–148. doi: 10.1162/1535350042380317
Zhou, B., Jiang, Q., Xiao, X., Xu, X., Xu, Y., Kong, Y., et al. (2019). Assisting anti-PD-1 antibody treatment with a liposomal system capable of recruiting immune cells. Nanoscale 11, 7996–8011. doi: 10.1039/c9nr01434a
Zhu, X. D., Zhang, J. B., Zhuang, P. Y., Zhu, H. G., Zhang, W., and Xiong, Y. Q. (2008). High expression of macrophage colony-stimulating factor in peritumoral liver tissue is associated with poor survival after curative resection of hepatocellular carcinoma. J. Clin. Oncol. 26, 2707–2716. doi: 10.1200/JCO.2007.15.6521
Keywords: ultrasound imaging, HCC tumor margin, non-invasive tumor margin detection, CSF-1R targeting, macrophage
Citation: Jiang Q, Zeng Y, Xu Y, Xiao X, Liu H, Zhou B, Kong Y, Saw PE and Luo B (2020) Ultrasound Molecular Imaging as a Potential Non-invasive Diagnosis to Detect the Margin of Hepatocarcinoma via CSF-1R Targeting. Front. Bioeng. Biotechnol. 8:783. doi: 10.3389/fbioe.2020.00783
Received: 10 May 2020; Accepted: 22 June 2020;
Published: 14 July 2020.
Edited by:
Jianxun Ding, Changchun Institute of Applied Chemistry (CAS), ChinaReviewed by:
Wenjun Miao, Nanjing Tech University, ChinaCopyright © 2020 Jiang, Zeng, Xu, Xiao, Liu, Zhou, Kong, Saw and Luo. This is an open-access article distributed under the terms of the Creative Commons Attribution License (CC BY). The use, distribution or reproduction in other forums is permitted, provided the original author(s) and the copyright owner(s) are credited and that the original publication in this journal is cited, in accordance with accepted academic practice. No use, distribution or reproduction is permitted which does not comply with these terms.
*Correspondence: Phei Er Saw, Y2FpcGVpZUBtYWlsLnN5c3UuZWR1LmNu; Baoming Luo, bHVvYm1AbWFpbC5zeXN1LmVkdS5jbg==
†These authors have contributed equally to this work
Disclaimer: All claims expressed in this article are solely those of the authors and do not necessarily represent those of their affiliated organizations, or those of the publisher, the editors and the reviewers. Any product that may be evaluated in this article or claim that may be made by its manufacturer is not guaranteed or endorsed by the publisher.
Research integrity at Frontiers
Learn more about the work of our research integrity team to safeguard the quality of each article we publish.