- 1Postgraduate Program in Translational Biomedicine, University of Grande Rio, Duque de Caxias, Brazil
- 2Directory of Metrology Applied to Life Sciences, National Institute of Metrology, Quality and Technology, Duque de Caxias, Brazil
- 3Postgraduate Program in Biotechnology, National Institute of Metrology Quality and Technology, Duque de Caxias, Brazil
- 4Fluminense Federal University, Rio de Janeiro, Brazil
- 5Pesquisa e Desenvolvimento, Grupo Boticário, Curitiba, Brazil
Titanium dioxide nanoparticles (TiO2 NPs) are regularly used in sunscreens because of their photoprotective capacity. The advantage of using TiO2 on the nanometer scale is due to its transparency and better UV blocking efficiency. Due to the greater surface area/volume ratio, NPs become more (bio)-reactive giving rise to concerns about their potential toxicity. To evaluate the irritation and corrosion of cosmetics, 3D skin models have been used as an alternative method to animal experimentation. However, it is not known if this model is appropriate to study skin irritation, corrosion and phototoxicity of nanomaterials such as TiO2 NPs. This systematic review (SR) proposed the following question: Can the toxicity of TiO2 nanoparticles be evaluated in a 3D skin model? This SR was conducted according to the Preliminary Report on Systematic Review and Meta-Analysis (PRISMA). The protocol was registered in CAMARADES and the ToxRTool evaluation was performed in order to increase the quality and transparency of this search. In this SR, 7 articles were selected, and it was concluded that the 3D skin model has shown to be promising to evaluate the toxicity of TiO2 NPs. However, most studies have used biological assays that have already been described as interfering with these NPs, demonstrating that misinterpretations can be obtained. This review will focus in the possible efforts that should be done in order to avoid interference of NPs with biological assays applied in 3D in vitro culture.
Introduction
The development of the nanotechnology area, concerning to the nanomaterials production, is in exponential growth (Wang and Tooley, 2011). According to the International Organization for Standardization (ISO), nanomaterial is defined as natural, incidental or manufactured material containing particles (unbound, aggregated, agglomerated state), where 50% or more of the particles, have one or more external dimensions in the size range between 1 and 100 nm (Potočnik, 2011; International Organization for Standardization, 2017).
Due to their nano dimensions, they can effectively have electrical, thermal, and mechanical features, desirable for several applications (Davis et al., 2010; Louro et al., 2019; Lüderwald et al., 2019). Consequently, the human exposure to nanoparticles (NPs) increased as a result of their use in industries such as: food, pharmaceutical, cosmetic, biomedical (medical devices: implants, prostheses, controlled drug delivery systems), aeronautics, textiles as well as environmental engineering (Kongsong et al., 2014; Sethi et al., 2014; Semenzin et al., 2015; Miyani and Hughes, 2016; Hanawa, 2019; Shetti et al., 2019).
Regarding cosmetics, it is already known that many products contain various types of nanometric materials such as: gold, zinc oxide, titanium dioxide, nanotubes, fullerenes, among others (Morganti, 2010). Some of the referred nanostructures were introduced in sunscreens, with the final goal of protecting skin from solar radiation, reducing the chances of melanoma and also early skin aging (Wolf et al., 2001; Rampaul et al., 2007). NPs are among the best photoprotective agents since they are able to block the ultraviolet radiation incidence (González et al., 2008). Currently, titanium dioxide nanoparticles (TiO2 NPs) are the nanostructures mostly used in commercially available sunscreens, due to their ability to reflect and spread ultraviolet A (UVA, 320–400 nm) and ultraviolet B (UVB, 290–320 nm) rays, protecting against sunburn and photoaging (Monteiro-Riviere et al., 2011; Martirosyan and Schneider, 2014). TiO2 was previously classified as an inert particle, unable to be absorbed by the skin (Nohynek et al., 2007). When these sunscreens were created, TiO2 was used in the micrometric scale, being visible in the skin as an opaque layer. With the advancement of nanotechnology and aiming to solve this undesirable visual effect, TiO2 NPs were introduced in the formulations. The EU's Scientific Committee on Consumer Safety (SCCS) approved nanometric titanium dioxide (in the three crystalline forms) to be considered safe for use in cosmetic products intended for application on healthy, intact or sunburnt skin. As UV filter it can be introduced in cosmetic formulations at a maximum concentration of 25%, except in applications that may lead to exposure of the end user's lungs by inhalation. The benefits of using TiO2 NPs is their high surface area, increased properties of scattering and reflection of ultraviolet rays and transparency in visible light (Wiesenthal et al., 2011). In contrast, this size reduction of TiO2, increases their chances of internalization by skin cells with possible biological consequences to consumers.
Some in vitro literature already described that TiO2 NPs induce toxicity, inflammation and genetic modifications that is enhanced upon UVA and UVB exposure (Jin et al., 2011; Shi et al., 2013; Tucci et al., 2013; Zhao et al., 2013; Wang et al., 2014). The possible mechanisms of toxicity include oxidative stress, where TiO2 NPs trigger the formation of reactive oxygen species (ROS) in different dermal cell lines (Tucci et al., 2013; Zhao et al., 2013; Wang et al., 2014; Foroozandeh and Aziz, 2015). ROS involvement in oxidative DNA damage, in human epidermal, HaCaT cells (Shukla et al., 2014) and human dermal fibroblasts (Saquib et al., 2012) was already reported. Resuming dermal toxicity is associated with ROS generation, oxidative stress, and collagen depletion that promote skin aging (Wu et al., 2009). Actually, the strategy in the sunscreen industry is to coat nano-TiO2 in order to minimize their potential toxicity (Dréno et al., 2019). Compounds such as: silica, alumina, cetylphosphate, manganese dioxide, triethoxycaprylylsilane, PEG among others, contribute to making sunscreens more passive, improving their ability of capture or inhibit the formation of free radicals' species as well as to restrain NPs penetration in the skin (Filipe et al., 2009; Osmond and McCall, 2010; Smijs and Pavel, 2011). These alterations in NPs surface characteristics give rise to the need of a new set of physicochemical characterization, in vitro and in vivo evaluation, since surface modification regulates both inter-particle and cell-NP interactions, mediating corona formation that is widely known to induce specific cellular responses such as cellular uptake, intracellular trafficking, accumulation and biodistribution (Oberdörster et al., 2005; Filipe et al., 2009; Osmond and McCall, 2010; Foroozandeh and Aziz, 2015; Ribeiro et al., 2017; Sanches et al., 2019). Results suggest that benefitting from a physical barrier in the form of a coating minimizes ROS formation and consequent dermal toxicity (Yu et al., 2020).
The in vitro and in vivo data regarding the potential of dermal absorption and/or penetration of TiO2 NPs from sunscreens exhibit controversial results. Although several articles describe the opposite (Filipe et al., 2009; Senzui et al., 2010; Crosera et al., 2015), the penetration of TiO2 NPs in healthy as well as in damaged or lesioned skin (such as in cases of scarring, sunburn and depilated skin) is demonstrated in the scientific community (Tan et al., 1996; Lekki et al., 2007; Gontier et al., 2008; Schneider M. et al., 2009; Lin et al., 2011; Monteiro-Riviere et al., 2011; Larese Filon et al., 2013; Gulson et al., 2015; Shakeel et al., 2016; Touloumes et al., 2020), with damaged or lesioned skin being more susceptible to TiO2 NPs penetration (Tan et al., 1996; Lekki et al., 2007; Gontier et al., 2008; Schneider M. et al., 2009; Lin et al., 2011; Monteiro-Riviere et al., 2011; Larese Filon et al., 2013; Gulson et al., 2015; Shakeel et al., 2016; Touloumes et al., 2020). Prolonged application of TiO2 NPs sunscreens in healthy human skin, reveal the detection of titanium levels in the epidermis and dermis of patients (Tan et al., 1996; Lin et al., 2011; Gulson et al., 2015; Næss et al., 2015; Shakeel et al., 2016). Recently, although the study has some limitations (few number of volunteers), Pelclova et al. using highly sensitive characterization techniques detected TiO2 NPs in plasma and urine after 6 to 48 h after sunscreen exposure, demonstrating that TiO2 NPs can pass the healthy protective layers of human skin and enter in blood circulation, even with lower exposure times of sunscreen application (Pelclova et al., 2019). The penetration of NPs is not exclusive to TiO2 NPs, with Brian Gulson et al. reporting also the detection of zinc oxide used in sunscreens in human blood and urine (Gulson et al., 2010). From our point of view, and also already stated by OECD report that evaluate the in vitro methods for human hazard assessment of nanomaterials (Organisation for Economic Co-operation Development, 2018), there exist many critical points in the available literature that contribute to all the controversy regarding TiO2 NPs penetration in human skin. It is important to refer that several factors such as: the model employed (animal, human with variations in gender), size, chemical composition and coating of NPs, site of application, particle solubility, dose and number of applications, period of the study, the flexion motion of skin, UV exposure among others, influence the dermal penetration of nanoparticles (Gulson et al., 2015; Shakeel et al., 2016). To the best of our knowledge, most of the studies are performed with a strong variety of conditions and methodologies, where no standardized protocols and reference nanomaterials are used. More important is that the characterization techniques used to evaluate NPs skin penetration were sometimes entering in the detection limit of the equipment, contribute to all this controversy.
