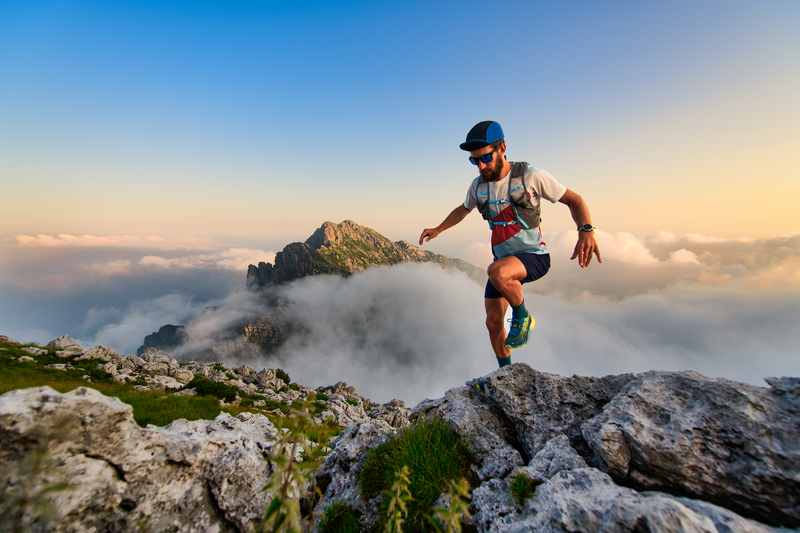
94% of researchers rate our articles as excellent or good
Learn more about the work of our research integrity team to safeguard the quality of each article we publish.
Find out more
REVIEW article
Front. Bioeng. Biotechnol. , 29 May 2020
Sec. Biomaterials
Volume 8 - 2020 | https://doi.org/10.3389/fbioe.2020.00519
This article is part of the Research Topic Innovative In Vitro Models for Pulmonary Physiology and Drug Delivery in Health and Disease View all 24 articles
Recent developments in epidemiology have confirmed that airborne particulates are directly associated with respiratory pathology and mortality. Although clinical studies have yielded evidence of the effects of many types of fine particulates on human health, it still does not have a complete understanding of how physiological reactions are caused nor to the changes and damages associated with cellular and molecular mechanisms. Currently, most health assessment studies of particulate matter (PM) are conducted through cell culture or animal experiments. The results of such experiments often do not correlate with clinical findings or actual human reactions, and they also cause difficulty when investigating the causes of air pollution and associated human health hazards, the analysis of biomarkers, and the development of future pollution control strategies. Microfluidic-based cell culture technology has considerable potential to expand the capabilities of conventional cell culture by providing high-precision measurement, considerably increasing the potential for the parallelization of cellular assays, ensuring inexpensive automation, and improving the response of the overall cell culture in a more physiologically relevant context. This review paper focuses on integrating the important respiratory health problems caused by air pollution today, as well as the development and application of biomimetic organ-on-a-chip technology. This more precise experimental model is expected to accelerate studies elucidating the effect of PM on the human body and to reveal new opportunities for breakthroughs in disease research and drug development.
With the development of epidemiology in recent years, scientists have confirmed that airborne particulate matter (PM) is directly associated with respiratory pathology and mortality (Kim et al., 2018; Khaniabadi et al., 2019). For every 10 μg/m3 increase in PM10 concentration, respiratory system-related mortality increases by 0.58% (Analitis et al., 2006), and for every 10 μg/m3 increase in PM2.5, the incidence of respiratory-system related diseases increases by 2.07% (Zanobetti et al., 2009). These studies indicate that impaired lung function also increases the incidence and mortality of cardiopulmonary disease (Zanobetti et al., 2009; de Oliveira et al., 2012). In addition to the problem of increased risk of respiratory disease caused by compromised lung function, PM may also increase the incidence of lung cancer (Raaschou-Nielsen et al., 2016). In a large-scale study conducted in the United States with a sample of 188,699 non-smokers, each 10 μg/m3 increase in PM2.5 concentrations increased lung cancer-related mortality by 15–27% (Turner et al., 2011). Although PM is a global concern, severe air pollution episodes are often associated with industrialization, and urbanization. As China's rapid economic growth, several recent studies paid attentions to frequent air pollution episodes (Li et al., 2016; Lin et al., 2018). The air quality statistics report of Beijing from 2013 to 2015 shows that the 2 year average PM2.5 concentrations from 69 to 89 μg/m3 and the daily average concentrations ranged from 3 to 437 μg/m3 (Batterman et al., 2016). These studies have shown that it is imperative to address the harmful effects of PM on the human body, in addition to traditionally known respiratory diseases such as asthma and chronic obstructive pulmonary disease (COPD) (Hopke et al., 2019). The incidence of cardiopulmonary disease and lung cancer are also associated with a high mortality rate (Hamanaka and Mutlu, 2018). Therefore, it is essential to quickly and accurately elucidate the effects of PM on the human body, determine the causes of diseases, and formulate response strategies.
