- 1State Key Laboratory of Microbial Metabolism, Joint International Research Laboratory of Metabolic & Developmental Sciences, School of Life Sciences and Biotechnology, Shanghai Jiao Tong University, Shanghai, China
- 2Biorefinery and Bioproduct Research Group, Enzyme Technology Laboratory, National Center for Genetic Engineering and Biotechnology, Pathum Thani, Thailand
Synthetic biology studies on filamentous fungi are providing unprecedented opportunities for optimizing this important category of microbial cell factory. Artificial transcription factor can be designed and used to offer novel modes of regulation on gene transcription network. Trichoderma reesei is commonly used for cellulase production. In our previous studies, a plasmid library harboring genes encoding artificial zinc finger proteins (AZFPs) was constructed for engineering T. reesei, and the mutant strains with improved cellulase production were selected. However, the underlying mechanism by which AZFP function remain unclear. In this study, a T. reesei Rut-C30 mutant strain T. reesei U5 bearing an AZFP named as AZFP-U5 was focused, which secretes high level protein and shows significantly improved cellulase and xylanase production comparing with its parental strain. In addition, enhanced sugar release was achieved from lignocellulosic biomass using the crude cellulase from T. reesei U5. Comparative transcriptome analysis was further performed, which showed reprogramming of global gene transcription and elevated transcription of genes encoding glycoside hydrolases by overexpressing AZFP-U5. Furthermore, 15 candidate regulatory genes which showed remarkable higher transcription levels by AZFP-U5 insertion were overexpressed in T. reesei Rut-C30 to examine their effects on cellulase biosynthesis. Among these genes, TrC30_93861 (ypr1) and TrC30_74374 showed stimulating effects on filter paper activity (FPase), but deletion of these two genes did not affect cellulase activity. In addition, increased yellow pigment production in T. reesei Rut-C30 by overexpression of gene ypr1 was observed, and changes of cellulase gene transcription were revealed in the ypr1 deletion mutant, suggesting possible interaction between pigment production and cellulase gene transcription. The results in this study revealed novel aspects in regulation of cellulase gene expression by the artificial regulators. In addition, the candidate genes and processes identified in the transcriptome data can be further explored for synthetic biology design and metabolic engineering of T. reesei to enhance cellulase production.
Introduction
Cellulase is widely used in detergents, textiles, pulp processing, food, and feed industries (Sharma et al., 2016). The application of cellulase in lignocellulosic biorefinery for producing renewable biofuels and biochemicals has particularly received widespread interest owning to the gradual depletion of fossil fuels and environmental concerns (Tiwari et al., 2018). Efficient production of cellulases is essential for reducing the bioconversion cost of lignocellulosic biomass. Trichoderma reesei (an anamorph of Hypocrea jecorina) and its derivative strains have been widely used for cellulase production (Bischof et al., 2016). However, so far high cellulase production cost is still a bottleneck for practical applications.
The T. reesei genome commonly contains over 220 putative genes encoding candidate carbohydrate-active enzymes (CAZymes), which include cellulases such as cellobiohydrolases (CBH), endoglucanases (EG), and β-glucosidases (BGL), as well as hemicellulases. In addition, auxiliary proteins that hydrolyze lignocellulose synergistically are also important for biodegradation of cellulosic feedstocks (Hakkinen et al., 2012). Cellulase biosynthesis by T. reesei is induced by various inducers such as cellulose, sophorose, lactose, and cellobiose, but is repressed by glucose. Besides these carbon sources, cellulase production is also responsive to a variety of environmental and physiological factors, such as Ca2+, light, pH, and physiological state of the mitochondria (Abrahao Neto et al., 1995; He et al., 2014; Chen et al., 2016; Schmoll, 2018). On the other hand, transcription factors also play important roles in cellulase production. So far, several key regulatory factors were well-characterized. Transcription factor Xyr1 is the major positive regulator governing a complex regulatory network for expression of (hemi-) cellulase genes, and recently, the function of Ace3 is characterized for cellulase production, and its cross talk with Xyr1 and Crt1 was revealed (Zhang et al., 2019). Furthermore, other regulators, such as Ace2, BglR, and Vib1 have also been identified to regulate cellulase production (Aro et al., 2001; Nitta et al., 2012; Zhang et al., 2018a). Recently, our group identified a novel repressor Ctf1 for cellulase biosynthesis in T. reesei Rut-C30 (Meng et al., 2020). In addition, transcription factor Cre1 mediates carbon catabolite repression (CCR) to suppress cellulase expression (Nakari-Setala et al., 2009). However, the detailed regulatory mechanisms of cellulase production from T. reesei are known to be complicated and are still not fully clear.
Synthetic biology studies of T. reesei are still in its early stage, but have opened new opportunities for metabolic engineering of this important species (Druzhinina and Kubicek, 2017; Liu and Qu, 2019). Artificial transcription factors (ATFs) could perturb and remodel the innate regulatory network, leading to new phenotypes (Yang et al., 2019). Several chimeric transcription factors were reported to have positive effects on cellulase production for T. reesei, which usually comprised DNA binding domains from characterized regulators with different effecter domains, such as minimal transcriptional activators comprising DBDace2(cre1)-linker-VP16AD, regulators DBDxyr1-EDypr1, and Xyr1-DBDcre1 (Zhang et al., 2017, 2018b; Derntl et al., 2019). Also, some novel fused regulators containing DBDcre1 and activation domains of Ace3, Clr2, Ace2, or Xyr1 were found to regulate the cellulase production profile in T. reesei, especially for CBH1 (Wang et al., 2019).
In our previous studies, the artificial zinc finger protein (AZFP) AZFP-U3 containing Cyc2-His2 type DNA binding domain significantly enhanced cellulase production in T. reesei Rut-C30 (Zhang et al., 2016). In our recent report, AZFP-M2 also led to improved cellulase activity in T. reesei Rut-C30 (Meng et al., 2020), but these two AZFPs showed different stimulating patterns on cellulase compositions. It will be of great interest to test more AZFPs for their regulatory functions, and reveal how the ATFs regulate cellulase production in T. reesei.
In this study, an additional AZFP which we named AZFP-U5 was identified to have a positive effect on cellulase production in T. reesei Rut-C30, whose feature on the regulatory network was further analyzed by comparative transcriptome analysis. Meanwhile, candidate regulatory genes related to cellulase production were further explored. The results in this study would provide novel insights in synthetic biology design and metabolic engineering of T. reesei for cellulase production.
Materials and Methods
Strains and Culture Media
T. reesei Rut-C30 was obtained from ARS Culture Collection (NRRL, Peoria, IL). Conidial suspensions were prepared by cultivating the strain on malt extract agar (MEA) medium (20 g/L Malt extract, 20 g/L Agar) at 28°C for 7 days. After harvesting, the conidia were suspended in 20% glycerol, which were then gauze-filtered, and stored at −80°C before use. Mandels-Andreotti (MA) medium (Mandels and Andreotti, 1978) containing 2% (m/v) microcrystalline cellulose (Merck, Germany) and 2% (m/v) wheat bran was used as a cellulase production medium. To induce cellulase production in the presence of soluble carbon sources, the carbon source in MA medium was replaced with 2% (m/v) glucose or 2% (m/v) lactose.
For cellulase production, the conidia of Trichoderma strains were collected from MEA medium, and cultured in 50 ml MA medium in a 250 mL Erlenmeyer flask supplemented with 0.1% (w/v) peptone and 2% (w/v) glucose to induce spore germination. After pre-cultivation at 28°C and 150 rpm in a rotary shaker for 24 h, 5 mL mycelia were taken from the germination medium and were added into 45 mL cellulase production medium in a 250 mL flask (10% inoculation size).
Escherichia coli DH5α was used for plasmid propagation and DNA manipulation. Agrobacterium tumefaciens AGL-1 was used for the A. tumefaciens-mediated genetic transformation (ATMT) of T. reesei. Transformation of A. tumefaciens AGL-1 with the pCB303-ZFP library and screening of T. reesei mutants were performed using the methods descried previously (Zhang et al., 2016). The T. reesei U5 mutant strain selected from the mutants was preserved in China General Microbiological Culture Collection Center (CGMCC) with the accession number of CGMCC 10649.
Determination of Cellulase Activity, Protein Concentration, and Sporulation Assay
The T. reesei cultured sample (1 mL) was collected each day and centrifuged at 10,000 rpm for 5 min, from which the supernatant was used for cellulase activity measurement and protein concentration assay. Filter paper activity (FPase activity), endoglucanase (CMCase) activity, and β-glucosidase activity were measured according to the standard IUPAC procedures. The FPase activity is expressed as filter paper units, one unit of filter paper enzymatic activity is defined as the amount of enzyme that releases 1 μmol of glucose per minute from 50 mg filter paper stripe. The total xylanase activity was assayed with 1% beechwood xylan (Sigma-Aldrich, USA) as the substrate according to the standard protocol (Bailey et al., 1992). Concentration of extracellular protein was measured by the Bio-Rad Protein Assay Kit (Bio-Rad, USA) following the manufacturer's instructions.
For sporulation assay, an aliquot of 10 μL (108/L) spores of the T. reesei strains were cultivated on MEA plates at 28°C for 7 days to obtain mature conidia, which were collected and counted using a hemocytometer.
Construction of T. reesei Mutants Overexpressing and Deleting Various Putative Regulatory Genes
All primers used for strain construction and confirmation were listed in Table S1. In order to study the effect of AZFP-U5 on cellulase synthesis in T. reesei, its nucleic acid sequence was amplified by PCR with the primers pCB303-U5-F and pCB303-U5-R, and the PCR product was ligated into the expression vector pCB303, then transformed into T. reesei Rut-C30 using the ATMT method. After confirmation of the correct transformants, the cellulase activities of at least three randomly selected transformants were determined.
For construction of the T. reesei mutants overexpressing endogenous putative regulators, the PCR products of 15 corresponding putative regulatory genes amplified from genomic cDNA were fused, respectively, into the pCZF3 vector (Zhang et al., 2018a) digested by Nco I and Xba I using Seamless Cloning Master Mix (Sangon Biotech, China). Each ligated product was then transformed into T. reesei Rut-C30 using the ATMT method, putative regulatory genes were overexpressed individually, driven by the pyruvate decarboxylase (pdc) promoter.