The mechanism of penetration of sunscreen nanoparticles was not clarified, however, it is suggested that TiO2 NPs can be absorbed follow different pathways that include the transcellular and paracellular transports, as well as hair follicles (transappendageal), sweat glands, skin folds or a combination of all, as shown in Figure 1 (Filipe et al., 2009; Wu et al., 2009). The fact that upon skin penetration, NPs can reach the bloodstream, and then undergo translocation to various distant tissues and organs, suggest that prolonged time exposure to NPs, may pose a health risk to consumers (Lademann et al., 1999; Baroli et al., 2007; Lekki et al., 2007; Saquib et al., 2012; Shakeel et al., 2016).
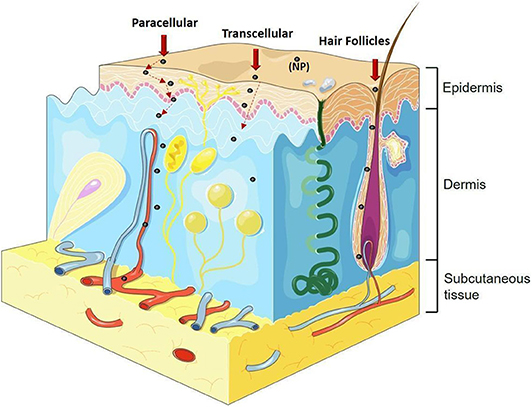
Figure 1. Different pathways of nanoparticle penetration. Paracellular transport (between cells), transcellular transport (inside the cells), transport by hair follicles, sweat glands, skin folds or a combination of all. Image adapted from: https://smart.servier.com.
As previously described, there are already many in vitro (using 2D models) and in vivo (using animals) studies on the cytotoxicity and genotoxicity effect of TiO2 NPs. However, cosmetic industries are using alternative methods, such as 3D skin reconstructed models, due to ethical, scientific and economic considerations. The EU cosmetic legislation is working toward the abolition of animal testing for cosmetics and their ingredients (Evans et al., 2016; Salamanna et al., 2016; Caddeo et al., 2017; Alépée et al., 2018; Owen et al., 2018). In fact, 3D engineered models mimicking human tissues are under development to overcome the limitations of 2D in vitro models regarding their limited predictivity (Vernetti et al., 2017). Currently, there are commercially available 3D skin models as well as in-house constructs with several levels of biological complexity. Figure 2 exhibits some of the commercially available models.
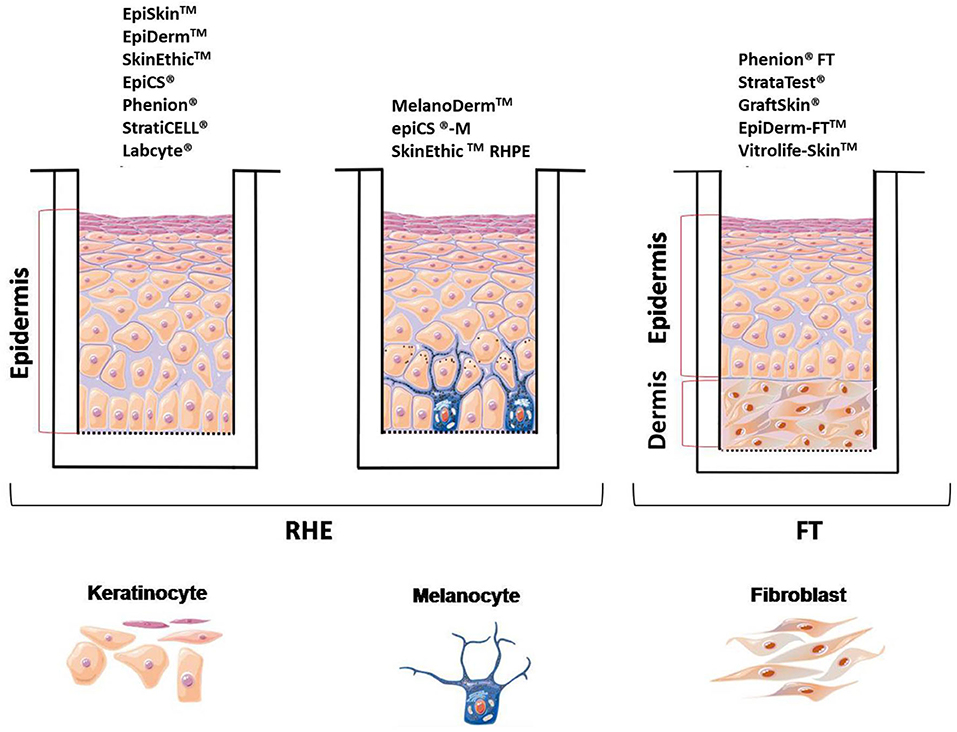
Figure 2. Scheme of the main 3D skin models used. Image adapted from https://smart.servier.com.
The simplest model consists of an epidermis where only keratinocytes are used, it is known as reconstructed human epidermis (RHE) and it is commercially available as EpiDerm™ (MatTek Corp), EpiSkin™ and SkinEthic™ (a subsidiary of L'Oreal). They make use of reconstructed human epidermis, which closely mimics the histological, morphological, biochemical, and physiological properties of the epidermal layer of human skin (Kim et al., 2016). The advantage of RHE models is that they contain all the epidermal layers of skin, with the main disadvantage of, it is not always possible to distinguish the basale, spinosum and granulosum stratum, important issue for penetration studies. Also, a low intra-batch variation and sometimes a high inter-batch variation is observed (Mathes et al., 2013; Almeida et al., 2017). In April 2007, EpiSkin™ and Epiderm™ models were approved by ECVAM (European Center for the Validation of Alternative Methods) to replace in vivo rabbit skin irritation test. They are also used for other regulatory purposes, such as in vitro skin irritation (OECD TG 439) and skin corrosion (OECD TG 431) tests of cosmetic ingredients. An update in 2019 was made to include two additional models namely SkinEthic™ and epiCS® (OECD, 2014, 2019). Besides that, these models are widely used for phototoxicity, genotoxicity, sensitization, metabolization tests as well as to test the administration of transdermal drugs (Mathes et al., 2013; Almeida et al., 2017). EpiSkin, for example, is an in vitro reconstructed human epidermis from normal human keratinocytes cultured on a collagen matrix at the air-liquid interface that is validated for skin corrosion/irritation studies (Alépée et al., 2018; Liu et al., 2018). Cell viability is the main endpoint, however complementary assays can be performed, such as gene array, histological, morphological analysis and cytokine release (Sarmento et al., 2012; Almeida et al., 2017). The Full-Thickness Skin Model (FT), commercially known as EpiDerm-FT™ is a more elaborated model that consists of an epidermis and dermis (keratinocytes and fibroblasts) and has been widely used in drug or efficacy treatments (Mathes et al., 2013). This model has the advantage of providing wall-to-wall tissue, as well as having a basal membrane similar to in vivo when compared to RHE models (simplest model). The permeability of RHE models is inferior to human and pig skin, however they are accepted to test in vitro permeation and penetration studies when drugs are applied as aqueous solutions (Asbill et al., 2000; Schäfer-Korting et al., 2008; Neupane et al., 2020). Resuming for in vitro skin corrosion tests following OECD TG 431, the commercially available models are: EpiSkinTM Standard Model (SM), EpiDermTM SCT, SkinEthicTM RHE, epiCS®, and LabCyte EPI-MODEL24 SCT (OECD, 2014). Regarding in vitro skin irritation following TG 439 the used models are: EpiSkinTM (SM), EpiDermTM (SIT), SkinEthicTM (RHE), LabCyte EPI-MODEL24 SIT, epiCS® and Skin+ ® (OHAT, 2015). In order to test sun care products companies are also developing 3D skin models incorporating melanocytes (e.g., MelanoDermTM, MatTek corp.; epiCS®-M, ATERA SAS & CellSystems Gmbh; and SkinEthicTM RHPE subsidiary of L'Oréal). Although 3D skin models have been used by the cosmetic industry for corrosion and skin irritation testing of chemical formulations, to the date it is not known whether this models are appropriate for studying the cytotoxicity, skin corrosion, irritation, and phototoxicity of formulations containing TiO2 NPs. For this reason, this systematic review aims to answer the following proposed question: Can the toxicity of TiO2 nanoparticles be assessed in the 3D skin model?
Materials and Methods
This systematic review (SR) was conducted in accordance with the Cochrane Handbook for Systematic Reviews of Interventions (Higgins and Green, 2011), the Preferred Reporting Items for Systematic Review and Meta-Analyses (PRISMA) guidelines (Moher et al., 2009) and the Office of Health Assessment and Translation (OHAT) handbook (OHAT, 2015), which was developed by National Toxicity Program. The protocol of this SR was registered in CAMARADES at http://www.dcn.ed.ac.uk/camarades/research.html#protocols. Also, a reliability assessment of in vitro toxicity studies named Toxicological data Reliability Assessment Tool (ToxRTool), was followed to increase the quality and transparency of this search.
Focused Question (Based on PICO Strategy) (Schardt et al., 2007)
• Population 3D skin model
• Interventions or exposure of TiO2 NPs in the 3D model
• Comparison 3D model without TiO2 NPs exposure
• Outcome Effects generated by TiO2 NPs in the 3D skin model: cytotoxicity, phototoxicity, irritation, corrosion.