PM is one of the most important components of air pollution that affects human health and disease. It is classified based on the relative size, which is defined in terms of aerodynamic equivalent diameter (AED), not directly by the diameter of their actual particles. In other words, the size of the actual particles is converted into an equivalent diameter having the same aerodynamic properties (Raabe, 1976). PM can be divided into three AED levels based on the deposition and penetration ability of the particles in the human respiratory system: ≤ 10 μm, ≤ 2.5 μm, and ≤ 0.1 μm (PM10, PM2.5, and PM0.1, respectively) (Figure 1A). Particles with AED diameters >10 μm have a relatively small half-life in suspension and are mostly filtered by the nasal and upper respiratory tract, so researchers have classified PM >10 μm into three categories: (i) coarse PM (PM2.5−10), (ii) fine PM (PM0.1−2.5), and (iii) ultrafine PM (PM0.1) (Anderson et al., 2012).
Figure 1. The transport and health effects of PM in human lung and cardiovascular. (A) Classification of PM size by aerodynamic equivalent diameter (AED). (B) PM deposition curves in the extrathoracic, bronchial, and alveolar regions for adult humans. Reproduced with permission from Tsuda et al. (2013). (C) The schematization of the immune responses and inflammation in lung cells by inhaled PM. Reproduced with permission from Nemmar et al. (2013). (D) There are three main hypotheses that PM can cause biological pathways for cardiovascular impairment. Reproduced with permission from Ngoc et al. (2018).
Although the effects of PM exposure depend on individual physical characteristics, such as the pattern and rate of breathing, weight, and age, the size of the particles has been identified as a direct cause of health problems (Brown et al., 2013). Generally, the smaller the particles, the faster they penetrate and deposit in the deeper levels of the respiratory system. In nasal breathing, cilia and mucous membranes are very effective in filtering most particles larger than 10 μm in diameter. Because of the rapid deposition of PM with larger particle size, they tend to remain in the trachea or bronchi (upper respiratory tract). They initially accumulate in the nose and throat, and the body will eliminate these invasive PM through some reactive processes such as sneezing and coughing. Up to now, particles <10 μm in diameter are considered to have the greatest impact on human health, and because of their high penetration ability, they can evade the protective mechanisms of the upper respiratory tract and enter the alveoli deep in the lungs (Löndahl et al., 2006; Kim et al., 2015). Computer simulations have shown that particles with diameters between 1 and 10 μm are primarily deposited in the nasopharyngeal and laryngeal of upper airway regions, and particles with diameters between 1 and 100 nm are deposited in the lower bronchial and alveolar region, where gas exchange occurs (Tsuda et al., 2013) (Figure 1B). However, particles smaller than 1 μm tend to behave like gas molecules, so it is extremely easy for them to penetrate into the alveoli and affect gas exchange in the lungs and even penetrate the barrier of the lungs and enter the circulatory system, further migrating to other cells, tissues, or circulatory and metabolic systems and leading to serious health problems (Table 1).