Regarding the construction of TrC30_93861 (ypr1) or TrC30_74374 knockout strains, the 2,000-bp upstream and downstream region of the corresponding genes were amplified from T. reesei Rut-C30 genome as the homologous arms individually. The hygromycin B resistance gene hph was employed as the selected marker, and the expression cassette was amplified from the pCZF3 vector using primers hphCAS-F and hphCAS-R. Then, the hph expression cassette together with the 2,000-bp flanking arms were cloned into plasmid pZF-MCS at Hind III and EcoR I sites using ClonExpress™ II One Step Cloning Kit (Vazyme, China), resulting in the plasmid pZF-93861D and pZF-74374D. The plasmids pZF-93861D and pZF-74374D were then introduced into T. reesei Rut-C30 Δku70 by the ATMT method. The correct deletion mutants were verified using four pairs of specific deletion primers, and the design scheme for strain constructions and verifications are provided in Figure S3.
Determination of the Copy Number and Integrated Site of AZFP-U5
To determine the copy number of the AZFP encoding gene AZFP-U5 in the T. reesei U5, genomic DNA of T. reesei U5 was isolated and used as the template for quantitative PCR (qPCR) with the primers AZFPU5-F and AZFPU5-R. The qPCR method is the same as that described by Solomon et al. (2008), in which tef1α (translation elongation factor 1-alpha) was used as a control for a single copy. Correspondingly, the integrated site of the AZFP-U5 was determined by the thermal asymmetric interlaced PCR (TAIL-PCR) according to the procedure described elsewhere (Liu and Chen, 2007). Additionally, the copy number of hph expression cassette in respective regulator deletion mutants was verified by qPCR with the primers hphqPCR-F and hphqPCR-R. The primers used for qPCR and TAIL-PCR are listed in Table S2.
Comparative Transcriptome Analysis
T. reesei U5 and Rut-C30 were grown in the MA medium with cellulose as the carbon source, then, the total RNAs from the mycelia were isolated at 24 and 48 h using the RNA extraction kit (Sangon Biotech, China). RNA sequencing (RNA-Seq) was performed at the BGI Company (Shenzhen, China) using the Illumina HiSeq2000 system. Gene expression levels were calculated using the fragments per kilobase of exon model per million reads (FPKM) method (Mortazavi et al., 2008). Threshold values of |log2 FC (fold change of U5 to Rut-C30)| ≥1 and FDR (False Discovery Rate) <0.001 were used to judge the significance. Genes were annotated according to the T. reesei Rut-C30 genome annotation, and proteins involved in CAZymes, transcription factors, transporters, secondary metabolism clusters, protein processing, and secretion were referred to genome database of Joint Genome Institute (https://mycocosm.jgi.doe.gov/pages/search-for-genes.jsf?organism=TrireRUTC30_1) (Grigoriev et al., 2014).
To elucidate the effects of ypr1 in pigment production, T. reesei Δypr1 and its parent strain T. reesei Rut-C30 Δku70 were cultured in the MA medium with 2% glucose as a carbon source, then the mycelia of these two strains at 24 h were collected for total RNA extraction. The process of transcriptome analysis for T. reesei Δypr1 and Rut-C30 Δku70 is the same as described above for T. reesei U5 and Rut-C30. The mRNA profile for specific genes was also analyzed for verification of RNA-seq results with corresponding primers (Table S1). The NCBI accession numbers for transcriptome data of T. reesei U5 and Rut-C30, T. reesei Δypr1 and Rut-C30 Δku70 are PRJNA612708 and PRJNA613769, respectively.
Enzymatic Hydrolysis of the Pretreated Biomass
Biomass hydrolysis efficiency of cellulase produced by T. reesei was measured using alkaline pretreated corn stover (APCS). Corn stover was pretreated by 2% (w/v) sodium hydroxide and 2%(w/v) hydrogen peroxide, washed to reach neutral pH, and lignocellulose components and hydrolysis were measured according to the method described previously (Zhang et al., 2016). Hydrolysis experiment was carried out at 5% total solids loading with APCS as the substrate, and the cellulase loading was 30 mg per gram of dry biomass. The hydrolysis conditions are incubation at 50°C, pH 4.8, shaking at 150 rpm for 48 h, and samples were collected at an interval of 12 h. The sugar ingredients and concentrations released in the hydrolysate were analyzed by HPLC. The experiments were performed in triplicate, and the results are represented as the average values ± standard deviation (SD).
Results
Cellulase Properties of T. reesei Rut-C30 and U5
The mutant strain T. reesei U5 with improved cellulase production was selected from the T. reesei Rut-C30 transformants transformed by the AZFP library (Zhang et al., 2016). The AZFP sequence from the transformant T. reesei U5 was named as AZFP-U5, and the corresponding gene AZFP-U5 was found to integrate into the genome as a single copy. The single integration site was identified to be located at the scaffold 33: 124903–124921 of T. reesei Rut-C30 chromosome, which is the intergenic region between terminators of two genes of TrC30_91501 and TrC30_91570, indicating that AZFP-U5 functions without disrupting other functional genes.
With cellulose as the carbon source in the MA medium, the FPase activity of T. reesei U5 was 2.1-folds of that from T. reesei Rut-C30, reaching 3.31 U/mL on the 7th day, while the FPase activity in the parental strain was 1.56 U/mL (Figure 1A). In addition to increasing the total FPase activity, U5 cellulase also exhibited superior profile for various cellulase components. As shown in Figure S1, the endoglucanase activity of T. reesei U5 reached 17.23 U/mL, increased by 1.88 times to that from Rut-C30. The activity of β-glucosidase secreted by U5 strain was 2.76 U/mL, much higher than that from T. reesei Rut-C30 (1.09 U/mL). The extracellular xylanase activities of T. reesei U5 and Rut-C30 were 596 and 388 U/mL, respectively. Meanwhile, the extracellular proteins of those two strains were significantly different, the amount of protein secretion from T. reesei U5 was increased by 86% relative to that from Rut-C30. The extracellular protein concentration from T. reesei U5 reached 2.62 mg/mL, while it was about 1.41 mg/mL for Rut-C30 (Figure 1B). When the re-constructed AZFP-U5 plasmid was transformed into T. reesei Rut-C30, the enhanced cellulase production phenotypes were observed in all three randomly selected mutants.
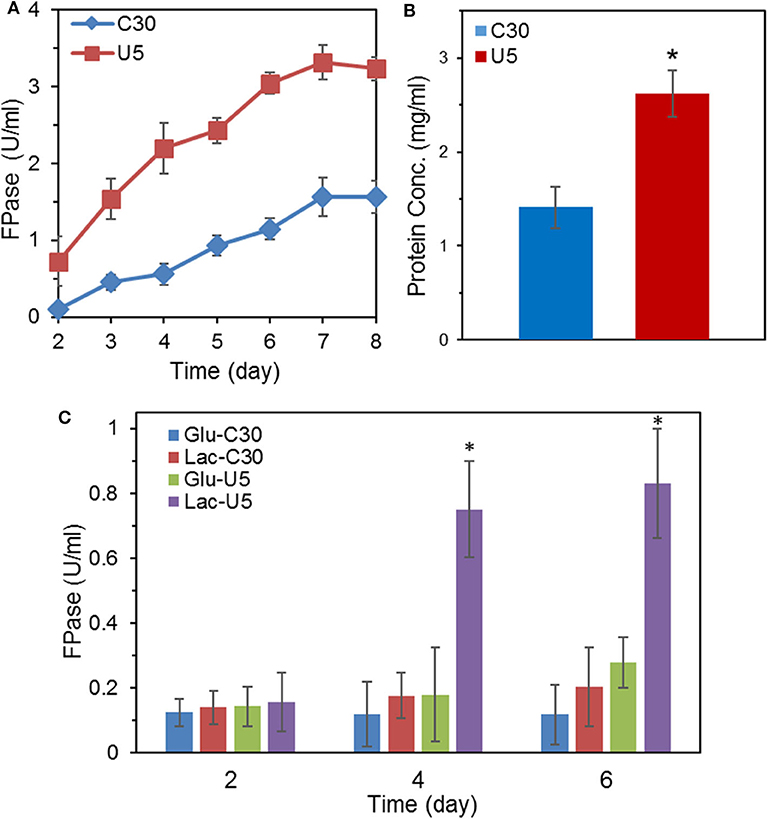
Figure 1. Comparison of FPase activities and secreted protein concentration between the mutant T. reesei U5 and the parental strain Rut-C30. (A) FPase activities of T. reesei U5 and Rut-C30 in MA medium with 2% cellulose and 2% wheat bran as carbon source. (B) Extracellular protein concentration of T. reesei U5 and Rut-C30 at 7th day's fermentation. (C) FPase activities of T. reesei U5 and Rut-C30 in MA medium with 2% glucose or lactose as a carbon source. Asterisks indicate significant differences from reference strains (*p < 0.05, Student's t-test).
In addition to cellulose, lactose also has an inducing effect on cellulase production. The FPase activities between cellulase U5 and Rut-C30 were found to be significantly different in the fermentation medium containing 2% glucose or lactose as carbon sources, as shown in Figure 1C. On the 6th day of fermentation in glucose medium, the FPase activity from U5 cellulase reached 0.28 U/mL, much higher than that in T. reesei Rut-C30 (0.12 U/mL). The FPase activities of cellulase U5 and Rut-C30 in the lactose medium were 0.83 and 0.20 U/ml, respectively, and over 4 times improvement was observed in cellulase U5 compared to that from Rut-C30, which demonstrates that T. reesei U5 partially abolished the CCR effect in the presence of glucose and lactose.
The hydrolytic ability for pretreated lignocellulose was further evaluated. In the course of hydrolysis on alkali pretreated corn stover by crude cellulase from T. reesei Rut-C30 and U5, the released glucose reached 35.22 g/L (corresponding to ~95% cellulose conversion) by the crude cellulase from T. reesei U5, which increased by 33.9% compared to that from Rut-C30 (26.30 g/L) at 48 h. Meanwhile, 31% higher xylose yields were also observed by the cellulase produced by T. reesei U5. At 48 h, slightly lower residual cellobiose was observed using the cellulase U5 (1.65 vs. 2.03 g/L from the parental strain) (Figure 2).
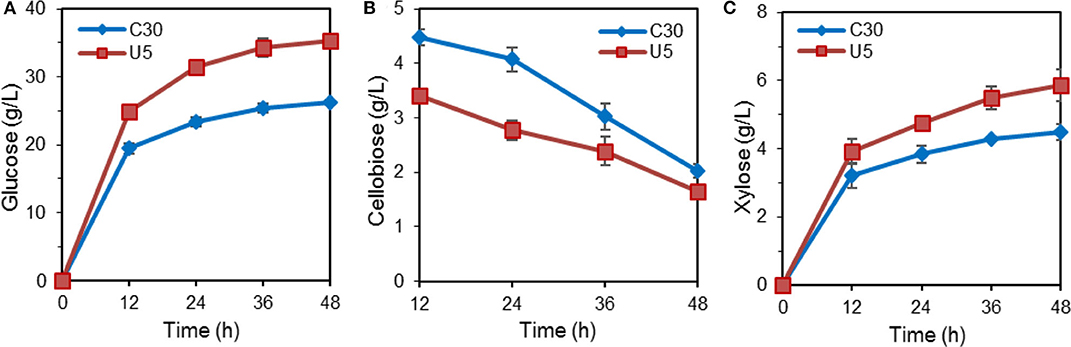
Figure 2. Hydrolysis of pretreated corn stalk by cellulase produced by T. reesei U5 and Rut-C30. (A) Glucose releasing concentration. (B) Cellobiose releasing concentration. (C) Xylose releasing concentration.