• Study design in vitro studies.
Search Strategy
An electronic search was carried out in the MEDLINE/PubMed, Science Direct, Web of Science, Scopus and SciELO library databases up to February 2019. Only studies in English, Portuguese, Spanish, or French were selected, without date restriction. In addition, searches in the references of the included studies (i.e., cross-referencing) were conducted. Furthermore, unpublished studies (i.e., gray literature) were analyzed in the Gray Literature Report and OpenGrey databases. A specific search strategy was used for each database, according to its characteristics (Table 1).
Eligibility Criteria (OHAT)
The inclusion and exclusion criteria are stated in Table 2.
Study Selection, Screening Process, and Data Extraction
Titles and abstracts of the retrieved articles were screened by two authors/reviewers (PS and LG) and then publications that fulfilled the inclusion criteria were identified. Disagreements between the reviewing authors were resolved through careful discussion, and any remaining disagreements were resolved by a third reviewer (JMG). After that, full text of eligible articles was obtained. Finally, based on the inclusion criteria two authors/reviewers (PS and LG) independently screened and selected the relevant full-text articles. Furthermore, disagreements between the reviewing authors were resolved through the same process used in the first selection phase.
When available, the following data were extracted from the publications by the reviewers (PS and RC): year, DOI, type of skin model, test substance, the crystalline structure of TiO2 NPs, primary particle size, size of the TiO2 after dispersion, exposure time, the expected outcome of the positive and negative controls, results in the evaluation of corrosion and/or irritation and phototoxicity and main conclusion.
Assessment of Reliability
The reliability assessment was done by two reviewers (PS and LG), using the ToxRTool developed by the European Center for the Validation of Alternative Methods (ECVAM) (Schneider K. et al., 2009). The in vitro part of this tool consists of a list of 18 criteria. Each criterion can be graded as “1” (i.e., “criterion met”) or as “0” (i.e., “criterion not met” or not reported). Those 18 criteria are grouped in five major groups: I-Test substance identification, II-Test system characterization, III-Study design description, IV-Study results documentation and V-Plausibility of study design and results. A final score was then recorded for each major group of each article, and an overall score was recorded for each study.
In this tool, there are some criteria considered indispensable for a study to be reliable, and they are highlighted in red (presented in Supplementary Information). Independently of the overall score, only if these criteria are assigned as “1” the tool will rate the study as a reliable category (1 or 2). Those categories are: 1 (reliable without restrictions), 2 (reliable with restrictions), 3 (not reliable) and 4 (not assignable).
Finally, ToxRTool classified under “A” box the category in which the article was assigned based on the sum of points (overall score), regardless the red criteria. And, under “B” the category is derived considering the red criteria.
Results
Search
The initial search identified 43 articles, comprising 11 titles from MEDLINE/PubMed, 13 from Science Direct, 12 from Scopus, and 7 from Web of Science. The search in the gray literature or cross-referencing did not yield any studies. After duplicates removal, 31 studies had their titles and abstracts screened, and 24 studies were excluded because they did not meet the eligibility criteria. Then, the full-text screening did not exclude any studies, resulting in 7 included articles (Park et al., 2011; Choi et al., 2014; Kato et al., 2014; Horie et al., 2016; Kim et al., 2016; Miyani and Hughes, 2016; Tang et al., 2018) in this systematic review (Figure 3 Prisma Flow diagram).
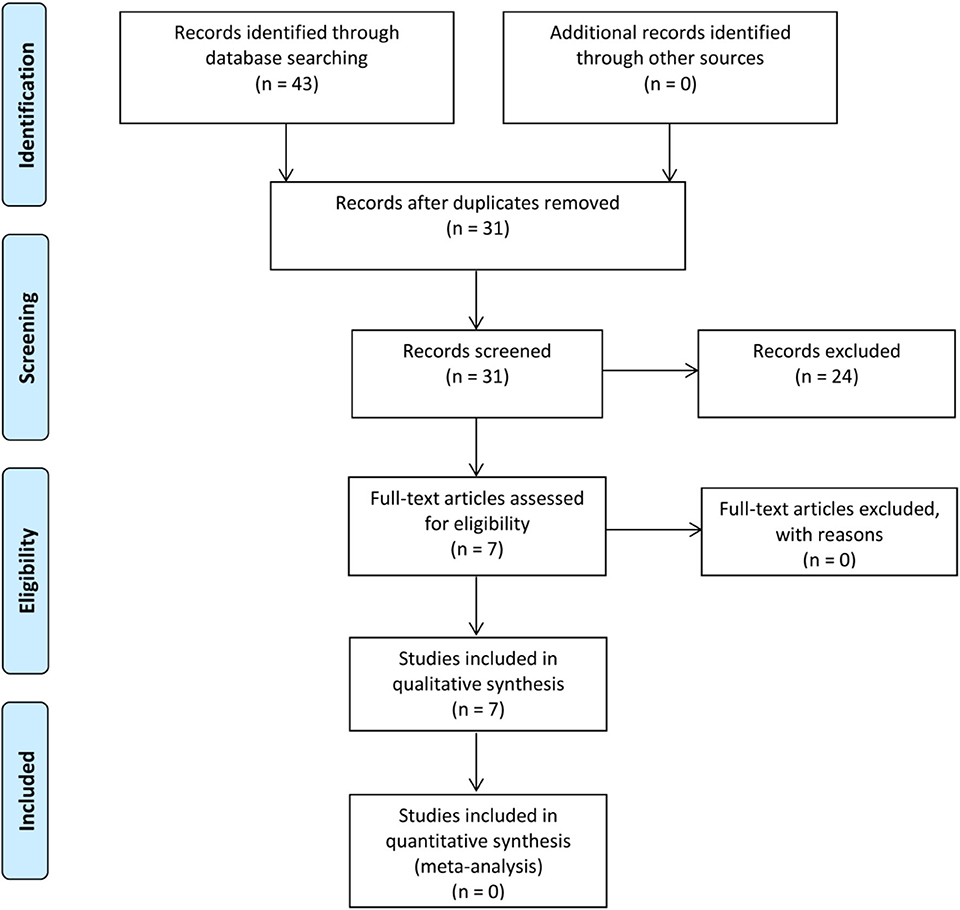
Figure 3. Flow diagram of literature selection process, from Moher et al. (2009).
Assessment of Reliability
As described previously, the reliability assessment was done using the ToxRTool tool. It can be observed that a few criteria were not met by the authors. The number of replicates and the statistical methods for data analysis given is some of the criteria that have not been described by some authors. In Table 3 it is possible to observe the general punctuation and categories of each selected article. Thus, all articles were classified in category A with “1,” which corresponded to that the articles that were considered as reliable without restrictions. Articles were also classified in category B with “1” when all criteria considered indispensable for the study was reliable and attended.
Study Characteristics
From the seven articles found and analyzed, four studies used EpiDerm™ (MatTek Corporation) (Park et al., 2011; Horie et al., 2016; Kim et al., 2016; Miyani and Hughes, 2016), one used the EpiKutis™ (Biocell Biotechnology, China) (Tang et al., 2018), one used KeraSkin (Modern Cell & Tissue Technology, Seoul, Korea) (Choi et al., 2014) and the last one was developed in their own laboratory (Kato et al., 2014). According to the inclusion criteria, all seven articles used TiO2 NPs as test substance. As it is well known, TiO2 NPs exhibits three crystalline forms: rutile, anatase, and brookite. However, some studies also use mixtures of anatase and rutile structures. From the selected articles, two articles used the rutile crystalline structures (Choi et al., 2014; Kato et al., 2014), two articles used the mixture (anatase and rutile) (Park et al., 2011; Kim et al., 2016), one article used two crystalline structures, anatase and rutile (Horie et al., 2016), and two articles used anatase, rutile, and mixture (Miyani and Hughes, 2016; Tang et al., 2018). Some authors have also used other test substances such as: NPs of zinc oxide, zinc oxide/titanium dioxide (Choi et al., 2014), silver (Kim et al., 2016; Miyani and Hughes, 2016), cerium dioxide (Miyani and Hughes, 2016), iron oxide (Kim et al., 2016), aluminum oxide (Kim et al., 2016), polystyrene (Park et al., 2011) and polyvinylpyrrolidone-entrapped fullerene-C60 (Kato et al., 2014).
The primary size of the TiO2 NPs ranged from 6 to 108 nm. Regarding the size of agglomerates after dispersion, as it can be seen in Table 4, only three authors precisely described NPS size (Choi et al., 2014; Horie et al., 2016; Tang et al., 2018), two authors did not describe the size (Kato et al., 2014; Miyani and Hughes, 2016), one author demonstrated the agglomerate size by transmission Electron Microscopy image (which is apparently around 200 nm (Park et al., 2011) and one author states that the size of NPs was several hundred nanometers (Kim et al., 2016).
The skin irritation test was performed in five articles. Two authors have done corrosion tests, and the other two performed phototoxicity tests. In addition to these tests, in some article's histopathology, cytokine assay, lactate dehydrogenase assay, IL-8 enzyme-linked immunosorbent assay, IL-1α assay, relative HO-1 gene expression, intracellular ROS-generation, and lipid hydroperoxides were performed.