Currently, there have been many studies on the biological mechanism and effects of PM on the respiratory system (Xing et al., 2016), which primarily involve the following aspects: (1) Functional injury caused by free radical peroxidation. Studies have shown that free radicals, metals, and organic components in PM2.5 can induce the free radical formation, cause oxidation of lung cells, and also cause the production of reactive oxygen species (ROS), resulting in DNA damage and cell death (Donaldson et al., 1996; Lodovici and Bigagli, 2011). (2) Imbalance of intracellular calcium regulation (calcium homeostasis). Calcium ion is a physiological index of cell function regulation. However, free radical and ROS reactions induced by PM2.5 can cause abnormal intracellular calcium concentrations, which lead to apoptosis and necrosis (Li et al., 2015; Al Hanai et al., 2019). (3) Inflammatory injury. This is also the most extensive part of current studies. PM2.5 can cause significant immune responses and inflammation in lung cells (Nadeau et al., 2010; He et al., 2017). These inflammatory responses to Th1 and Th2 triggered by Toll-like receptor (TLRs) pathways can lead to activation of neutrophils, T cells, and alveolar macrophages, which is also associated with asthma and COPD (Nemmar et al., 2013) (Figure 1C). Although studies on PM and its biological mechanisms are currently ongoing, an important future research direction is to elucidate the inflammatory response to PM in the lungs.
With the development of epidemiology in recent years, scientists have validated the effects of long-term PM exposure on the respiratory system. PM can penetrate deep into the trachea and bronchi and can even deposit in alveolar tissue. Hydroxyl radicals (·OH) produced from reactive oxygen species (ROS) through activated metals are the main factors that cause oxidative damage to DNA. If damaged DNA is not repaired in a timely matter, it can induce cancer or lead to other irreversible damage (Valavanidis et al., 2005). Environmental exposure to this fine PM not only causes respiratory disease but also affects heart rate, blood pressure, vascular tone, blood coagulation, and formation of atherosclerotic lesions (Suwa et al., 2002; Bennett et al., 2018; Huang et al., 2018). In addition, there is a positive correlation between fine PM and the incidence and mortality of cardiopulmonary disease. The World Health Organization (WHO) reported that 3.7 million deaths in 2012 were due to air pollution, which accounted for 6.7% of deaths worldwide. Of these, 16% were deaths due to lung cancer, 11% were due to chronic obstructive pulmonary disease and associated diseases, 29% were due to heart disease and stroke, and about 13% were due to respiratory infections. In addition, fine PM in the air that is inhaled into the lungs can translocate to the bloodstream and be transported to the blood vessels and the heart, which can induce arrhythmia and reduce myocardial contractility and coronary blood flow (Nemmar et al., 2003). The possible mechanisms for cardiopulmonary risk following inhalation of fine PM into the lungs can be roughly classified into three groups: (1) Stimulating the production of inflammatory factors: inducing the secretion of inflammatory factors such as cytokines, activated immune cells, platelets, and endothelin, from basal cells in the lungs (Kido et al., 2011; Tsai et al., 2012). (2) Translocation of PM: toxic effects caused by translocation of PM or its components to the circulatory system (Nemmar et al., 2002). (3) Neuroendocrine disorders: the balance of the autonomic nervous system or heart rate is affected by the binding of PM to receptors located on the lungs or nerves (Ngoc et al., 2018; Snow et al., 2018) (Figure 1D).
Du et al. summarized recent research results on short- and long-term exposure to PM2.5 (Du et al., 2016). The results show that every 10 μg/m3 increase in short-term PM2.5 exposure concentration increases overall mortality and cardiovascular-related mortality by 0.4–1.0%, while every 10 μg/m3 increase in long-term exposure increases overall mortality by 10% and cardiovascular-related mortality by 3–76%. With respect to various cardiovascular diseases, PM2.5 has the greatest impact on coronary heart disease, moderate impact on heart failure and stroke, and the smallest impact on peripheral vascular diseases and arrhythmia. Because these risk factors not only cause a sharp increase in the risk of cardiovascular disease, and the metabolic syndrome secondary to it is closely associated with some of the most significant causes of death worldwide, including increased risk of Alzheimer's disease, Parkinson disease, dementia, and stroke (Fu et al., 2019). Although the true pathogenic mechanism is currently unknown and the impairment of cardiopulmonary function due to fine PM is due to a complex series of effects, the establishment of an in vitro model that represents the human body in a large number of studies is an urgent need. It is expected that establishing an in vitro model of cardiopulmonary function will yield new possibilities and opportunities for understanding the hazards and influencing mechanisms associated with environmental engineering and human health.