Transcriptional Changes of Major Cellulases and Transcription Factors
As shown in Figure 3, transcription levels of the five major cellulase components in T. reesei U5, including the major cellulase genes cbh1/2, eg1/2, and bgl1, increased significantly (2–16-fold) compared with that of T. reesei Rut-C30. In the U5 strain, the transcript of bgl1 gene increased over 1-fold, and transcription of the endoglucanse genes eg1 and eg2 increased by 3-fold at 24 h compared to that in T. reesei Rut-C30. At 48 h, the transcription levels of main cellulase genes in T. reesei U5 increased by 4–16 times compared to that from Rut-C30, and the transcription levels of the two major xylanase genes xyn1 and xyn2 improved 32 and 128 times, respectively. Meanwhile, transcription of the major transcription factor xyr1 gene also increased, of which the transcript in T. reesei U5 was 5-fold to that in Rut-C30 at 48 h. The transcription levels of other regulators for cellulase regulation were also remarkably increased, which include ace1, ace2, and bglr.
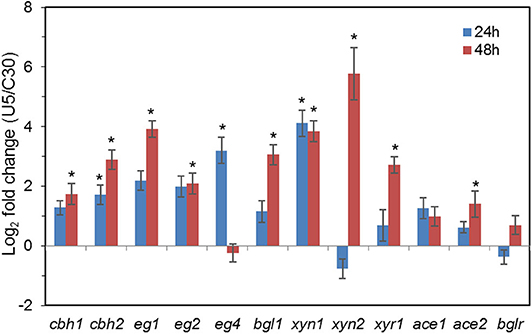
Figure 3. Transcription of genes encoding major cellulases and transcription factors in T. reesei U5 and Rut-C30. Asterisks indicate significant differences from reference strains (*p < 0.05, Student's t-test).
The artificial regulator AZFP-U5 (Table S3) is composed of C2H2 type zinc finger and Gal4 effector domain. The zinc finger sequence of AZFP-U5 is VSSR-RDER-ISNR-QNTQ, and the potential binding site is GYDGHGGAHATA (where Y represents C/T, D represents G/A/T, and H represents C/T/A) (Park et al., 2003). After querying the corresponding binding sites in the Rut-C30 genome, 47 from 106 potential binding sites were identified to be located within 1,500 bp upstream of the coding region of specific genes. These 47 genes might be direct targets of the artificial regulator AZFP-U5, of which the profiles of transcripts and potential functions were provided in Additional file 1. These genes are related to carbohydrate and nucleic acid metabolism, transcriptional regulatory signaling pathways (transcription factors, protein kinases), protein folding and secretion, putative extracellular proteins, 13 of these 47 potential genes are classified as proteins with unknown functions. Since the transcription of major cellulase and hemicellulase genes in T. reesei U5 has increased significantly, it is speculated that proteins related to signal transduction and transcription regulation play a key role for AZFP-U5's regulatory effects. The putative sugar transporter gene (TrC30_85306), putative histidine kinase (TrC30_129764), and putative protein kinase (TrC30_93459) may participate in the cellulase induction and gene expression. The effect of TrC30_129764 on cellulase production was further tested together with the other regulators, which were described in the section Exploration of New Regulators for T. reesei Cellulase Production.
Comparative Transcriptome Analysis of T. reesei U5 and Rut-C30
The total transcriptome reads of T. reesei U5 and C30 at 24 h were 48,803,620 and 49,017,490, respectively. At 48 h, the reads were 48,988,334 and 48,998,278, respectively, which have met the coverage assessment for the randomness and sequencing saturation assessment. Differentially expressed genes (DEGs) showed that there were 2,203 up-regulated genes and 786 down-regulated genes at 24 h, and 1,500 up-regulated genes and 383 down-regulated genes were observed at 48 h.
In addition to the five glycoside hydrolase genes, five down-regulated genes were randomly selected for RT-qPCR to verify the reliability of the transcriptome data (Figure S2). Similar trends of difference were observed by the RT-qPCR analysis and the transcriptome analysis. Therefore, the transcriptome data of T. reesei U5 and C30 can be considered reliable for further analysis.
Gene Ontology (GO) and Functional Category of DEGs
In the course of transcriptome analysis, some functional DEGs exceed 40% of the total corresponding functionally classified genes in several GO terms at 24 h, including biological regulation, cell process regulation, stress response, antioxidant activity, transport activity, and transcriptional regulation (Figure 4A). At 48 h, the functional DEGs accounting for over 15% of the overall functional classification genes are located in the following categories, localization function, stress response, membrane fraction, transport activity, and transcription factors (Figure 4B). GO analysis at two time points indicated that the transcriptional changes of functional DEGs, such as stress response, transporter and transcription factor proteins, were relatively significant.
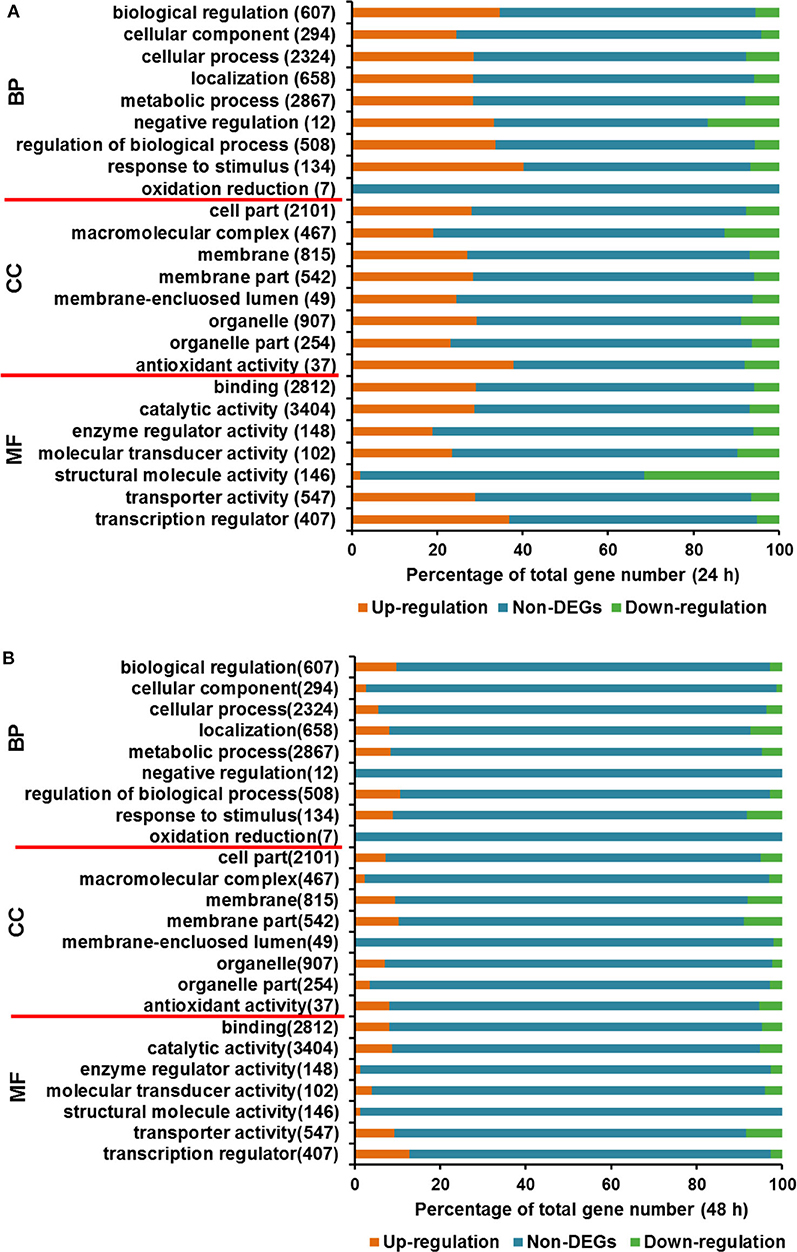
Figure 4. Gene Ontology analysis for DEGs between T. reesei U5 and Rut-C30. (A) Gene Ontology analysis at 24 h. (B) Gene Ontology analysis at 48 h. BP, biological process; CC, cellular component; MF, molecular function.
Besides the GO analysis, functional category of DEGs between T. reesei U5 and Rut-C30 was performed to evaluate statistically significant enrichment of gene functions (Figure 5). A number of DEGs were associated with metabolic functions, which account for 20.84 and 18.95% of total DEGs at 24 and 48 h, respectively. More importantly, the functional groups of DEGs included in protein fate (protein translation, folding, modification, sorting, and secretion), RNA processing (synthesis, modification, surveillance, and degradation), and transcription and signal transduction, take up 12.41%, 6.09%, and 10.17% of the total DEGs at 24 h, and 7.66%, 4.11%, and 7.90% at 48 h, respectively (Table S4, Additional file 2). The functional classification of DEGs demonstrates that RNA and protein processing kept active in T. reesei U5, especially at 24 h, which were analyzed in detail below.
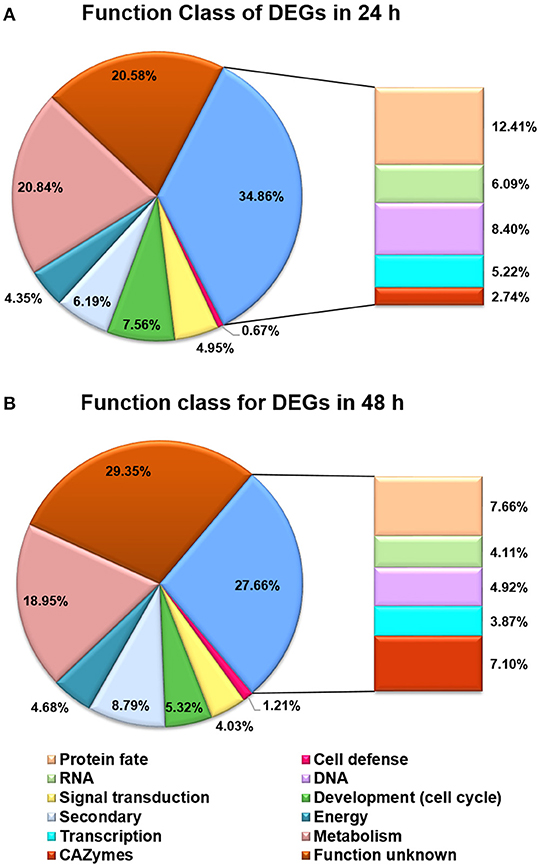
Figure 5. Functional categories for DEGs between T. reesei U5 and Rut-C30. (A) Functional categories for DEGs at 24 h. (B) Functional categories for DEGs at 48 h. Protein fate (protein translation, folding, modification, sorting, and secretion); RNA (RNA synthesis, processing, modification, surveillance and degradation); DNA (DNA replication, precursor, repairs).