Three authors used the concentration of 100 μg/mL, however, each author used different exposure times: 1 h (Park et al., 2011), 2 h (Tang et al., 2018) and 4 h (Horie et al., 2016). In addition, one author used the concentration of 15 μg/mL for 3 h of exposure (Kato et al., 2014), another used the concentration of 1 mg/mL for 1 h of exposure (Miyani and Hughes, 2016). Furthermore, two authors used the same exposure period of 3 min and 1 h. In one study the epidermis was moistened with deionized water and 25 mg of the test substance (Kim et al., 2016) and in another study, 25% of the test substance in deionized water was used (Choi et al., 2014).
Toxicity Results
Skin Irritation
All articles that assessed skin irritation used 3- (4,5-dimethylthiazol-2-yl)−2,5-diphenyltetrazolium bromide (MTT) assay (Park et al., 2011; Choi et al., 2014; Kim et al., 2016; Miyani and Hughes, 2016). In all cases, TiO2 NPs was shown to be non-irritating on the 3D model, where no reduction in cell viability was observed. In all studies, the results were compared with the positive and negative controls, where Sodium Dodecyl Sulfate (SDS) was used as a positive control, Dulbecco's Phosphate Buffered Saline (DPBS) and Phosphate Buffered Saline (PBS) were used in some studies as a negative control.
Skin Corrosion
Two authors used TiO2 NPs to evaluate skin corrosion (Choi et al., 2014; Kim et al., 2016). Choi et al. and Kim et al., used potassium hydroxide (8N KOH) as a positive control (Choi et al., 2014; Kim et al., 2016). Choi et al. observed that after 3 min of exposure with 8N KOH the viability was reduced to 1%, while the TiO2 NPs treated sample viability was 94% (± 3.0). After 60 min of TiO2 NPs exposure, it was observed that the viability reduced in comparison to the time of 3 min, however, the viability was higher than 50% (Choi et al., 2014). Kim et al. presented similar results. They demonstrated that after 3 min of exposure, treatment with 25 mg of TiO2 NPs led to the viability of 96.3% (± 2.4) while 8N KOH reduced viability to 9.8% (± 1.6). After an exposure time of 60 min, the viability of the treated sample with TiO2 NPs decreased to 85.3% (±3.9) (Kim et al., 2016). Therefore, the two studies concluded that TiO2 NPs are non-corrosive, considering that viability was >50 and 15% after 3 and 30 min of exposure, respectively.
Phototoxicity
The assessment of TiO2 NPs phototoxicity in the 3D skin model was performed in two articles. It was applied variable exposition times, non-toxic doses of UVA of 6 J/cm2 (Park et al., 2011) and 40 J/cm2 (Tang et al., 2018). In both cases, phototoxicity was evaluated by the reduction of mitochondrial conversion of MTT to formazan. The authors concluded that TiO2 NPs did not exhibit phototoxicity in the 3D skin model in the presence of UV radiation.
Discussion
With the advancement of nanotechnology, many products with nanoscale materials have been introduced in several areas such as cosmetics, food, drugs and electronics (Louro et al., 2019; Lüderwald et al., 2019). Therefore, exposure to nanomaterials is in exponential growth, and can occur both during the synthesis as well as in the use of the final product. Due to the greater surface area/volume ratio, NPs become more (bio) reactive compared to normal bulk materials, giving rise to concerns about their potential toxicity to humans (Sharifi et al., 2012; Shi et al., 2013).
TiO2 NPs are widely used in the cosmetic industries, especially in sunscreens as an alternative to available chemical UV absorbers (p-aminobenzoic acid and benzophenones) that are known to cause some allergic reactions and/or endocrine disruption. Titanium dioxide has three different crystalline structures (anatase, rutile and brookite), however, rutile is the most used in cosmetic, due to its high refraction index, protecting skin from the harmful effects of ultraviolet rays (Martirosyan and Schneider, 2014). The International Agency of Cancer Research (IARC) classified titanium dioxide as a possible human carcinogen (group 2B), however, there is no distinction regarding titanium size (macro, micron and/or nanoscale). The heterogeneity of TiO2 nanoparticles (particle size distribution; agglomeration and aggregation; morphology, crystal structure; purity) for sunscreen applications is high, becoming fiercely debated the hazard of TiO2 NPs (Jacobs et al., 2010).
A long time ago that cosmetic industries were testing their products using corrosion and skin irritation tests in rabbits (in accordance with the Organization for Economic Co-operation and Development (OECD) (OECD, 2015). However, due to ethical (3 R principle–replacement, refinement and reduction of animal trials), scientific and economic restrictions, skin bioengineering 3D tissues (Evans et al., 2016; Salamanna et al., 2016; Caddeo et al., 2017; Alépée et al., 2018; Liu et al., 2018; Owen et al., 2018) are actually used for testing new pharmaceuticals. The main advantages of the bioengineering 3D tissues are their physiological relevance since they recapitulate tissue's microenvironment, increased reproducibility comparing to ex-vivo models, superior predictive potential due to the possible use of human cells, along with decreased in cost and ethical concerns (Sarmento et al., 2012; Gholobova et al., 2018; Madl et al., 2018; Qiao and Tang, 2018). High quality skin constructs (that mimic the morphology, lipid composition and differentiation of native human skin) are commercially available. Current available 3D skin constructs still do not contain all essential cell types (dendritic cells as well as macrophages), neither integrate blood vessels, neither the dynamic crosstalk between epithelium and connective tissue, that are essential to regulate epidermal morphogenesis and homeostasis. This reinforces the need of more complex 3D constructs that can address complex toxicological endpoints. Nowadays the main strategy is to use biofabrication technique's such as electrospinning and bioprinting to develop relevant skin biological models constituted by specialized cell types (melanocytes, adipocytes, Langerhans, immune, stem cells, among others) with a perfused vasculature allowing a physiological oxygen and nutrient delivery (Mathes et al., 2013). The main goal is to achieve a physiological relevant 3D skin construct that can be used for toxicology assessment of new cosmetic formulations but also anti-cancer drugs development for example, reducing the animal studies.
A few articles study the hazard effect of TiO2 NPs in a three-dimensional skin model. Mostly, available literature uses quite diverse in vitro (primary cells and cell lines derived from different organs/tissues) and in vivo models to evaluate the hazard effect of TiO2 NPs. Results suggest that TiO2 NPs induce the release of ROS leading to loss of vital cellular functions, ultimately leading to cell death and accumulate in liver and spleen preferentially (Jin et al., 2011; Shi et al., 2013; Tucci et al., 2013; Zhao et al., 2013; Wang et al., 2014; Carriere et al., 2016; Proquin et al., 2016).
This systematic review demonstrates that the 3D skin models used, mimic the histology, morphological, physiological and biochemical properties of human epidermis. The reliability of these studies was evaluated by ToxRTool, and all 18 criteria evaluated by this tool are presented in Tables S2–S8 (Supplementary Information). This table describes a simulation of a work that meets 100% of the criteria as well as the evaluation performed in the seven selected articles (see Table S1).
As it can be observed in Table 3, all articles were well-evaluated, however, when analyzing Table 4, it was observed the diversity of the study design. Each article worked with TiO2 NP unknown stability possibly holding large and sedimenting agglomerates, different TiO2 NPs exposure times, crystal structures and surface coatings, dissimilar measures for exposure doses (mass, area or particle number) that in practice result in a difficulty to convert into each other as well as did not adequately characterize particles' size and stability during cell exposure. From the 7 articles analyzed, six of them concluded that TiO2 NPs are non-irritating, non-corrosive and non-phototoxic (Park et al., 2011; Choi et al., 2014; Kato et al., 2014; Horie et al., 2016; Kim et al., 2016; Miyani and Hughes, 2016; Tang et al., 2018). What was noticed was that in the six articles analyzed, the toxicity of TiO2 NPs was evaluated using the MTT assay, which is reported in the literature to interfere with TiO2 NPs (Kroll and Hendrik, 2012; Ong et al., 2014). TiO2 NPs interference is attributed to the light adsorption properties of NP over the same spectral region used by MTT (Holder et al., 2012; Kroll and Hendrik, 2012; Ong et al., 2014). However, NPs also adsorb constituents of the assay on their surface that obstruct proper transformation of molecules, chemical reactions between the NPs and the test compounds can occur and even the release of metal ions from NPs can modify the mitochondrial catalytic activity of cells altering MTT reading (Kroll et al., 2009). Some authors reported that interference increases with increased NP concentration (Holder et al., 2012; Kroll and Hendrik, 2012; Ong et al., 2014), and emphasize that it is necessary to use concentrations of NPs that do not reduce MTT. It is important to stress that in the last years, extensive washing steps were introduced in the protocols and the possible interference of TiO2 NPs with the MTT assay was reduced and in some cases overcome (Kroll and Hendrik, 2012; Ong et al., 2014). However great care must be taken, because even with multiple washes or centrifugations, TiO2 NPs can remain adhered to the culture plate or even adsorbed on the surface of the cells. The contribution of NPs to the MTT light absorption signal depends also with the concentration of reduced MTT-formazan present in the MTTred/MTTox mixtures, suggesting different mechanisms of interference that cannot be predicted a priori (Kroll and Hendrik, 2012). It seems that redox- active metals with different sizes and coatings can change the magnitude of the reaction kinetics causing different levels of interference (Mello et al., 2020). Figure 4 shows an interference scheme of NP with the MTT assay. As it is possible to observe the properties of the NPs can generate artifacts and misinterpretation of the results (Holder et al., 2012; Kroll and Hendrik, 2012; Guadagnini et al., 2013; Lupu and Popescu, 2013; Ong et al., 2014; Lammel and Sturve, 2018). A recent article demonstrated that TiO2 NPs adsorbed on cell surface and those internalized by cells interfere with the fluorescence readout by reflecting/absorbing part of the incident and emitted light (Lammel and Sturve, 2018). This adds new complexity to NPs hazard evaluation since each specific cell type characteristics influence NPs internalization, intracellular trafficking and final destiny.