Epidemiological and clinical studies have linked exposure to fine PM to adverse health outcomes, which may also be associated with increased mortality and morbidity in various cardiopulmonary diseases. Despite much evidence of the effects of PM on human health, the causes of physiological responses and the changes and damage to cellular and molecular mechanisms have not yet been fully explained. There are currently two main methods for elucidating the mechanisms of PM toxicity (Fröhlich and Salar-Behzadi, 2014; Yang et al., 2017) (Figure 2A). One is the use of in vivo animal models to evaluate the effects of fine PM on the respiratory and cardiovascular systems (Figure 2B). The other type is in vitro cell experiment models (Figure 2C); the use of various in vitro models has proven valuable for studying the molecular and cellular mechanisms behind different physiological effects more deeply.
Figure 2. Two main methods for elucidating the mechanisms of PM toxicity. (A) In vitro and in vivo toxicity assessment of PM. Reproduced with permission from Yang et al. (2017). (B) In vitro animal experiments exposed to aerosols mainly include nose exposure, intratracheal instillation, and oropharyngeal aspiration. Reproduced with permission from Fröhlich and Salar-Behzadi (2014). (C) Air–liquid interface (ALI) in vitro models for investigating respiratory research.
Studies using animal models have demonstrated the effects of fine PM exposure on different organs and the incidence of different diseases. With respect to acute reactions, most studies have focused on inflammatory responses, and relatively few researchers have investigated specific responses to disease (Hong et al., 2016; Wang H. et al., 2017). Conversely, with respect to chronic reactions, a large number of disease-related findings have been reported, including DNA damage, lung parenchyma damage, pulmonary fibrosis, and granuloma formation (Xu et al., 2013; Shadie et al., 2014). Meanwhile, because in vitro models have demonstrated that cell experiments are the most suitable model for investigating mechanisms of toxicity, such as initiation events for inflammatory effects or genotoxicity, these data cannot be explained because of genetic differences in animal experiments (Wang J. et al., 2017). In view of this, the genotoxicity and biological indicators linking disease and cancer have been clearly recognized in particular, but this is still a challenge in other applications, which also includes further elucidation of the mechanism of fine PM toxicity in humans (Vawda et al., 2013).
Although current knowledge does not fully understand the health effects of PM exposure, studies over the past decade have suggested the potential cytotoxicity. It has been observed in cell studies that PM stimulation has caused cell viability decline, cell death, ultrastructural disruptions, genetic toxicity (mutagenicity and DNA damage), and oxidative stress (Peixoto et al., 2017). These mechanisms involved in the inflammatory response, including the up-regulation of cytokines downstream of the caspase cascade and the kinase pathway, the up-regulation of metal-redox sensitive transcription factors NF-κβ and AP-1 (Øvrevik et al., 2015). In addition, the PM also increases inflammatory mediator-related gene expression and protein secretion, such as TNF-α, IL-1β, IL-8, IL-6, and MCP-1 (Fuentes-Mattei et al., 2010; Ryu et al., 2019). On the other hand, similar reactions have been observed in animal experiments, showing DNA damage, oxidative stress and inflammatory mediator-related protein secretion and recruitment of inflammatory cells in many organs (de Oliveira et al., 2018). In addition, many studies have shown association between exposure to PM and chronic diseases. These adverse health effects include asthma, chronic obstructive pulmonary disease (COPD), atherosclerosis, diabetes and allergic sensitization (Guo et al., 2018; Li et al., 2018).
Mammalian cell culture studies are often used as the first step in toxicity evaluation, cell-based studies are still greatly limited with respect to the complex structures of physiological mechanisms in humans, and it is impossible to simulate the complex conditions and the interrelated physiological information of the entire organism. Animal experiments play an important role in PM research, where they allow in vivo toxicological testing by exposing animals to various PM environments via the oral and dermal routes. Although animals can inhale PM and develop comprehensive systemic outcomes, there is often a large difference between mechanistic and genetic indicator data and clinical outcomes. This is primarily due to differences among species and their physiological functions, such as differences in respiratory rate between experimental mice and humans (Curbani et al., 2019), as well as the problems of genetics, low throughput, high cost, and ethical concerns.