Analysis of Genes Encoding Transcription Factors, GHs and Major Transporters
Regulators that potentially play crucial roles in the induction and production of cellulase in T. reesei were analyzed. Totally 420 genes in the T. reesei genome were predicted to encode transcriptional regulators, from which the transcripts of 223 genes (195 up-regulated; 38 down-regulated) and 91 genes (68 up-regulated; 23 down-regulated) changed significantly at 24 and 48 h, respectively (Additional file 3). Although the transcription levels of main cellulases improved remarkably in T. reesei U5 during the course of analysis, the known major transcription factor genes, such as xyr1, ace2, ace3, and vib1, have not changed consistently at 24 h, whose corresponding transcripts increased to a certain extent at 48 h in T. reesei U5 (Figure 6A). At 48 h, the transcription of xyr1 gene in T. reesei U5 improved more than 2-folds compared to that in Rut-C30. Meanwhile, the transcript level of the positive regulatory gene vib-1 in T. reesei U5 reached 4-fold compared to that in Rut-C30 at 48 h. The Clr-2 homologous protein is the important glycoside hydrolase positive regulator in Penicillium oxalicum and Neurospora crassa (Coradetti et al., 2012; Li et al., 2015), whose transcript levels in T. reesei U5 were 3 and 2-folds to that of Rut-C30 at 24 and 48 h, respectively. Protein functions of several differentially expressed transcription factors have been identified, among which overexpression of the gene TrC30_81999 has a positive regulatory effect on cellulase production of T. reesei (Kubicek, 2013), and the gene TrC30_101064 encoding the transcription factor Xpp1, has a feedback inhibitory effect on xylanase induction under high-concentration xylose induction conditions (Derntl et al., 2015). The transcripts of TrC30_74374 and TrC30_96554 related to light-mediated regulation in T. reesei U5 were up-regulated 16-fold and down-regulated 8-fold at 24 h (Tisch and Schmoll, 2013). The results above provide a basis for further exploring novel regulators for cellulase synthesis and regulation. Interestingly, the transcripts of repressor genes for cellulase production, ctf1 (TrC30_10530) (Meng et al., 2020) and rce1 (TrC30_6520) (Cao et al., 2017), always improved about 1-2-fold in T. reesei U5 in the time course compared to that in Rut-C30. The velvet family proteins Ve1 and Vel2 possess positive effect on cellulase, however, the transcript levels of ve1 (TrC30_98965), vel2 (TrC30_102739) in T. reesei U5 were similar as that in Rut-C30. Genes gcn5 (TrC30_82854) and lae1 (TrC30_9778) encoding histone acetyltransferase and methyltransferase, respectively, whose functions were verified to be relevant to cellulase regulation (Seiboth et al., 2012; Xin et al., 2013; Liu et al., 2016), also showed no significant changes.
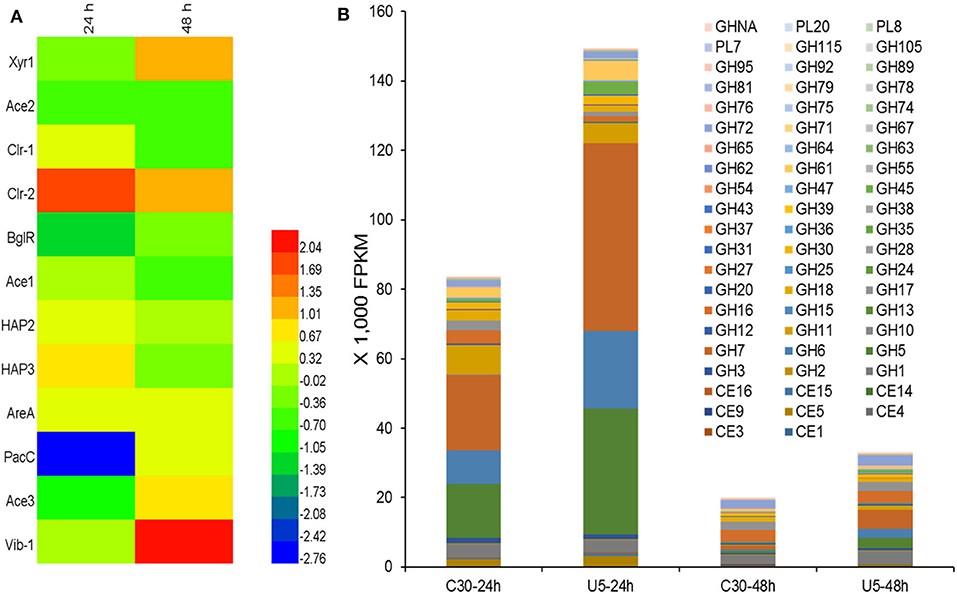
Figure 6. Differential transcription of genes encoding potential transcription regulators (A) and CAZymes (B). CE, Carbohydrate esterase; GH, Glycoside hydrolase; GHNA, Glycoside hydrolase in uncategorized family; PL, Polysaccharide lyase.
The main transcription factors Xyr1 and Cre1 proteins could affect transporter family proteins in T. reesei, especially major facilitator superfamily (MFS) (dos Santos Castro et al., 2014, 2016), of which several sugar transporters transmit extracellular carbon-induced signals or transport oligosaccharide inducer into cell, such as the sugar transporters Cdt1 and Cdt2 in N. crassa (Znameroski et al., 2013), CltB in Aspergillus nidulans (dos Reis et al., 2016), and Crt1 (Zhang et al., 2013) in T. reesei. About 448 genes in the T. reesei genome are predicted to have transporter function (Chaudhary et al., 2016), among which 51 transporter genes changed significantly (|Log2FC|>1, p < 0.05). However, the transcription of crt1 (TrC30_109243) kept the same level between T. reesei U5 and T. reesei Rut-C30. Besides ATP-binding cassette transporter superfamily genes, the transcripts of several predicted sugar transporters also showed the remarkable changes, in which the transcriptional levels of TrC30_7623, TrC30_95062, TrC30_97401, and TrC30_12083 gene were up-regulated at least 2-fold, whereas transcripts of TrC30_38765, TrC30_136619, TrC30_124396, and TrC30_133544 decreased at least 1-fold in comparison to that of Rut-C30. The sugar transporters above may play crucial roles on inducer transportation or transmission of carbon signals in T. reesei U5, which may re-modulate the profile of cellulase induction and production.
Transcription Analysis of Extracellular Protein Processing
As shown in Figure 6B, the transcript quantities of the major cellulases belonging to GH5, GH6, and GH7 families accounted for 56.2% of the total transcription of glycoside hydrolases (82,000 FPKM) in cellulase Rut-C30 at 24 h. However, the corresponding ratio is up to 75.5% for cellulase U5, whose total transcripts is 148,000 FPKM. The total transcripts of extracellular glycoside hydrolases, especially, major induced cellulases genes, such as genes cbh1, cbh2, eg1, and eg2, decreased significantly at 48 h compared to that at 24 h in two Trichoderma strains, probably due to repression under secretion stress (RESS) (Pakula et al., 2003). At 48 h, the transcription of extracellular major glycoside hydrolase genes in the two strains decreased significantly compared to that at 24 h, and the total FPKM values of extracellular protein U5 and Rut-C30 at 48 h decreased to 22–25% of that at 24 h, meanwhile, the transcripts of genes encoding the major cellulases from GH5, GH6, and GH7 families occupied 11.2% of the total cellulase transcripts in cellulase Rut-C30, 33.6% in cellulase U5 (Additional file 4). Furthermore, the transcripts of three major proteases genes (TrC30_111063, TrC30_122545, and TrC30_26076) in T. reesei U5 kept the same level to that of Rut-C30, which may exert negative effect on the stability of extracellular glycoside hydrolases (Qian et al., 2019).
Genes related to protein processing, folding, transporting, and secretion exhibited significant changes in T. reesei U5 compared to that of Rut-C30. At 24 h, the transcription levels of 14 aminoacyl-tRNA synthetase genes in T. reesei U5 were significantly higher than that in Rut-C30, whose transcript levels increased by 2 to 5.6 times. The transcriptional levels of genes encoding endoplasmic reticulum-related proteins responsible for protein processing and folding were also changed in T. reesei U5, over 40% endoplasmic reticulum protein, transporting and sorting proteins were significantly up-regulated in T. reesei U5, which may be beneficial to protein secretion (Additional file 5). The transcription levels of genes related to N-glycosylation modification in the endoplasmic reticulum were significantly increased (Ruddock and Molinari, 2006). In addition, the transcription levels of genes such as mannosyl oligosaccharide glucosidase (ERManI homolog), α-1, 3 glucanase (GlcII homolog), and protein oligosaccharyltransferase (UGGT homolog) increased by 2–3.5 times, which all are the key glycosylation enzymes and hydrolases in the endoplasmic reticulum for protein processing. Furthermore, the EDEM homolog gene encoding the marker protein of the endoplasmic reticulum associated degradation pathway, was significantly up-regulated (8-fold) compared to that in Rut-C30 (Wang and Hebert, 2003). The transcription of the eukaryotic translation initiation factor 2α kinase (PERK homolog) gene was improved 1.3-fold, which is a characteristic enzyme for endoplasmic reticulum stress response (Harding et al., 1999). Results above indicate the enhanced effect of endoplasmic reticulum-associated degradation (ERAD) on protein processing for glycoside hydrolases in T. reesei U5 at 24 h. However, at 24 h, the transcription levels of the genes encoding disulphide isomerase Pdi and ER-resident transmembrane kinase Ire1 proteins were not up-regulated, the transcript levels of hac1 gene encoding regulator for UPR, and bip1 gene encoding the luminal binding protein, an ER-localized member of the HSP70 family, also kept in similar level between T. reesei U5 and Rut-C30 at 24 h, which suggests that the unfolded protein response (UPR) was not changed in T. reesei U5. The positive function of genes above for protein processing in endoplasmic reticulum in T. reesei have been confirmed before (Saloheimo et al., 2003; Gao et al., 2018).