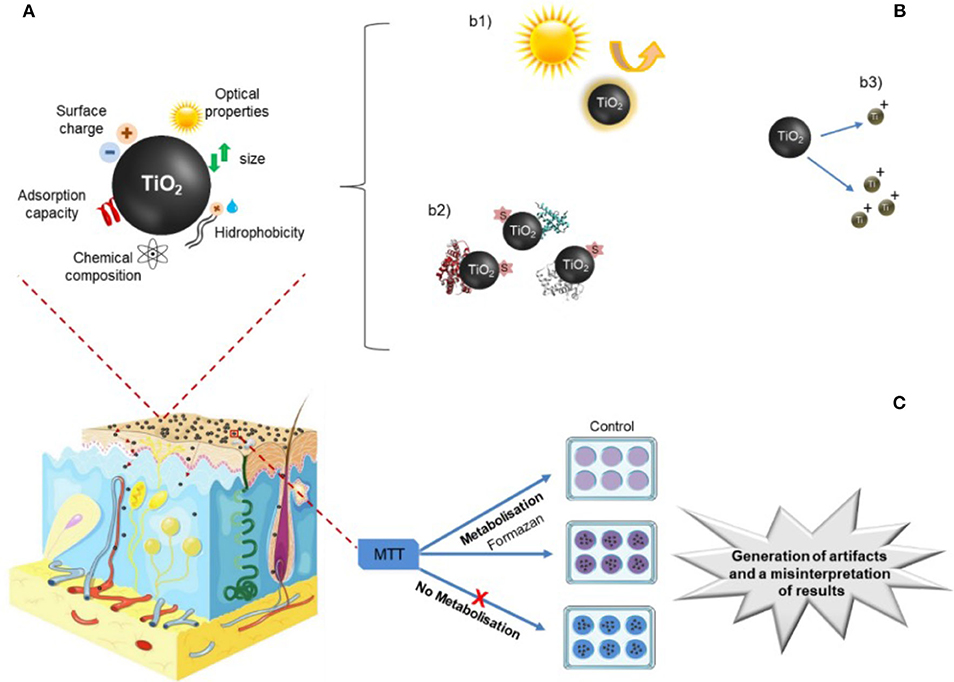
Figure 4. Toxicological assays. (A) Physico-chemical characteristics of NPs TiO2 NPs that possible interfere with the biological assays; that include (B) optical capabilities of TiO2 NPs such as intrinsic absorbance and/or fluorescence (b1); adsorption of proteins, salts and dyes (s) to NPs (b2); and dissolution of NPs with the consequent release of metal ions to supernatant (b3). (C) Conventional MTT test analyzing TiO2 NPs demonstrating that NPs can adsorb to MTT dye that avoid metabolization of reagents. Image adapted from: https://smart.servier.com.
Besides MTT, other biological assays interfere with TiO2 NPs (see Table S9 in Supplementary Information). Lactate dehydrogenase (LDH) assay was also reported to interfere with TiO2 NPs, since they can adsorb or inactivate LDH protein (Holder et al., 2012; Kroll and Hendrik, 2012), as well as the Neutral Red (NR) (Guadagnini et al., 2013), Alamar Blue assay (Ong et al., 2014; Lammel and Sturve, 2018),5-Carboxyfluorescein diacetateacetoxymethylester (CFDA-AM) assay (Lammel and Sturve, 2018) and 2',7'-Dichlorofluorescein (DCF) (Kroll and Hendrik, 2012). Furthermore, interferences may be cumulative when for example two fluorometric assays are used on the same cells to quantify a unique endpoint.
Assessing the toxicity of TiO2 NPs, is not a trivial issue. Therefore, researchers need to use every time that it is possible validated protocols for skin irritation, corrosion and phototoxicity testing with 3D models. Apply concentration exposures that mimic real situations but at the same time concentrations below interfering levels. Every time that it is possible introduce centrifugation, several washes, or even removing supernatants, are recommended approaches to reduce interference. We believe that with effective washing procedures, we can expect that NPs cannot effectively and sufficiently interact with MTT in the way that could significantly alter the results, however we need to take in consideration that this approach introduces another concern regarding exposure characterization and applied dose metrics. Resuming assays adaptations have to be ascertained case by case with a series of control experiments for each NP to obtain reliable nanotoxicity data. We also suggest perform complementary tests such as the evaluation of cytokines, histopathology and evaluation of cell membrane integrity by detecting transepithelial electrical resistance (TEER), always working with specific controls.
A long time ago that the nanotoxicology community has been addressing technical questions, such as dosing issues, aggregation state of materials as a function of time. We believe to deliver a realistic risk assessment of NPs, it is necessary to identify the key physicochemical characteristics that can foresee toxicological results, work with exposure conditions that mimic a real situation and characterize NPs and their interactions with biological systems (example: protein corona, its transformational capacity in the biological system). Very subtle alterations in the properties of NPs can completely alter protein corona that gets absorbed onto the NPs resulting in surprising changes in vivo. As the degree of interference may be relevant depending on the optical properties of the NPs, stability of NP, cytotoxicity testing and the type of fluorometric endpoint assay, it is encouraged to use more than one in vitro assay (for example flow cytometry is considered the method with less interference with NPs), specifically with different detection methods and use adequate controls (as negative controls, it would be advisable to separately test the dispersant agents used as NPs stabilizers under the same conditions). Adequate reference materials are needed in toxicological studies and efforts should be done in order to evaluate more than cell viability (ex: cell cycle) (Singh et al., 2019).
Conclusion
Through the data obtained by this systematic review, TiO2 NPs was considered non-irritant, non-corrosive and non-phototoxic for the 3D skin model, regardless of the crystalline type, and the size of the NPs studied. We can conclude that skin 3D models can be used to test the hazard effect of TiO2 NPs, however, we emphasize the need of standardized protocols with efficient washing procedures to remove the NP from the surface of 3D skin model to avoid potential interfere of TiO2 NPs with the assay.
Data Availability Statement
All datasets generated for this study are included in the article/Supplementary Material.
Author Contributions
PS, LG, RC, AR, and JG contributed to study concept and design. PS, LG, and RC contributed to the literature search and data collection. PS, LG, RC, DS, ML, AR, and JG contributed to data analysis. PS, RC, and AR wrote the paper. All authors contributed to a critical revision of the manuscript.
Funding
This work was supported by National Council for Scientific and Technological Development, CNPq (grants 405030/2015-0, 306672/2016-2, 467513/2014-7, and 400030/2018-7); FAPERJ (Grant nos. E-26/102.993/2012, E-26/203.012/2016), and Coordenação de Aperfeiçoamento de Pessoal de Nível Superior – Brasil (CAPES) (Finance Code 001), National Centre of Science and Technology on Regenerative Medicine – INCT Regenera (http://www.inctregenera.org.br/). AR especially thanks to Propesq-Unigranrio-FUNADEP Scholarship and Jovem Ciêntista do Nosso Estado award from FAPERJ. JMG thanks Cientista do Nosso Estado award from FAPERJ.
Conflict of Interest
The authors declare that the research was conducted in the absence of any commercial or financial relationships that could be construed as a potential conflict of interest.
Supplementary Material
The Supplementary Material for this article can be found online at: https://www.frontiersin.org/articles/10.3389/fbioe.2020.00575/full#supplementary-material
References
Alépée, N., Grandidier, M., and Cotovio, J. (2018). Usefulness of the EpiSkinTM reconstructed human epidermis model within integrated approaches on testing and assessment (IATA) for skin corrosion and irritation. Toxicol. Vitr. 54, 147–167. doi: 10.1016/j.tiv.2018.09.015
Almeida, A., Sarmento, B., and Rodrigues, F. (2017). Insights on in vitro models for safety and toxicity assessment of cosmetic ingredients. Int. J. Pharm. 519, 178–185. doi: 10.1016/j.ijpharm.2017.01.024
Asbill, C., Kim, N., El-Kattan, A., Creek, K., Wertz, P., and Michniak, B. (2000). Evaluation of a human bio-engineered skin equivalent for drug permeation studies. Pharm. Res. 17, 1092–1097. doi: 10.1023/A:1026405712870
Baroli, B., Ennas, M. G., Loffredo, F., Isola, M., Pinna, R., and Lo, M. A. (2007). Penetration of metallic nanoparticles in human full-thickness skin. Soc. Investig. Dermatol. 127, 1701–1712. doi: 10.1038/sj.jid.5700733
Caddeo, S., Boffito, M., and Sartori, S. (2017). Tissue engineering approaches in the design of healthy and pathological in vitro tissue models. Front. Bioeng. Biotechnol. 5:40. doi: 10.3389/fbioe.2017.00040
Carriere, M., Sauvaigo, S., Douki, T., Ravanat, J., Lésions, L., Alpes, U. G., et al. (2016). Impact of nanoparticles on DNA repair processes: current knowledge and working hypotheses. Mutagenesis 32, 203–213. doi: 10.1093/mutage/gew052
Choi, J., Kim, H., Choi, J., Oh, S. M., Park, J., and Park, K. (2014). Skin corrosion and irritation test of sunscreen nanoparticles using reconstructed 3D human skin model. Environ. Health Toxicol. 29:e2014004. doi: 10.5620/eht.2014.29.e2014004
Crosera, M., Prodi, A., Mauro, M., Pelin, M., Florio, C., Bellomo, F., et al. (2015). Titanium dioxide nanoparticle penetration into the skin and effects on HaCaT cells. Int. J. Environ. Res. Public Health 12, 9282–9297. doi: 10.3390/ijerph120809282
Davis, J., Wang, A., and Shtakin, J. (2010). Nanomaterial Case Studies: Nanoscale Titanium Dioxide in Water Treatment and in Topical Sunscreen (Final). Washington, DC: U.S. Environmental Protection Agency, EPA/600/R-09/057F.