As yet, there is currently no ideal experimental model for study the toxicity of fine particulate matter since both in vitro and in vivo models have limitations. Notably, the interpretation of chronic toxicity studies is relatively lacking of information, which requires consideration of whether the information obtained from animal studies is similar to human responses. This issue may be expected to be overcome through the advancement of biotechnology and biomedical engineering technologies, thereby obtaining a useful in vitro model that allow long-term cultivation of functional responses that express the human organ. In addition, due to the diversity and regional differences of PM composition, how to systematically study the toxicology of PM on the human body (including single components and the interaction between components) is also the focus of future research (Jia et al., 2017; Park et al., 2018).
With respect to the effects of PM exposure on the human body, many studies have demonstrated the relationship between PM and health risk, but further understanding of the mechanisms of human toxicity is still lacking. The problems faced are derived from the major differences in genes and structures between the species used in animal experiments and humans. Although biomimetic technology experiments are the most appropriate model for investigating mechanisms of toxicity, only a complete description and definition of genotoxicity and indicators between disease and cancer have been made at present, and other aspects are still being researched. In this field, improving the representativeness of in vitro experiments and strengthening the reference value of data has become important issues in research. This topic has been discussed by other authors (Vanderburgh et al., 2017; Ahadian et al., 2018; Costa and Ahluwalia, 2019). Developments and progress in biomimetic technology will bring unlimited potential for breaking through the research bottlenecks faced in this field.
To resolve the great differences between animal experiments and clinical trials, techniques have been developed in recent years for construction of organs-on-chips, with the goal of replacing animal experiments and achieving more accurate and reliable preclinical data (Alépée et al., 2014; Zhang et al., 2017, 2018) (Figure 3A). Currently, organ-on-a-chip development mostly relies on materials with high biocompatibility for construction of a 3D microenvironment suitable for cell growth so that cells can establish cell-to-cell interactions that are not possible in most 2D cell culture environments, to observe the phenomena of simulated organs more accurately. In particular, this new organ-on-a-chip models provide unlimited potential to replicate critical tissue-tissue responses by reconstructing dynamic physiological forces, cellular microenvironments, and 3D structures of human organ. The development of organ-on-chips and in-depth descriptions have been heatedly discussed in other reviews (Rothbauer et al., 2018; Nawroth et al., 2019) (Figure 3B). In addition, when combined with the “induced pluripotent stem cell (iPSCs)” technique in somatic cells, it is possible to successfully differentiate individualized target tissues of interest without traumatizing the organs. Researchers have successfully integrated various iPSC-derived cells with organ-on-chips, such as blood vessels-on-chip, a blood–brain barrier-on-chip and heart-on-chip systems. The different methods for creating organ-on-chips using stem cells also have been described in depth by other groups (Geraili et al., 2018; Jodat et al., 2018; Cochrane et al., 2019).
Figure 3. Organs-on-chips technology for tissue model development. (A) Organs-on-chips platform provides an in vitro model of various organs. Reproduced with permission from Zhang et al. (2017). (B) Comparison of experimental strategies for current in vitro, in vivo, and Organs-on-chips models. Adapted with permission from Nawroth et al. (2019). (C) A lung-on-a-chip microdevice reproduce human physiological respiratory movements. Reproduced with permission from Huh et al. (2010). (D) Construction of a lung-on-a-chip with tissue/organ-level physical microstructure and microenvironment. Reproduced with permission from Jain et al. (2018).