The ERAD and misfolding protein degradation pathway are always coupled. At 24 h, transcription quantity of the ubiquitination-proteasome system-related genes in T. reesei U5 was significantly up-regulated, which is important to reduce the pressure on the endoplasmic reticulum owing to the accumulation of post-translated glycoside hydrolases. The transcription of genes encoding Derlin and Ubx family proteins involved in the ERAD pathway was increased in T. reesei U5, facilitating the entry of misfolding protein into the proteasome. In the course of protein ubiquitination, genes coding the ubiquitin-activating enzyme E1 family, the ubiquitin-binding enzyme E2 family, and the ubiquitin ligase E3 family in T.reesei U5 were all increased to various degrees, among which genes encoding ubiquitin-activating enzyme UBE1, UBLE1A, UBLE1B, and UBE1C were up-regulated by over 3-fold, and the ubiquitin-binding enzyme UBE2Q gene was up-regulated by 6-fold. Correspondingly, transcription levels of the proteasome subunit genes in T. reesei U5 also improved to various degrees, at least twice as high as that in Rut-C30 at both time points. Transcripts of genes in the ERAD pathway, as well as ubiquitination and proteasome-related genes increased remarkably, indicating that the protein processing of T. reesei U5 has begun speedily at 24 h, which resulted in a large increase in extracellular cellulase protein secretion in T. reesei U5 compared to that in T. reesei Rut-C30.
In addition, there are several significantly different transcriptional levels in metabolic pathways or cellular physiological activities between T. reesei U5 and Rut-C30 at 24 h, and the transcript level of genes in the following groups always improved remarkably in T. reesei U5: RNA polymerase II subunit and its basic transcriptional regulatory factors, mRNA regulatory pathways, RNA degradation, peroxisomes, CoA synthesis pathways, N-glycoside synthesis pathways, ammonia Acyl-tRNA synthesis pathway, nicotinamide metabolism pathway, fatty acid metabolism pathway, endoplasmic reticulum membrane protein processing, and proteasome complex (Additional file 2).
Exploration of New Regulators for T. reesei Cellulase Production
In the transcriptome data analysis of T. reesei U5, putative regulatory genes changing significantly at both 24 and 48 h are likely to be targets for activating the transcription of cellulase genes. Therefore, 15 genes possessing predicted regulatory function were selected as targets for further analysis. The predicted protein-encoding genes screened are shown in Table 1, which mainly include the fungal Zn2Cys6 type binuclear cluster transcription factor, histone kinase, LysR family regulatory proteins, helix-transition-helix regulatory proteins, and NmrA family regulatory proteins.
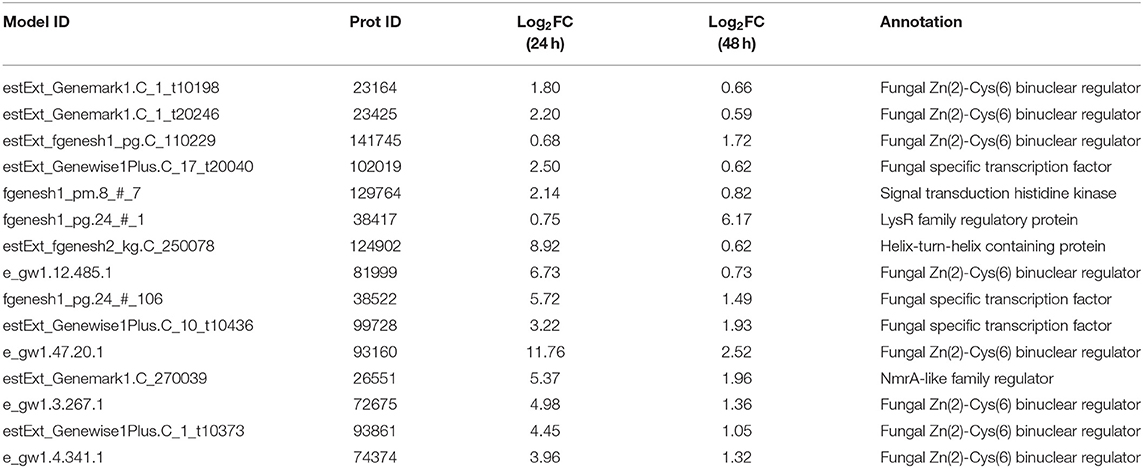
Table 1. Predicting differentially expressed genes with regulatory functions by overexpression of AZFP-U5.
Recombinant strains with overexpression of the 15 putative regulators were obtained. With the exception of T. reesei Tr93861 strain overexpressing gene TrC30_93861, whose spore-forming ability was reduced significantly and mycelium growth was impaired, the other strains did not have significant changes in growth and mycelial morphology compared to T. reesei Rut-C30. Notably, the TrC30_93861 protein homolog in the wild-type strain T. reesei QM6a has been identified as the transcription factor Ypr1 (Derntl et al., 2016), so TrC30_93861 gene is named as ypr1 in the following text. Through the fermentation performance of the transformants overexpressing 15 putative regulatory genes, it can be deduced that these regulators, when individually overexpressed, did not exert any positive regulation on cellulase production, and even the overall enzyme activities from some certain transformants showed a decreased level compared to Rut-C30, as shown in Figure 7. Gene TrC30_129764 is the potential target gene regulated by AZFP-U5 directly, whose transcript also improved significantly in the T. reesei U5. However, TrC30_129764 overexpressed strain showed similar cellulase activity profile with that in Rut-C30, which suggest that TrC30_129764 is not a crucial factor for cellulase regulation. Furthermore, the transcript level of gene TrC30_38522 encoding a putative transcriptional regulator in T. reesei U5 improved by over 50 and 2-fold at 24 and 48 h compared to that in Rut-C30, whose expressional level also elevated several folds when T. reesei Rut-C30 was cultured into the sugarcane bagasse medium (Borin et al., 2018). However, no difference was observed for cellulase production when TrC30_38522 was overexpressed in T. reesei Rut-C30. Among the transformants, the strains overexpressing genes ypr1 and TrC30_74374 showed 38.8% and 42.7% higher FPase activities than that of Rut-C30, respectively. Meanwhile, the corresponding extracellular protein secretion increased by 29.44% and 36.54%, respectively.
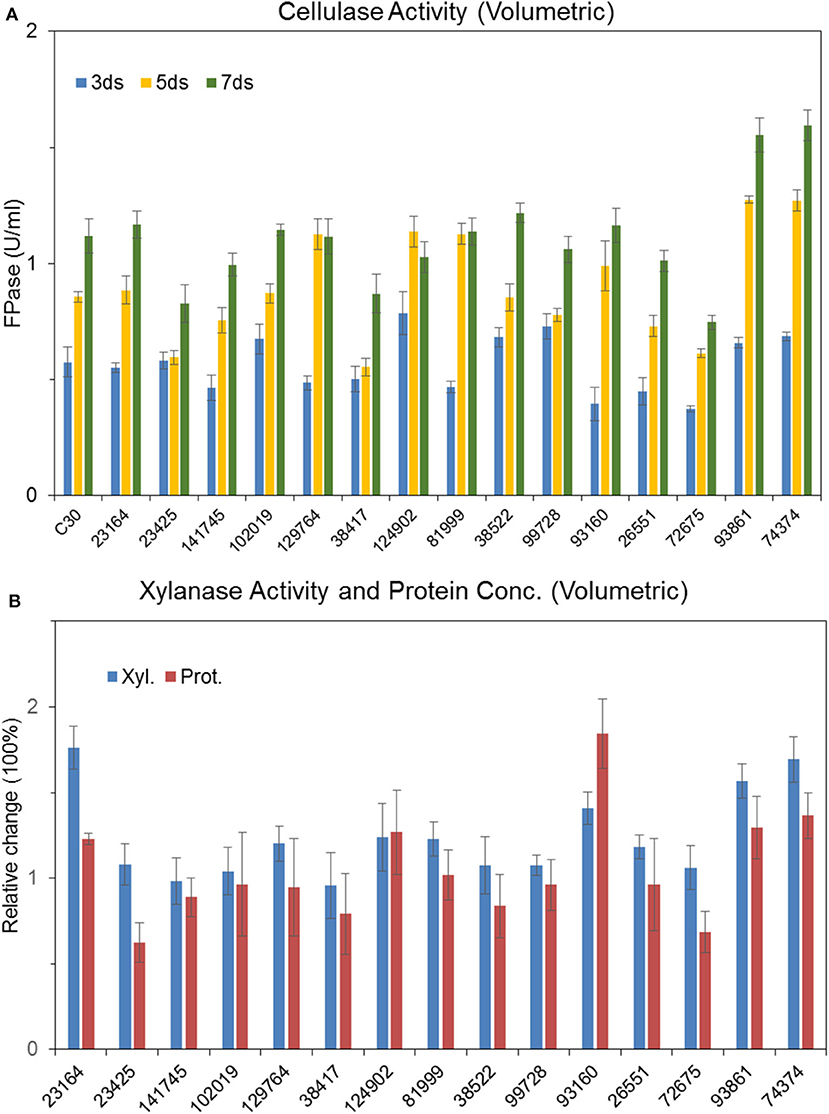
Figure 7. Effects of overexpression of 15 transcription factors on cellulase production Cellulase (A), xylanase and protein productions (B) by T. reesei Rut-C30 recombinant strains overexpressing the 15 candidate transcription factors.
Possible Crosslink Between Cellulase Production and Pigment Secretion
In order to further study the functions of ypr1 or TrC30_74374 in T. reesei Rut-C30, ypr1, and TrC30_74374 gene knockout strains Δypr1 and Δ74374 were constructed and verified individually with the correct integration as a single copy (Figure S4). Unexpectedly, no change in cellulase activities were observed in strains Δypr1 and Δ74374 as to that in the control strain T. reesei Rut-C30 (Figure S5), suggesting that these two regulators are not essential for regulation of cellulase biosynthesis under the conditions used in this work.
In terms of cell morphology, the phenotype of T. reesei Δ74374 had no obvious change compared with the parental strain, and the fermentation broth was pale yellow under the condition of cellulose medium. T. reesei Δypr1 recovered the similar level of spore-forming ability to the parental strain, so did the growth biomass and mycelial morphology. During the cellulase fermentation process, the Δypr1 strain no longer secreted yellow pigment, and the fermentation broth became white in contrast to Rut-C30. However, the yellow pigment production in the ypr1 overexpression strain Tr93861 enhanced significantly. Meanwhile, conidiation of Tr93861 strain decreased remarkably (Figure 8). Hypersecretion of the yellow pigment may be the direct cause of significant reduction in vegetative growth owing to the metabolic burden or the growth limiting effect of yellow pigment (Derntl et al., 2017a). The Ypr1 homolog in Rut-C30 (663 aa) have four regions of amino acid residues (insertions and deletions) in the C-terminal part of middle homology region (MHR) and regulatory region compared that of Ypr1 in QM6a (gene ID: 102499, 686 aa), which maybe resulted in the different regulatory profile for cellulase production and pigment secretion between Rut-C30 and QM6a (Figure S6).