Dréno, B., Alexis, A., Chuberre, B., and Marinovich, M. (2019). Safety of titanium dioxide nanoparticles in cosmetics. J. Eur. Acad. Dermatol. Venereol. 33, 34–46. doi: 10.1111/jdv.15943
Evans, S. J., Clift, M. J. D., Singh, N., Mallia, J. D. O., Burgum, M., Wills, J. W., et al. (2016). Critical review of the current and future challenges associated with advanced in vitro systems towards the study of nanoparticle (secondary) genotoxicity. Mutagenesis 32, 233–241. doi: 10.1093/mutage/gew054
Filipe, P., Silva, J. N., Silva, R., Cirne De Castro, J. L., Marques Gomes, M., Alves, L. C., et al. (2009). Stratum corneum is an effective barrier to TiO2 and ZnO nanoparticle percutaneous absorption. Skin Pharmacol. Physiol. 22, 266–275. doi: 10.1159/000235554
Foroozandeh, P., and Aziz, A. A. (2015). Merging worlds of nanomaterials and biological environment : factors governing protein corona formation on nanoparticles and its biological consequences. Nanoscale Res. Lett. 10:221. doi: 10.1186/s11671-015-0922-3
Gholobova, D., Gerard, M., Decroix, L., Des, L, Callewaert, N., and Annaert, P. (2018). Human tissue-engineered skeletal muscle : a novel 3D in vitro model for drug disposition and toxicity after intramuscular injection. Sci. Rep. 8:12206. doi: 10.1038/s41598-018-30123-3
Gontier, E., Ynsa, M. D., Bíró, T, Hunyadi, J., Kiss, B., Gáspár, K., et al. (2008). Is there penetration of titania nanoparticles in sunscreens through skin? A comparative electron and ion microscopy study. Nanotoxicology 2, 218–231. doi: 10.1080/17435390802538508
González, S., Fernández-Lorente, M., and Gilaberte-Calzada, Y. (2008). The latest on skin photoprotection. Clin. Dermatol. 26, 614–626. doi: 10.1016/j.clindermatol.2007.09.010
Guadagnini, R., Halamoda Kenzaoui, B., Walker, L., Pojana, G., Magdolenova, Z., Bilanicova, D., et al. (2013). Toxicity screenings of nanomaterials: challenges due to interference with assay processes and components of classic in vitro tests. Nanotoxicology 9, 13–24. doi: 10.3109/17435390.2013.829590
Gulson, B., Mccall, M., Korsch, M., Gomez, L., Casey, P., Taylor, A., et al. (2010). Small amounts of zinc from zinc oxide particles in sunscreens applied outdoors are absorbed through human skin. Toxicol. Sci. 118, 140–149. doi: 10.1093/toxsci/kfq243
Gulson, B., Mccall, M. J., Bowman, D. M., and Pinheiro, T. (2015). A review of critical factors for assessing the dermal absorption of metal oxide nanoparticles from sunscreens applied to humans and a research strategy to address current deficiencies. Arch. Toxicol. 89, 1909–1930. doi: 10.1007/s00204-015-1564-z
Hanawa, T. (2019). Titanium–tissue interface reaction and its control with surface treatment. Front. Bioeng. Biotechnol. 7:170. doi: 10.3389/fbioe.2019.00170
Higgins, J., and Green, S. (2011). Cochrane handbook for systematic reviews of interventions version 5.1.0 [updated March 2011]. Colloids Surf. B Biointerfaces. Available online at: www.handbook.cochrane.org
Holder, A. L., Goth-Goldstein, R., Lucas, D., and Koshland, B. K. (2012). Particle-induced artifacts in the MTT and LDH viability assays. Chem. Res. Toxicol. 25, 1885–1892. doi: 10.1021/tx3001708
Horie, M., Sugino, S., Kato, H., Tabei, Y., Nakamura, A., and Yoshida, Y. (2016). Does photocatalytic activity of TiO2 nanoparticles correspond to photo-cytotoxicity? cellular uptake of TiO2 nanoparticles is important in their photo-cytotoxicity. Toxicol. Mech. Methods 26, 284–294. doi: 10.1080/15376516.2016.1175530
International Organization for Standardization (2017). ISO/TS 20477:2017: Nanotechnologies — Standard terms and Their Definition for Cellulose Nanomaterial. International Organization for Standardization.
Jacobs, J. F., van de Poel, I., and Osseweijer, P. (2010). Sunscreens with titanium dioxide (TiO2) nano-particles: a societal experiment. Nanoethics 4, 103–113. doi: 10.1007/s11569-010-0090-y
Jin, C., Tang, Y., Yang, F. G., and Li, X. L. (2011). Cellular toxicity of TiO 2 nanoparticles in anatase and rutile crystal phase. Biol. Trace Elem. Res. 141, 3–15. doi: 10.1007/s12011-010-8707-0
Kato, S., Aoshima, H., Saitoh, Y., and Miwa, N. (2014). Fullerene-C60 derivatives prevent UV-irradiation/ TiO2-induced cytotoxicity on keratinocytes and 3D-skin tissues through antioxidant actions. J. Nanosci. Nanotechnol. 14, 3285–3291. doi: 10.1166/jnn.2014.8719
Kim, H., Choi, J., Lee, H., Park, J., Yoon, B.-I., Jin, S. M., et al. (2016). Skin corrosion and irritation test of nanoparticles using reconstructed three-dimensional human skin model, epiderm TM. Toxicol. Res. 32, 311–316. doi: 10.5487/TR.2016.32.4.311
Kongsong, P., Sikong, L., Niyomwas, S., and Rachpech, V. (2014). Photocatalytic antibacterial performance of glass fibers thin film coated with N-doped SnO2/TiO2. Sci. World J. 2014:869706. doi: 10.1155/2014/869706
Kroll, A., and Hendrik, M. (2012). Interference of engineered nanoparticles with in vitro toxicity assays. Arch. Toxicol. 86, 1123–1136. doi: 10.1007/s00204-012-0837-z
Kroll, A., Pillukat, M. H., Hahn, D., and Schnekenburger, J. (2009). Current in vitro methods in nanoparticle risk assessment : limitations and challenges. Eur. J. Pharm. Biopharm. 72, 370–377. doi: 10.1016/j.ejpb.2008.08.009
Lademann, J., Weigmann, H.-J., Rickmeyer, C., Barthelmes, H., Schaefer, H., Mueller, G., et al. (1999). Penetration of titanium dioxide microparticles in a sunscreen formulation into the horny layer and the follicular. Ski. Pharmacol. Appl. Ski. Physiol. 12, 247–256. doi: 10.1159/000066249
Lammel, T., and Sturve, J. (2018). NanoImpact assessment of titanium dioxide nanoparticle toxicity in the rainbow trout (Onchorynchus mykiss) liver and gill cell lines RTL-W1 and RTgill-W1 under particular consideration of nanoparticle stability and interference with fl uorometric assays. NanoImpact 11, 1–19. doi: 10.1016/j.impact.2018.01.001
Larese Filon, F., Crosera, M., Timeus, E., Adami, G., Bovenzi, M., Ponti, J., et al. (2013). Human skin penetration of cobalt nanoparticles through intact and damaged skin. Toxicol. Vitr. 27, 121–127. doi: 10.1016/j.tiv.2012.09.007
Lekki, J., Stachura, Z., Dabrós, W., Stachura, J., Menzel, F., Reinert, T., et al. (2007). On the follicular pathway of percutaneous uptake of nanoparticles: ion microscopy and autoradiography studies. Nucl. Instrum. Methods Phys. Res. 260, 174–177. doi: 10.1016/j.nimb.2007.02.021
Lin, L. L., Grice, J. E., Butler, M. K., Zvyagin, A. V., Becker, W., Robertson, T. A., et al. (2011). Time-correlated single photon counting for simultaneous monitoring of zinc oxide nanoparticles and NAD (P) H in intact and barrier-disrupted volunteer skin. Pharm. Res. 28, 2920–2930. doi: 10.1007/s11095-011-0515-5
Liu, W., Zhou, W., Liu, S., Zhang, C., Huang, S., Li, Y., et al. (2018). Electrical impedance performance of metal dry bioelectrode with different surface coatings. Sens. Actuators A Phys. 269, 515–523. doi: 10.1016/j.sna.2017.12.006
Louro, H., Saruga, A., Santos, J., and Silva, M. J. (2019). Biological impact of metal nanomaterials in relation to their physicochemical characteristics. Toxicol. Vitr. 56, 172–183. doi: 10.1016/j.tiv.2019.01.018
Lüderwald, S., Dackermann, V., Seitz, F., Adams, E., Feckler, A., Schilde, C., et al. (2019). Science of the total environment a blessing in disguise? Natural organic matter reduces the UV light-induced toxicity of nanoparticulate titanium dioxide. Sci. Total. Environ. 663, 518–526. doi: 10.1016/j.scitotenv.2019.01.282
Lupu, A. R., and Popescu, T. (2013). Toxicology in Vitro the noncellular reduction of MTT tetrazolium salt by TiO 2 nanoparticles and its implications for cytotoxicity assays. Toxicol Vitr. 27, 1445–1450. doi: 10.1016/j.tiv.2013.03.006
Madl, C. M., Heilshorn, S. C., and Blau, H. M. (2018). Review bioengineering strategies to accelerate stem cell therapeutics. Nature 557, 335–342. doi: 10.1038/s41586-018-0089-z
Martirosyan, A., and Schneider, Y. J. (2014). Engineered nanomaterials in food: Implications for food safety and consumer health. Int. J. Environ. Res. Public Health. 11, 5720–5750. doi: 10.3390/ijerph110605720
Mathes, S. H., Ruffner, H., and Graf-hausner, U. (2013). The use of skin models in drug development. Adv. Drug Deliv. Rev. 69, 81–102. doi: 10.1016/j.addr.2013.12.006
Mello, D. F., Trevisan, R., Rivera, N., Geitner, N. K., Di Giulio, R. T., Wiesner, M. R., et al. (2020). Caveats to the use of MTT, neutral red, hoechst and resazurin to measure silver nanoparticle cytotoxicity. Chem. Biol. Interact. 315:108868. doi: 10.1016/j.cbi.2019.108868
Miyani, V. A., and Hughes, M. F. (2016). Assessment of the in vitro dermal irritation potential of cerium, silver, and titanium nanoparticles in a human skin equivalent model. Cutan. Ocul. Toxicol. 36, 145–151. doi: 10.1080/15569527.2016.1211671
Moher, D., Liberati, A., Tetzlaff, J., and Altman, D. G. (2009). Preferred reporting items for systematic reviews and meta-analyses: the PRISMA statement. PLoS Med. 6:e1000097. doi: 10.1371/journal.pmed.1000097.