Although different organs-on-chips have their own requirements, applications associated with the respiratory tract are always very strong. For example, when the lungs are infected by fine PM, bacteria, or viruses, white blood cells accumulate, and the mucus produced block the airway. These processes are difficult to observe in animals and further highlight the importance of developing lung-on-a-chip technology. The Wyss Institute at Harvard University has been a worldwide pioneer in the development of in vitro organ-on-a-chip, and the lung-on-a-chip they developed was the first in the world (Huh et al., 2010) (Figures 3C,D). It is entirely based on polydimethylsiloxane (PDMS) material, with an upper and a lower layer of channels separated by a porous membrane coated with extracellular matrix. In its internal structure, the upper layer consists of alveolar epithelial cells that allow gases to pass through, and the lower layer consists of microvascular epithelial cells that allow white blood cells to pass through, thus simulating lung function. In 2016, Benam et al. used this technology to test smoking and non-smoking conditions, and confirmed that using the lung-on-a-chip yielded experimental results that were closer to clinical physiological and inflammatory reactions compared with those from animal experiments, and previously undiscovered biomarkers that were even more accurate were found and analyzed (Benam et al., 2016a). At the same time, other teams have developed lung chip models with different design structures and physiological responses (Fishler et al., 2015; Fishler and Sznitman, 2016; Humayun et al., 2018; Stucki et al., 2018; Khalid et al., 2020). The lung-on-a-chip have been developed to demonstrate their importance in drug development and disease models, but still have several practical challenges must be overcome if such devices are to be used in toxicology research and application (Low and Tagle, 2017; Wu et al., 2020). The aim of overcoming these challenges is to improve the usability of these devices and to simulate metabolism in the human body more accurately.
In addition, evidence from animal studies has shown that nanoparticles can cross the alveolar-capillary barrier and subsequently deposit in extrapulmonary organs such as the vasculature and heart (Choi et al., 2010). Using specific organ chips, such as heart-on-a-chip to investigate the toxicity of PM may also have great potential value. The heart-on-a-chip is mainly used to study electrical stimulation (Xiao et al., 2014), cardiac electrophysiology (Sidorov et al., 2017) and disease models (Wang et al., 2014). Marsano et al. recently established heart-on-a-chip platform integrates mechanical stress and 3D matrix microenvironment, showing better differentiation and electromechanical coupling of the iPSC-derived cardiomyocyte (Marsano et al., 2016). Liu et al. demonstrated the latest bioelectronic heart-on-a-chip model, which can regulate the concentration of oxygen through the microfluidic channel, and integrated bioelectronic devices to successfully monitor the cardiac electrophysiology responses to acute hypoxia (Liu et al., 2020). These examples fully demonstrate that heart-on-a-chip may provide greater ability to recapitulation the cellular microenvironment and tissue function. In the future, it is expected that suitable heart-on-a-chip models can be selected to investigate the human toxicity investigation of PM deposition, penetration and metabolism, but it is necessary to consider whether to choose particles with corresponding size, composition and complex for stimulation or exposure assessment. Of particular interest are the mechanisms of PM-mediated toxicity on the systemic health effects. In recent years, with the gradual advancement of multi-organ chips technology, it is expected to provide more clues to accelerate the clarification of the human toxicity of PM deposition, penetration and metabolism (Yuancheng et al., 2018; Carvalho et al., 2019), especially for the specific examples of lung- heart- on chip model, which could be used to investigate the systemic toxicology of PM into the human body.
It is worth mentioning that the organ-on-a-chip not only shows the application value in research, but also the establishment of related companies has started to appear in the past 5 years (Mastrangeli et al., 2019), such as TissUse GmbH, Emulate, Inc., MIMETAS Inc., Nortis, Inc., AlveoliX AG., Hesperos Inc. In addition, the U.S. Food and Drug Administration (FDA) announced in April 2017 that it had signed a multi-year cooperation agreement with Emulate Inc. (spinoff from the Wyss Institute for Biologically Inspired Engineering at Harvard University), and will begin a series of trials using organ-on-a-chip technology to develop a testing platform for toxicological safety assessment (Isoherranen et al., 2019). These results demonstrate the potential of applying organ-on-a-chip systems to human health assessments. In the future, the organ-on-a-chip technology is able to integrate stem cell technology, microenvironment and personalization parameters (e.g., breathing pattern, heart rate, substance abuse, etc.) to allow the construction of models of different genders, regions, ages, and diseases to minute minor physiological differences, thereby promoting the development of precision health (van den Berg et al., 2019).