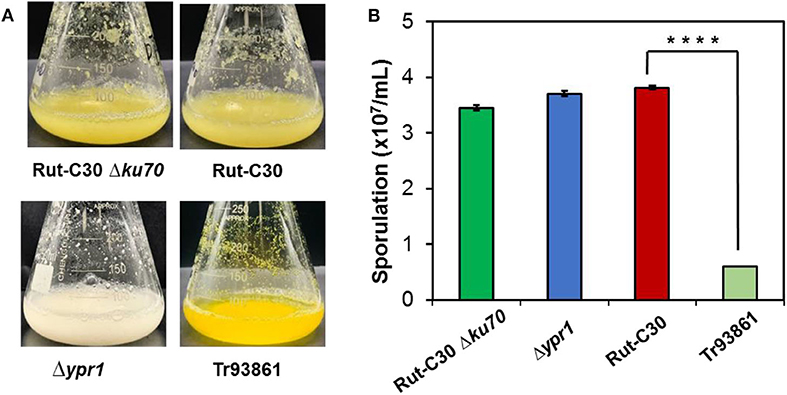
Figure 8. Effects of ypr1 on pigment production and sporulation. T. reesei Tr93861 is the mutant strain overexpressing ypr1 in T. reesei Rut-C30, whereas strain Δypr1 was obtained by deleting ypr1 in T. reesei Δku70. The mutant strains and their respective parental strains were cultured on cellulase production medium for 5 days, and pigment production was shown (A). The sporulation assay of the T. reesei strains was implemented when cultured on MEA for 7 days (B). Asterisks indicate significant differences from reference strains (****p < 0.001, Student's t-test).
To further analyze the effect of ypr1 knockout on the secretion of T. reesei cellulase, comparative transcriptome analysis was performed for the knockout mutant T. reesei Δypr1 and the parental strain Rut-C30 Δku70 in MA medium with glucose as carbon source. Unexpectedly, we found that the transcription of the glycoside hydrolase genes in T. reesei Δypr1 mutant were significantly changed compared to the parental strain T. reesei Rut-C30 Δku70. Table 2 lists transcription changes in several major glycoside hydrolases and transcriptional regulator genes. The transcriptional quantity of major cellulases has been improved remarkably in T. reesei Δypr1, the minimum change fold is 1-fold increased (cbh1 gene), and the maximum transcript level change happened to the eg1 gene which increased by 3.8 times. The transcriptional levels for most hemicellulase genes are improved by at least 1.35-fold. Meanwhile, the transcriptions of cel61a, cip1 genes encoding the auxiliary proteins, and axe1 gene (encoding acetylxylan esterase) have increased by 0.8, 1.6 and 2.4-fold, respectively, whose positions are located in close proximity to the gene ypr1 locus (scaffold_1:507183-509547), which means that the deletion of Ypr1 protein facilitates the transcription of the surrounding glycoside hydrolase genes. Meanwhile, the transcripts of genes encoding the major cellulase regulatory factors, such as the genes of Xyr1 and Ace3, have increased significantly, except for Vib1.
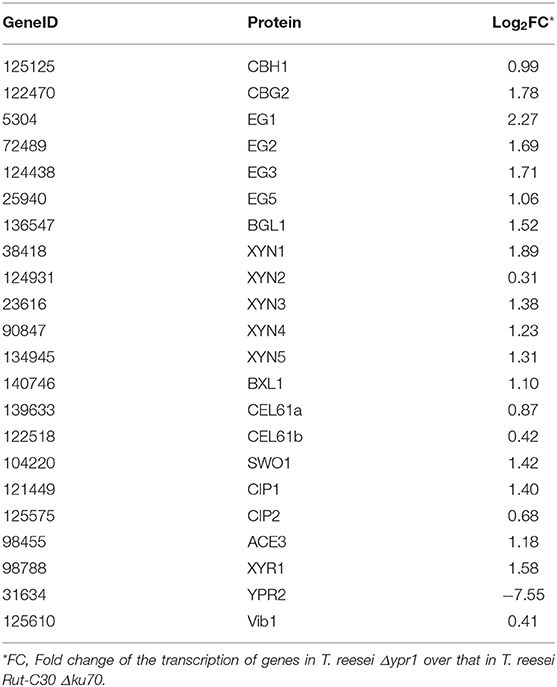
Table 2. Changes of major glycoside hydrolases and transcription factors in T. reesei Δypr1 comparing with that of the control strain.
Discussion
In our previous work, T. reesei strains overexpressing AZFP-U3 (Zhang et al., 2016) and AZFP-M2 (Meng et al., 2020) showed enhanced cellulase production. Comparing with these two AZFP genes, AZFP-U5 has different sequence and also showed different regulatory patterns on cellulase biosynthesis. The strain overexpressing AZFP-U5 showed the best phenotypes among the three AZFP strains, with higher protein secretion level and higher activities of various cellulases and xylanases. Therefore, it is of great interest to further explore the mechanisms by which this AZFP functions.
In the previous studies, transcriptome analysis was performed using T. reesei grown in different carbon source (glucose, lactose, sophorose, or cellulose) and lignocellulose substrates, and data analysis has been focused on the functions of known major regulators, such as Xyr1 and Cre1 (dos Santos Castro et al., 2014, 2016; Hakkinen et al., 2014). Nevertheless, detailed analysis of global transcription as well as metabolic pathways for cellulase production in T. reesei is still lacking. In this work, we present extensive and comprehensive analysis of global transcription network reprogramming by the artificial regulator AZFP-U5, and assume that multiple pathways may be involved in cellulase biosynthesis by the function of AZFP-U5. For example, AZFP-U5 activates the transcription of glycoside hydrolase genes in the mRNA regulatory pathway, and also leads to changes of a series of metabolic processes, including mRNA synthesis, processing, and degradation, which may then lead to an increase in protein translation, processing, and secretion. Meanwhile, activation of the protein degradation pathway may be achieved by accumulation of unfolded proteins. The improvement of tRNA synthetase genes in the aminoacyl-tRNA synthesis pathway further indicated that the protein synthesis in T. reesei U5 were more active compared to that in T. reesei Rut-C30. In the reprogramming of global gene transcription, T. reesei U5 strain may be supplied with more energy, reducing power, and protein synthesis precursors by the activated intercellular metabolic pathways, which was also reflected from the comparative transcriptome results. After investigating dynamic changes of global transcription, we found different transcription profiles at 24 and 48 h. At 48 h, genes encoding marker proteins in the endoplasmic reticulum still have an up-regulation in T. reesei U5, indicating that AZPF-U5 may also have stimulating effects on protein processing, traffickling, and secretion pathway.
In addition to changes in the key metabolic processes, variation of transcription in several sugar transporters were discovered. AZFP-U5 may activate transcription of major transporter genes, which could transport potential carbon source inducers or transmit the carbon signal for induction, followed by transcriptional activation of the glycoside hydrolase genes regulated by AZFP-U5, directly, or indirectly. Meanwhile, the AZFP-U5 activates the stress response pathway and the transcription of the peroxisome protein genes, indicating that this artificial regulator has a potential positive effect on the release of reactive oxygen and external environmental stress response in protein and peptide synthesis. The possible mechanism by which AZFP-U5 works on cellulase production is shown in Figure 9. Further studies are needed to explore the directly regulated genes by AZFP-U5 as well as key genes on regulation of glycoside hydrolase genes.
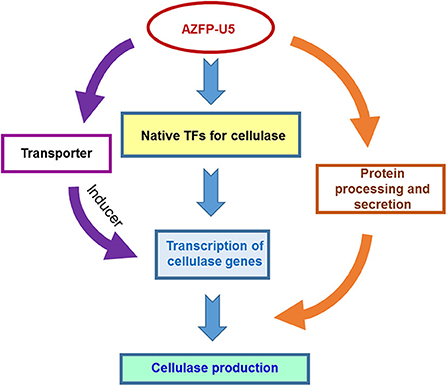
Figure 9. Proposed model for the regulatory effect of AZF-U5 on cellulase synthesis. TFs transcription factors.
During the transcriptome analysis, many putative regulator genes showed enhanced transcription by AZFP-U5 overexpression, of which 15 were selected for further investigation. However, the results did not show significant increase in cellulase production by overexpressing these regulators. It may be possible that individual expression of these regulators may not be sufficient to enhance cellulase biosynthesis, and the advantage of AZFP-U5 is that it simultaneously regulates multiple gene transcription by binding with various target genes, and induce changes in related signal transduction and metabolic pathways. It will be interesting to test the combinatory effects of the regulators for regulating cellulase production in the future work.
In this study, we found that Ypr1 in T. reesei Rut-C30 not only regulates the formation of secondary metabolites, but also participates in the transcriptional regulation of glycoside hydrolase genes when glucose was used as a carbon source. From transcriptome analysis, deletion of Ypr1 significantly increased the transcription of extracellular glycoside hydrolases and major transcription factor genes. We speculate that the secondary metabolites have a coupling effect with the cellulase production. This crosslink is also reflected in the transcription factor Xpp1 in T. reesei, the presence of Xpp1 can inhibit the synthesis of hemicellulase genes and inhibit the production of secondary metabolites in the cell, which acts as a switch function between the primary and secondary metabolism (Derntl et al., 2017b). Moreover, T. reesei ZC121 with up-regulated Ypr1 could overproduce the yellow pigment, but almost produce no extracellular cellulase (Li et al., 2018).
Ypr2 is a transcription factor that affects the production of yellow pigment, which has an inhibition effect on Ypr1 expression (Derntl et al., 2016). Deletion of Ypr1 also severely affected the transcription of Ypr2 (almost no ypr2 transcript in T. reesei Δypr1 strain). Moreover, under dark conditions, Ypr2 (gene ID: 102497 in T. reesei QM6a, 664 aa) can affect the transcription of genes regulated by Cre1 in T. reesei QM6a, and Ypr2 also could regulate Ypr1 (Hitzenhammer et al., 2019). However, the Ypr2 protein in T. reesei Rut-C30 (gene ID: TrC30_31634, 511 aa) contains a zinc finger-free DNA binding domain in T. reesei Rut-C30 (the first 175 amino acid residues missed), which will cause severe impact on DNA binding and regulatory function. Therefore, the specific role and function of Ypr2 lacking the binding domain in metabolism and pigment secretion requires further analysis (Figure S7).