Monteiro-Riviere, N. A., Wiench, K., Landsiedel, R., Schulte, S., Inman, A. O., and Riviere, J. E. (2011). Safety evaluation of sunscreen formulations containing titanium dioxide and zinc oxide nanoparticles in UVB sunburned skin: an in vitro and in vivo study. Toxicol. Sci. 123, 264–280. doi: 10.1093/toxsci/kfr148
Morganti, P. (2010). Use and potential of nanotechnology in cosmetic dermatology. Clin. Cosmet. Investig. Dermatol. 3, 5–13. doi: 10.2147/CCID.S4506
Næss, E. M., Hofgaard, A., Skaug, V., Gulbrandsen, M., and Danielsen, T. E. (2015). Titanium dioxide nanoparticles in sunscreen penetrate the skin into viable layers of the epidermis : a clinical approach. Photodermatol. Photoimmunol. Photomed. 32, 48–51. doi: 10.1111/phpp.12217
Neupane, R., Boddu, S. H. S., Renukuntla, J., Babu, R. J., and Tiwari, A. K. (2020). Alternatives to biological skin in permeation studies: current trends and possibilities. Pharmaceutics 12:152. doi: 10.3390/pharmaceutics12020152
Nohynek, G. J., Lademann, J., Ribaud, C., and Roberts, M. S. (2007). Grey Goo on the skin? Nanotechnology, cosmetic and sunscreen safety. Crit. Rev. Toxicol. 37, 251–277. doi: 10.1080/10408440601177780
Oberdörster, G., Maynard, A., Donaldson, K., Castranova, V., Fitzpatrick, J., Ausman, K., et al. (2005). Principles for characterizing the potential human health effects from exposure to nanomaterials : elements of a screening strategy. Part. Fibre Toxicol. 2:8. doi: 10.1186/1743-8977-2-8
OECD (2014). Test No. 431: In Vitro Skin Corrosion: Reconstructed Human Epidermis (Rhe) Test Method. Paris: OECD Publishing. doi: 10.1787/9789264224193-en
OECD (2015). Test No. 404: Acute Dermal Irritation/Corrosion, OECD Guidelines for the Testing of Chemicals, Section 4. OECD Publishing. doi: 10.1787/9789264242678-en
OECD (2019). Test No. 439: In Vitro Skin Irritation: Reconstructed Human Epidermis Test Method, OECDGuidelines for the Testing of Chemicals, Section 4. Paris: OECD Publishing. doi: 10.1787/9789264242845-en
OHAT, N. (2015). National Toxicology Program (US Department of Health and Human Services). Handbook for Conducting a Literature-based Health Assessment Using OHAT Approach for Systemic Review and Evidence Integration. 1–98. Available online at: https://ntp.niehs.nih.gov/ntp/ohat/pubs/handbookjan2015_508.pdf (accessed June 18, 2019).
Ong, K. J., Maccormack, T. J., Clark, R. J., Ede, J. D., Ortega, V. A., Felix, L. C., et al. (2014). Widespread nanoparticle-assay interference : implications for nanotoxicity testing. PLoS ONE 9:e90650. doi: 10.1371/journal.pone.0090650
Organisation for Economic Co-operation and Development (2018). Evaluation of in vitro methods for human hazard assessment applied in the OECD Testing Programme for the Safety of Manufactured Nanomaterials. Organisation for Economic Co-operation and Development.
Osmond, M. J., and McCall, M. J. (2010). Zinc oxide nanoparticles in modern sunscreens: an analysis of potential exposure and hazard. Nanotoxicology. 4, 15–41. doi: 10.3109/17435390903502028
Owen, R., Reilly, G. C., and Kingdom, U. (2018). In vitro models for studying bone remodelling. Front. Bioeng. Biotechnol. 6:134. doi: 10.3389/fbioe.2018.00134
Park, Y.-H., Jeong, S. H., Yi, S. M., Choi, B. H., Kim, Y.-R., Kim, I.-K., et al. (2011). Analysis for the potential of polystyrene and TiO2 nanoparticles to induce skin irritation, phototoxicity, and sensitization. Toxicol. In Vitro 25, 1863–1869. doi: 10.1016/j.tiv.2011.05.022
Pelclova, D., Navratil, T., Kacerova, T., Zamostna, B., Fenclova, Z., Vlckova, S., et al. (2019). NanoTiO 2 sunscreen does not prevent systemic oxidative stress caused by UV radiation and a minor amount of NanoTiO 2 is absorbed in humans. Nanomaterials 9:888. doi: 10.3390/nano9060888
Potočnik, J. (2011). Commission recommendation of 18 october 2011 on the definition of nanomaterial (2011/696/EU). Off. J. Eur. Union 275, 38–40. Available online at: https://ec.europa.eu/research/industrial_technologies/pdf/policy/commission-recommendation-on-the-definition-of-nanomater-18102011_en.pdf.