Despite the progress made with organ-on-a-chip models, there remains a question that the organ-level functional replication is limited by the source of cells. In the case of pulmonary alveolar model, the aspect of long-term culture of primary human alveolar type I and type II epithelial cells is particularly challenging limitation (Shiraishi et al., 2019; Weiner et al., 2019). Therefore, the organ-on-a-chip technology faces limited availability and the inability to expand primary cells, requiring the establishment of cell cultures directly from donors and patients, which will increase the cost of experiments and the difficulty of popularizing the technology. On the other hand, the most organ-on-a-chip are made of PDMS due to their high biocompatibility, oxygen permeability, and transparency. The PDMS chip devices can directly match conventional cell culture incubators and biological microscopes. However, a large amount of protein molecules will be adsorbed on the surface of PDMS (Wong and Ho, 2009; Gokaltun et al., 2017), which results in that the supplement or stimulating substance of the cell culture cannot fully interact with the cells. To avoid adsorption of non-specific proteins, some teams have used polylactic acid (PLA) (Ongaro et al., 2020), poly (methyl methacrylate) (PMMA) (Nguyen et al., 2019), polystyrene (PS) (Lee et al., 2018), and polycarbonate (PC) (Henry et al., 2017), and more advantages and limitations of PDMS materials also have been introduced in detail by other teams (Halldorsson et al., 2015; Gokaltun et al., 2017).
With the development of organs-on-chips in the past decade, the establishment of single types of organ-on-a-chip or the development of disease models on a chip has gradually matured. Although the organ-on-chips have seen great progress, it is not enough to rely on a simulation single organ model for a comprehensive understanding due to the highly complex interactions between human organs. In 2004, Dr. Shuler and colleagues first proposed the concept of reproducing human physiological functions in chip devices (Sin et al., 2004). The increasing demand for in vitro models, chips integrating multiple organs have become a major topic in recent years, and they also represent a major step forward in organ-on-a-chip technology (Figure 4A). Currently, chips capable of representing multiple organs in an integrated manner and fully and accurately simulating human tissue are still being developed (Skardal et al., 2017; Oleaga et al., 2018; Boos et al., 2019; Sung et al., 2019; Zhao et al., 2019). For example, a new model for physiological pharmacokinetics (PKs) and pharmacodynamics (PDs) has successfully predicted the clinical patient data of cisplatin PDs. This model is linked through fluidically coupled vascularized organ chips to investigate PK and PD parameters of oral and injectable drugs (Herland et al., 2020). It is worth noting that the experiments of this model have reached an automated system through robotic fluidic coupling of multiple organ chips, and maintained the long-term culture of organ-specific functions for 3 weeks (Novak et al., 2020). The automated multi-organ chip system integrated with high-throughput screening has the potential to improve the prediction of drugs (or other foreign substances) absorption, distribution, metabolism, excretion and toxicity for clinical trials.
Figure 4. Integrate multi-organ chip platforms to create complex interactions between human organs. (A) The design concept of the human body chip. One of the most promising in vitro system for replicating the systemic responses of human body. Reproduced with permission from Huh et al. (2011). (B) Four-organs-on-a-chip system employed intestine, liver, skin, and kidney tissue that proportionately simulated the physiological environment of the human body. Reproduced with permission from Maschmeyer et al. (2015).