The results in this study showed possible cross talk between cellulase production and pigment secretion in T. reesei regulated by the major regulator Ypr1 protein. Meanwhile, deletion of Ypr1 significantly increase the transcription of major glycoside hydrolase and transcription factor genes. Actually, CAZyme genes in T. reesei are non-randomly distributed within the genome, about (41%) CAZyme genes are found in 25 discrete regions containing specific regulators or secondary metabolites clusters (Martinez et al., 2008; Hakkinen et al., 2014). Ypr1 not only locates into nearly adjacent region of glycoside hydrolases, but also possess the co-regulated effect for TrC30_69551 and some glycoside hydrolase genes, including axe1, cip1, and cel61a. However, there is no significant change in Δypr1 for extracellular cellulase secretion compared to that from parental strain when cellulose or glucose is used as the substrate. In T. reesei Δypr1, the transcript level of ypr2 nearly disappeared (Log2FC = −7.5), which may intensify CCR effect on cellulase translation and secretion. Another reason may be the existence of complex post-transcriptional or post-translational mechanisms for cellulase proteins, which need to be revealed in further studies.
Conclusion
T. reesei mutant U5 bearing an AZFP (AZFP-U5) was focused, which secretes more proteins and showed significantly higher cellulase and xylanase production than its parental strain T. reesei Rut-C30. Comparative transcriptome analysis showed enhanced transcription and post-translational modifications of glycoside hydrolases by overexpression of AZFP-U5. Furthermore, deletion of ypr1 and TrC30_74374 did not affect cellulase production. Deletion of ypr1 affected cellulase gene transcription, suggesting possible crosslink between pigment production and cellulase gene expression by the AZFP-U5-associated regulator Ypr1.
Data Availability Statement
The raw data supporting the conclusions of this article will be made available by the authors, without undue reservation, to any qualified researcher.
Author Contributions
FZ and X-QZ designed the experiments. FZ and J-XL performed the experiments as well as data analysis, and also prepared the draft of the manuscript. VC, C-GL, and F-WB participated in revision of the manuscript. X-QZ supervised the experiments and critically revise the manuscript. All authors contributed to the article and approved the submitted version.
Conflict of Interest
The authors declare that the research was conducted in the absence of any commercial or financial relationships that could be construed as a potential conflict of interest.
Acknowledgments
This work was financially funded by Natural Science Foundation of China with a grant reference number of 21536006, and the Open Funding Project of the State Key Laboratory of Bioreactor Engineering and the Fundamental Research Funds for the Central Universities (No. 222201714053). The authors acknowledge ToolGen Inc., South Korea for providing the plasmid containing artificial zinc finger protein genes.
Supplementary Material
The Supplementary Material for this article can be found online at: https://www.frontiersin.org/articles/10.3389/fbioe.2020.00649/full#supplementary-material
References
Abrahao Neto, J., Rossini, C., El-Gogary, S., Henrique-Silva, F., Crivellaro, O., and El-Dorry, H. (1995). Mitochondrial functions mediate cellulase gene expression in Trichoderma reesei. Biochemistry 34, 10456–10462. doi: 10.1021/bi00033a018
Aro, N., Saloheimo, A., Ilmen, M., and Penttila, M. (2001). ACEII, a novel transcriptional activator involved in regulation of cellulase and xylanase genes of Trichoderma reesei. J. Biol. Chem. 276, 24309–24314. doi: 10.1074/jbc.M003624200
Bailey, M. J., Biely, P., and Poutanen, K. (1992). Interlaboratory testing of methods for assay of xylanase activity. J. Biotechnol. 23, 257–270. doi: 10.1016/0168-1656(92)90074-J
Bischof, R. H., Ramoni, J., and Seiboth, B. (2016). Cellulases and beyond: the first 70 years of the enzyme producer Trichoderma reesei. Microb. Cell Fact. 15:106. doi: 10.1186/s12934-016-0507-6
Borin, G. P., Carazzolle, M. F., dos Santos, R. A. C., Riaño-Pachón, D. M., and Oliveira, J. V. (2018). Gene co-expression network reveals potential new genes related to sugarcane bagasse degradation in Trichoderma reesei RUT-30. Front. Bioeng. Biotechnol. 6:151. doi: 10.3389/fbioe.2018.00151
Cao, Y. L., Zheng, F. L., Wang, L., Zhao, G. L., Chen, G. J., Zhang, W. X., et al. (2017). Rce1, a novel transcriptional repressor, regulates cellulase gene expression by antagonizing the transactivator Xyr1 in Trichoderma reesei. Mol. Microbiol. 105, 65–83. doi: 10.1111/mmi.13685
Chaudhary, N., Kumari, I., Sandhu, P., Ahmed, M., and Akhter, Y. (2016). Proteome scale census of major facilitator superfamily transporters in Trichoderma reesei using protein sequence and structure based classification enhanced ranking. Gene 585, 166–176. doi: 10.1016/j.gene.2016.03.043
Chen, L., Zou, G., Wang, J. Z., Wang, J., Liu, R., Jiang, Y., et al. (2016). Characterization of the Ca2+-responsive signaling pathway in regulating the expression and secretion of cellulases in Trichoderma reesei Rut-C30. Mol. Microbiol. 100, 560–575. doi: 10.1111/mmi.13334
Coradetti, S. T., Craig, J. P., Xiong, Y., Shock, T., Tian, C., and Glass, N. L. (2012). Conserved and essential transcription factors for cellulase gene expression in ascomycete fungi. Proc. Natl. Acad. Sci. U.S.A. 109, 7397–7402. doi: 10.1073/pnas.1200785109
Derntl, C., Guzmán-Chávez, F., Mello-de-Sousa, T. M., Busse, H.-J., Driessen, A. J., Mach, R. L., et al. (2017a). In vivo study of the sorbicillinoid gene cluster in Trichoderma reesei. Front. Microbiol. 8:2037. doi: 10.3389/fmicb.2017.02037
Derntl, C., Kluger, B., Bueschl, C., Schuhmacher, R., Mach, R. L., and Mach-Aigner, A. R. (2017b). Transcription factor Xpp1 is a switch between primary and secondary fungal metabolism. Proc. Natl. Acad. Sci. U.S.A. 114, E560–E569. doi: 10.1073/pnas.1609348114
Derntl, C., Mach, R. L., and Mach-Aigner, A. R. (2019). Fusion transcription factors for strong, constitutive expression of cellulases and xylanases in Trichoderma reesei. Biotechnol. Biofuels 12:231. doi: 10.1186/s13068-019-1575-8
Derntl, C., Rassinger, A., Srebotnik, E., Mach, R. L., and Mach-Aigner, A. R. (2015). Xpp1 regulates the expression of xylanases, but not of cellulases in Trichoderma reesei. Biotechnol. Biofuels 8:112. doi: 10.1186/s13068-015-0298-8
Derntl, C., Rassinger, A., Srebotnik, E., Mach, R. L., and Mach-Aigner, A. R. (2016). Identification of the main regulator responsible for synthesis of the typical yellow pigment produced by Trichoderma reesei. Appl. Environ. Microbiol. 82, 6247–6257. doi: 10.1128/AEM.01408-16
dos Reis, T. F., de Lima, P. B., Parachin, N. S., Mingossi, F. B., de Castro Oliveira, J. V., Ries, L. N., et al. (2016). Identification and characterization of putative xylose and cellobiose transporters in Aspergillus nidulans. Biotechnol. Biofuels 9:204. doi: 10.1186/s13068-016-0611-1
dos Santos Castro, L., de Paula, R. G., Antoniêto, A. C., Persinoti, G. F., Silva-Rocha, R., and Silva, R. N. (2016). Understanding the role of the master regulator XYR1 in Trichoderma reesei by global transcriptional analysis. Front. Microbiol. 7:175. doi: 10.3389/fmicb.2016.00175
dos Santos Castro, L., Pedersoli, W. R., Antoniêto, A. C. C., Steindorff, A. S., Silva-Rocha, R., Martinez-Rossi, N. M., et al. (2014). Comparative metabolism of cellulose, sophorose and glucose in Trichoderma reesei using high-throughput genomic and proteomic analyses. Biotechnol. Biofuels 7:41. doi: 10.1186/1754-6834-7-41
Druzhinina, I. S., and Kubicek, C. P. (2017). Genetic engineering of Trichoderma reesei cellulases and their production. Microb. Biotechnol. 10, 1485–1499. doi: 10.1111/1751-7915.12726
Gao, F., Hao, Z. Z., Sun, X. H., Qin, L., Zhao, T., Liu, W. Q., et al. (2018). A versatile system for fast screening and isolation of Trichoderma reesei cellulase hyperproducers based on DsRed and fluorescence-assisted cell sorting. Biotechnol. Biofuels 11:261. doi: 10.1186/s13068-018-1264-z
Grigoriev, I. V., Nikitin, R., Haridas, S., Kuo, A., Ohm, R., Otillar, R., et al. (2014). MycoCosm portal: gearing up for 1000 fungal genomes. Nucleic Acids Res. 42, D699–704. doi: 10.1093/nar/gkt1183
Hakkinen, M., Arvas, M., Oja, M., Aro, N., Penttila, M., Saloheimo, M., et al. (2012). Re-annotation of the CAZy genes of Trichoderma reesei and transcription in the presence of lignocellulosic substrates. Microb. Cell Fact. 11:134. doi: 10.1186/1475-2859-11-134
Hakkinen, M., Valkonen, M. J., Westerholm-Parvinen, A., Aro, N., Arvas, M., Vitikainen, M., et al. (2014). Screening of candidate regulators for cellulase and hemicellulase production in Trichoderma reesei and identification of a factor essential for cellulase production. Biotechnol. Biofuels 7:14. doi: 10.1186/1754-6834-7-14
Harding, H. P., Zhang, Y., and Ron, D. (1999). Protein translation and folding are coupled by an endoplasmic-reticulum-resident kinase. Nature 397:271. doi: 10.1038/16729
He, R. L., Ma, L. J., Li, C., Jia, W. D., Li, D. M., Zhang, D. Y., et al. (2014). Trpac1, a pH response transcription regulator, is involved in cellulase gene expression in Trichoderma reesei. Enzyme Microb. Technol. 67, 17–26. doi: 10.1016/j.enzmictec.2014.08.013
Hitzenhammer, E., Büschl, C., Sulyok, M., Schuhmacher, R., Kluger, B., Wischnitzki, E., et al. (2019). YPR2 is a regulator of light modulated carbon and secondary metabolism in Trichoderma reesei. BMC Genomics 20:211. doi: 10.1186/s12864-019-5574-8
Kubicek, C. P. (2013). Systems biological approaches towards understanding cellulase production by Trichoderma reesei. J. Biotechnol. 163, 133–142. doi: 10.1016/j.jbiotec.2012.05.020
Li, C. C., Lin, F. M., Sun, W., Yuan, S. X., Zhou, Z. H., Wu, F. G., et al. (2018). Constitutive hyperproduction of sorbicillinoids in Trichoderma reesei ZC121. Biotechnol. Biofuels 11:291. doi: 10.1186/s13068-018-1296-4
Li, Z., Yao, G., Wu, R., Gao, L., Kan, Q., Liu, M., et al. (2015). Synergistic and dose-controlled regulation of cellulase gene expression in Penicillium oxalicum. PLoS Genet. 11:e1005509. doi: 10.1371/journal.pgen.1005509
Liu, G. D., and Qu, Y. B. (2019). Engineering of filamentous fungi for efficient conversion of lignocellulose: Tools, recent advances and prospects. Biotechnol. Adv. 37, 519–529. doi: 10.1016/j.biotechadv.2018.12.004
Liu, K., Dong, Y., Wang, F., Jiang, B., Wang, M., and Fang, X. (2016). Regulation of cellulase expression, sporulation, and morphogenesis by velvet family proteins in Trichoderma reesei. Appl. Microbiol. Biotechnol. 100, 769–779. doi: 10.1007/s00253-015-7059-2
Liu, Y. G., and Chen, Y. L. (2007). High-efficiency thermal asymmetric interlaced PCR for amplification of unknown flanking sequences. Biotechniques 43, 649–556. doi: 10.2144/000112601
Mandels, M., and Andreotti, R. (1978). Problems and challenges in the cellulose to cellulase fermentation. Proc Biochem. 13, 6–13.