Proquin, H., Rodríguez-ibarra, C., Moonen, C. G. J., Ortega, I. M. U., Bried,é, J. J, Kok, T. M., De, et al. (2016). Titanium dioxide food additive (E171) induces ROS formation and genotoxicity : contribution of micro and nano-sized fractions. Mutagenesis 32, 139–149. doi: 10.1093/mutage/gew051
Qiao, H., and Tang, T. (2018). Engineering 3D approaches to model the dynamic microenvironments of cancer bone metastasis. Bone Res. 6, 1–12. doi: 10.1038/s41413-018-0008-9
Rampaul, A., Parkin, I. P., and Cramer, L. P. (2007). Damaging and protective properties of inorganic components of sunscreens applied to cultured human skin cells. J. Photochem. Photobiol. A Chem. 191, 138–148. doi: 10.1016/j.jphotochem.2007.04.014
Ribeiro, A. R., Leite, P. E., Falagan-Lotsch, P., Benetti, F., Micheletti, C., Budtz, H. C., et al. (2017). Challenges on the toxicological predictions of engineered nanoparticles. NanoImpact 8, 59–72. doi: 10.1016/j.impact.2017.07.006
Salamanna, F., Contartese, D., Maglio, M., and Fini, M. (2016). A systematic review on in vitro 3D bone metastases models : a new horizon to recapitulate the native clinical scenario? Oncotarget 7:44803. doi: 10.18632/oncotarget.8394
Sanches, P. L., Souza, W., Gemini-Piperni, S., Rossie, A. L., Scapin, S., Midlej, V., et al. (2019). Rutile nano-bio-interactions mediate dissimilar intracellular destiny in human skin cells. Nanoscale Adv. 1, 2216–2228. doi: 10.1039/C9NA00078J
Saquib, Q., Al-Khedhairy, A. A., Siddiqui, M. A., Abou-Tarboush, F. M., Azam, A., and Musarrat, J. (2012). Titanium dioxide nanoparticles induced cytotoxicity, oxidative stress and DNA damage in human amnion epithelial (WISH) cells. Toxicol. Vitr. 26, 351–361. doi: 10.1016/j.tiv.2011.12.011
Sarmento, B., Andrade, F., Baptista, S., and Rodrigues, F. (2012). Cell-based in vitro models for predicting drug permeability. Expert Opin. Drug Metab. Toxicol. 8, 607–621. doi: 10.1517/17425255.2012.673586
Schäfer-Korting, M., Bock, U., Diembeck, W., Düsing, H. J., Gamer, A., Haltner-Ukomadu, E., et al. (2008). The use of reconstructed human epidermis for skin absorption testing: Results of the validation study. ATLA Altern. Lab Anim. 36, 161–187. doi: 10.1177/026119290803600207
Schardt, C., Adams, M. B., Owens, T., Keitz, S., and Fontelo, P. (2007). Utilization of the PICO framework to improve searching PubMed for clinical questions. BMC Med. Inform. Decis. Mak. 7:16. doi: 10.1186/1472-6947-7-16
Schneider, K., Schwarz, M., Burkholder, I., Kopp-Schneider, A., Edler, L., Kinsner-Ovaskainen, A., et al. (2009). “ToxRTool”, a new tool to assess the reliability of toxicological data. Toxicol. Lett. 189, 138–144. doi: 10.1016/j.toxlet.2009.05.013
Schneider, M., Stracke, F., Hansen, S., and Schaefer, U. F. (2009). Nanoparticles and their interactions with the dermal barrier. Dermatoendocrinology 1, 197–206. doi: 10.4161/derm.1.4.9501
Semenzin, E., Lanzellotto, E., Hristozov, D., Critto, A., Zabeo, A., Giubilato, E., et al. (2015). Species sensitivity weighted distribuition for ecological risk assessment of engineered nanomaterials: the nTiO2 case study. Environ. Toxicol. Chem. 34, 2644–2659. doi: 10.1002/etc.3103
Senzui, M., Tamura, T., Miura, K., Ikarashi, Y., Watanabe, Y., and Fujii, M. (2010). Study on penetration of titanium dioxide (TiO2) nanoparticles into intact and damaged skin in vitro. J. Toxicol. Sci. 35, 107–113. doi: 10.2131/jts.35.107
Sethi, D., Pal, A., Sakthivel, R., Pandey, S., Dash, T., Das, T., et al. (2014). Journal of photochemistry and photobiology B : biology water disinfection through photoactive modified titania. J. Photochem. Photobiol. B Biol. 130, 310–317. doi: 10.1016/j.jphotobiol.2013.12.003
Shakeel, M., Jabeen, F., Shabbir, S., Asghar, M. S., Khan, M. S., and Chaudhry, A. S. (2016). Toxicity of nano-titanium dioxide (TiO 2 -NP) through various routes of exposure : a review. Biol. Trace Elem. Res. 172, 1–36. doi: 10.1007/s12011-015-0550-x
Sharifi, S., Behzadi, S., Laurent, S., and Forrest, M. L. (2012). Toxicity of nanomaterials. Chem. Soc. Rev. 41, 2323–2343. doi: 10.1039/C1CS15188F
Shetti, N. P., Bukkitgar, S. D., Reddy, K. R., Reddy, C. V., and Aminabhavi, T. M. (2019). Nanostructured titanium oxide hybrids-based electrochemical biosensors for healthcare applications. Colloids Surf. B Biointerfaces 178, 385–394. doi: 10.1016/j.colsurfb.2019.03.013
Shi, H., Magaye, R., Castranova, V., and Zhao, J. (2013). Titanium dioxide nanoparticles: a review of current toxicological data. Part. Fibre Toxicol. 10:15. doi: 10.1186/1743-8977-10-15
Shukla, R. K., Kumar, A., Vallabani, N. V. S., Pandey, A. K., and Dhawan, A. (2014). Titanium dioxide nanoparticle-induced oxidative stress triggers DNA damage and hepatic injury in mice. Nanomedicine. 9, 1423–1434. doi: 10.2217/nnm.13.100
Singh, A. V., Laux, P., Luch, A., Sudrik, C., Wild, A., Santamauro, G., et al. (2019). Review of emerging concepts in nanotoxicology: opportunities and challenges for safer nanomaterial design. Toxicol. Mech. Methods. 29, 378–387. doi: 10.1080/15376516.2019.1566425
Smijs, T. G., and Pavel, S. (2011). Titanium dioxide and zinc oxide nanoparticles in sunscreens: focus on their safety and effectiveness. Nanotechnol. Sci. Appl. 4, 95–112. doi: 10.2147/NSA.S19419
Tan, M., Commens, G. A., Burnett, L., and Snitch, P. J. (1996). A pilot study on the percutaneous absorption of microfine titanium dioxide from sunscreens. Aust. J. Dermatol. 37, 185–187. doi: 10.1111/j.1440-0960.1996.tb01050.x
Tang, Y., Cai, R., Cao, D., Kong, X., and Lu, Y. (2018). Photocatalytic production of hydroxyl radicals by commercial TiO2 nanoparticles and phototoxic hazard identification. Toxicology 406–407, 1–8. doi: 10.1016/j.tox.2018.05.010
Touloumes, G. J., Ardoña, H. A. M., Evan, K., Zimmerman, J. F., Chantre, C. O., Demokritou, P., et al. (2020). Mapping 2D- and 3D-distributions of metal/metal oxide nanoparticles within cleared human ex vivo skin tissues. Nanoimpact 17:100208. doi: 10.1016/j.impact.2020.100208
Tucci, P., Porta, G., Agostini, M., Dinsdale, D., Iavicoli, I., Cain, K., et al. (2013). Metabolic effects of TIO2 nanoparticles, a common component of sunscreens and cosmetics, on human keratinocytes. Cell Death Dis. 4:e549. doi: 10.1038/cddis.2013.76
Vernetti, L., Gough, A., Baetz, N., Blutt, S., Broughman, J. R., Brown, J. A., et al. (2017). Corrigendum: functional coupling of human microphysiology systems: intestine, liver, kidney proximal tubule, blood-brain barrier and skeletal muscle. Sci. Rep. 7:44517. doi: 10.1038/srep44517
Wang, S. Q., and Tooley, I. R. (2011). Photoprotection in the era of nanotechnology. YSDER Semin. Cutan. Med. Surg. 30, 210–213. doi: 10.1016/j.sder.2011.07.006
Wang, Y., Cui, H., Zhou, J., and Li, F. (2014). Cytotoxicity, DNA damage and apoptosis induced by titanium dioxide nanoparticles in human non-small cell lung cancer A549 cells. Environ. Sci. Pollut. Res. 22, 5519–5530. doi: 10.1007/s11356-014-3717-7
Wiesenthal, A., Hunter, L., Wang, S., Wickliffe, J., and Wilkerson, M. (2011). Nanoparticles: Small and mighty. Int. J. Dermatol. 50, 247–254. doi: 10.1111/j.1365-4632.2010.04815.x
Wolf, R., Wolf, D., Morganti, P., and Ruocco, V. (2001). Sunscreens. Elsevier Sci. Inc. All Rights Reserv. 19, 452–459. doi: 10.1016/S0738-081X(01)00190-0
Wu, J., Liu, W., Xue, C., Zhou, S., Lan, F., Bi, L., et al. (2009). Toxicity and penetration of TiO2 nanoparticles in hairless mice and porcine skin after subchronic dermal exposure. Toxicol. Lett. 191, 1–8. doi: 10.1016/j.toxlet.2009.05.020
Yu, B., Ai, K., and Lu, L. (2020). Dual-protective nano-sunscreen enables high-efficient elimination of the self-derived hazards. Appl. Mater. Today 18:100493. doi: 10.1016/j.apmt.2019.100493
Keywords: titanium dioxide, nanoparticles, 3D skin model, alternative method, toxicity
Citation: Sanches PL, Geaquinto LRO, Cruz R, Schuck DC, Lorencini M, Granjeiro JM and Ribeiro ARL (2020) Toxicity Evaluation of TiO2 Nanoparticles on the 3D Skin Model: A Systematic Review. Front. Bioeng. Biotechnol. 8:575. doi: 10.3389/fbioe.2020.00575
Received: 23 December 2019; Accepted: 12 May 2020;
Published: 10 June 2020.
Edited by:
Jianbo Jia, Guangzhou University, ChinaReviewed by:
Xiaofei Zhou, Hebei Agricultural University, ChinaHainan Sun, Shandong University, China
Copyright © 2020 Sanches, Geaquinto, Cruz, Schuck, Lorencini, Granjeiro and Ribeiro. This is an open-access article distributed under the terms of the Creative Commons Attribution License (CC BY). The use, distribution or reproduction in other forums is permitted, provided the original author(s) and the copyright owner(s) are credited and that the original publication in this journal is cited, in accordance with accepted academic practice. No use, distribution or reproduction is permitted which does not comply with these terms.
*Correspondence: José Mauro Granjeiro, am1ncmFuamVpcm8mI3gwMDA0MDtnbWFpbC5jb20=; Ana Rosa Lopes Ribeiro, YW5hbG9wZXMwNzgxJiN4MDAwNDA7Z21haWwuY29t
†Present address: Ana Rosa Lopes Ribeiro, 3B's Research Group, Research Institute on Biomaterials, Biodegradables and Biomimetics, Headquarters of the European Institute of Excellence on Tissue Engineering and Regenerative Medicine, University of Minho, Guimarães, Portugal