Martinez, D., Berka, R. M., Henrissat, B., Saloheimo, M., Arvas, M., Baker, S. E., et al. (2008). Genome sequencing and analysis of the biomass-degrading fungus Trichoderma reesei (syn. Hypocrea jecorina). Nat. Biotechnol. 26, 553–560. doi: 10.1038/nbt1403
Meng, Q. S., Zhang, F., Liu, C. G., Zhao, X. Q., and Bai, F. W. (2020). Identification of a novel repressor encoded by the putative gene ctf1 for cellulase biosynthesis in Trichoderma reesei through artificial zinc finger engineering. Biotechnol. Bioeng. 117, 1747–1760. doi: 10.1002/bit.27321
Mortazavi, A., Williams, B. A., McCue, K., Schaeffer, L., and Wold, B. (2008). Mapping and quantifying mammalian transcriptomes by RNA-Seq. Nat. Methods 5:621. doi: 10.1038/nmeth.1226
Nakari-Setala, T., Paloheimo, M., Kallio, J., Vehmaanpera, J., Penttila, M., and Saloheimo, M. (2009). Genetic modification of carbon catabolite repression in Trichoderma reesei for improved protein production. Appl. Environ. Microbiol. 75, 4853–4860. doi: 10.1128/AEM.00282-09
Nitta, M., Furukawa, T., Shida, Y., Mori, K., Kuhara, S., Morikawa, Y., et al. (2012). A new Zn(II)(2)Cys(6)-type transcription factor BglR regulates beta-glucosidase expression in Trichoderma reesei. Fungal Genet. Biol. 49, 388–397. doi: 10.1016/j.fgb.2012.02.009
Pakula, T. M., Laxell, M., Huuskonen, A., Uusitalo, J., Saloheimo, M., and Penttilä, M. (2003). The effects of drugs inhibiting protein secretion in the filamentous fungus Trichoderma reesei. J. Biol. Chem. 278, 45011–45020. doi: 10.1074/jbc.M302372200
Park, K. S., Lee, D. K., Lee, H., Lee, Y., Jang, Y. S., Kim, Y. H., et al. (2003). Phenotypic alteration of eukaryotic cells using randomized libraries of artificial transcription factors. Nat. Biotechnol. 21, 1208–1214. doi: 10.1038/nbt868
Qian, Y. C., Zhong, L. X., Sun, Y., Sun, N. N., Zhang, L., Liu, W. F., et al. (2019). Enhancement of cellulase production in Trichoderma reesei via disruption of multiple protease genes identified by comparative secretomics. Front. Microbiol. 10:2784. doi: 10.3389/fmicb.2019.02784
Ruddock, L. W., and Molinari, M. (2006). N-glycan processing in ER quality control. J. Cell Sci. 119, 4373–4380. doi: 10.1242/jcs.03225
Saloheimo, M., Valkonen, M., and Penttilä, M. (2003). Activation mechanisms of the HACI-mediated unfolded protein response in filamentous fungi. Mol. Microbiol. 47, 1149–1161. doi: 10.1046/j.1365-2958.2003.03363.x
Schmoll, M. (2018). Regulation of plant cell wall degradation by light in Trichoderma. Fungal. Biol. Biotechnol. 5:10. doi: 10.1186/s40694-018-0052-7
Seiboth, B., Karimi, R. A., Phatale, P. A., Linke, R., Hartl, L., Sauer, D. G., et al. (2012). The putative protein methyltransferase LAE1 controls cellulase gene expression in Trichoderma reesei. Mol. Microbiol. 84, 1150–1164. doi: 10.1111/j.1365-2958.2012.08083.x
Sharma, A., Tewari, R., Rana, S. S., Soni, R., and Soni, S. K. (2016). Cellulases: classification, methods of determination and industrial applications. Appl. Biochem. Biotechnol. 179, 1346–1380. doi: 10.1007/s12010-016-2070-3
Solomon, P. S., Ipcho, S. V., Hane, J. K., Tan, K.-C., and Oliver, R. P. (2008). A quantitative PCR approach to determine gene copy number. Fung. Gene. Rep. 55:5–8. doi: 10.4148/1941-4765.1082
Tisch, D., and Schmoll, M. (2013). Targets of light signalling in Trichoderma reesei. BMC Genomics 14:657. doi: 10.1186/1471-2164-14-657
Tiwari, R., Nain, L., Labrou, N. E., and Shukla, P. (2018). Bioprospecting of functional cellulases from metagenome for second generation biofuel production: a review. Crit. Rev. Microbiol. 44, 244–257. doi: 10.1080/1040841X.2017.1337713
Wang, F. Z., Zhang, R. Q., Han, L. J., Guo, W., Du, Z. Q., Niu, K. L., et al. (2019). Use of fusion transcription factors to reprogram cellulase transcription and enable efficient cellulase production in Trichoderma reesei. Biotechnol. Biofuels 12, 1–12. doi: 10.1186/s13068-019-1589-2
Wang, T., and Hebert, D. N. (2003). EDEM an ER quality control receptor. Nat. Struct. Mol. Biol. 10:319. doi: 10.1038/nsb0503-319
Xin, Q., Gong, Y. J., Lv, X. X., Chen, G. J., and Liu, W. F. (2013). Trichoderma reesei histone acetyltransferase Gcn5 regulates fungal growth, conidiation, and cellulase gene expression. Curr. Microbiol. 67, 580–589. doi: 10.1007/s00284-013-0396-4
Yang, J., Kim, B., Kim, G. Y., Jung, G. Y., and Seo, S. W. (2019). Synthetic biology for evolutionary engineering: from perturbation of genotype to acquisition of desired phenotype. Biotechnol. Biofuels 12:113. doi: 10.1186/s13068-019-1460-5
Zhang, F., Bai, F. W., and Zhao, X. Q. (2016). Enhanced cellulase production from Trichoderma reesei Rut-C30 by engineering with an artificial zinc finger protein library. Biotechnol. J. 11, 1282–1290. doi: 10.1002/biot.201600227
Zhang, F., Zhao, X. Q., and Bai, F. W. (2018a). Improvement of cellulase production in Trichoderma reesei Rut-C30 by overexpression of a novel regulatory gene Trvib-1. Bioresour. Technol. 247, 676–683. doi: 10.1016/j.biortech.2017.09.126
Zhang, J. J., Chen, Y. M., Wu, C., Liu, P., Wang, W., and Wei, D. Z. (2019). The transcription factor ACE3 controls cellulase activities and lactose metabolism via two additional regulators in the fungus Trichoderma reesei. J. Biol. Chem. 294, 18435–18450. doi: 10.1074/jbc.RA119.008497
Zhang, J. J., Zhang, G. X., Wang, W., and Wei, D. Z. (2018b). Enhanced cellulase production in Trichoderma reesei RUT C30 via constitution of minimal transcriptional activators. Microb. Cell Fact. 17:75. doi: 10.1186/s12934-018-0926-7
Zhang, W. X., Kou, Y. B., Xu, J. T., Cao, Y. L., Zhao, G. L., Shao, J., et al. (2013). Two major facilitator superfamily sugar transporters from Trichoderma reesei and their roles in induction of cellulase biosynthesis. J. Biol. Chem. 288, 32861–32872. doi: 10.1074/jbc.M113.505826
Zhang, X. Y., Li, Y. H., Zhao, X. Q., and Bai, F. W. (2017). Constitutive cellulase production from glucose using the recombinant Trichoderma reesei strain overexpressing an artificial transcription activator. Bioresour. Technol. 223, 317–322. doi: 10.1016/j.biortech.2016.10.083
Keywords: Trichoderma reesei, cellulase production, artificial zinc finger protein, comparative transcriptome analysis, transcription factor, lignocellulosic biomass
Citation: Zhang F, Li J-X, Champreda V, Liu C-G, Bai F-W and Zhao X-Q (2020) Global Reprogramming of Gene Transcription in Trichoderma reesei by Overexpressing an Artificial Transcription Factor for Improved Cellulase Production and Identification of Ypr1 as an Associated Regulator. Front. Bioeng. Biotechnol. 8:649. doi: 10.3389/fbioe.2020.00649
Received: 20 March 2020; Accepted: 27 May 2020;
Published: 03 July 2020.
Edited by:
Min Jiang, Nanjing Tech University, ChinaCopyright © 2020 Zhang, Li, Champreda, Liu, Bai and Zhao. This is an open-access article distributed under the terms of the Creative Commons Attribution License (CC BY). The use, distribution or reproduction in other forums is permitted, provided the original author(s) and the copyright owner(s) are credited and that the original publication in this journal is cited, in accordance with accepted academic practice. No use, distribution or reproduction is permitted which does not comply with these terms.
*Correspondence: Xin-Qing Zhao, eHF6aGFvJiN4MDAwNDA7c2p0dS5lZHUuY24=