As another example, the device in a recent study mainly integrates four tissues—liver, heart, muscle, and neurons (Oleaga et al., 2016). It is composed of the liver (which serves to process drug metabolites and drug processes drugs or prodrugs), heart (which is the most important organ in the human body), skeletal muscle (which is responsible for glucose storage levels in the body), and neurons (which represent a particularly sensitive cell system). After culturing this system in a continuous flow environment for 14 days, its feasibility and functionality were demonstrated, and because the cells used in the system were primary cells and cells derived from iPSCs, they exhibited the exchange of metabolites and signaling molecules. In addition, by measuring heart rate, muscle contractility, neuroelectrophysiology, and production of liver albumin and urea, it served as an accurate model for predicting toxicity in multiple human organs. In another study, Maschmeyer et al. integrated pre-formed bowel and skin models into a hepatic spheroid and renal epithelial barrier tissue model, establishing a microchannel system that could support the functions of four types of organs in a co-culture over a long period of 1 month (Maschmeyer et al., 2015) (Figure 4B). In addition, this four-organs-on-a-chip system employed a structure that more proportionately simulated the physiological fluid and tissue environment of the human body. It simulated drug absorption and metabolism in the small intestine, metabolism by the liver, and excretion by the kidneys, which are all key factors that determine the efficacy and safety of drug treatments. These systems allow us to further understand metabolic and genetic analyses and provide an alternative to systemic toxicity testing. In addition, these examples demonstrate that integrated multi-organ chips are an important part in the ability to simulate complex reactions and interactions between tissues, whether in drug testing, toxicological screening, or construction of organ-on-a-chip models.
Therefore, integrating multi-organ chips are expected to replace the inadequacies of traditional in vitro models, promoting studies of the effects of air pollution on the body and the early development of drugs, as these devices are designed to mimic the physiological structure of internal organs and interactions with soluble metabolites, thereby achieving in vitro the interactive effects between organs. However, current multi-organ chip models are mainly used for systemic processes of oral and injectable drugs, but lacks models for PM inhalation. In a recent human inhalation study, Miller et al. investigated the transport behavior of gold nanoparticle inhaled into the lung (Miller et al., 2017). The results showed that the blood and urine of the volunteers still found gold nanoparticle after 3 months of exposure, indicating systemic retention and delayed urinary excretion. This study clearly understands the ability of inhaled nanoparticles to penetrate lung tissue, but investigating the interactions between human organs, especially for the cardiopulmonary system remains a challenge. Based on the most direct impact of PM on cardiopulmonary function, in the future, it is urgent to form an integrated platform by connecting the organ chips of the lung and heart in the future. Even PM gas can be exposed to such a platform for discussion. It is hoped that the cardiopulmonary function model established in vitro can be used to obtain new possibilities and opportunities for PM analysis, so that it can more effectively clarify the impact of PM on the human body in vitro and find out the causes of cardiopulmonary diseases.
In addition to well-known respiratory diseases such as COPD, fine PM in the air that is inhaled into the lungs are translocated to the bloodstream and transported to the blood vessels and heart, where they induce arrhythmia, reduce myocardial contractility, and reduce coronary blood flow, thereby increasing the incidence and mortality of cardiopulmonary diseases. Related studies have shown that the harmful effects of fine PM on health may reach an uncontrollable point by 2030. Therefore, it is essential to quickly and accurately elucidate their effects on the human body, determine the causes of disease, and formulate response strategies.
Although epidemiological and clinical studies have produced much evidence of the effects of fine PM on human health, it has not yet been fully explained how physiological responses and cellular and molecular mechanisms of change and injury are caused. Currently, most health evaluation studies of fine PM are conducted through cell culture or animal experiments. Cell-based studies are still greatly limited compared to the complex structures of physiological mechanisms in humans, and it is impossible to simulate the complex conditions and the interrelated physiological information of the entire organism. Animal experiments play an important role in studies on fine PM, where they allow in vivo toxicological testing by exposing animals to various fine PM environments via the oral and dermal routes. Although animals can inhale fine PM and develop comprehensive systemic outcomes, there is often a large difference between mechanistic and genetic indicator data and clinical outcomes. This is primarily due to differences among species and their physiological functions, such as differences in respiratory rate between experimental mice and humans, as well as the problems of genetics, low throughput, high cost, and ethical concerns. These reasons have caused difficulty when investigating the causes of air pollution and associated human health hazards, the analysis of biomarkers, and the development of future pollution control strategies. Organ-on-a-chip biomimetic technology will bring unlimited potential for breaking through the bottlenecks faced in previous studies.
Reviewing the current development of organ-on-chips, most research focuses on drug development and disease models (Huh et al., 2012; Esch et al., 2015; Benam et al., 2016b). Except for the toxicological applications of lung-on-a-chip and cigarette smoking, other integrated studies related to environmental PM have not been extensive. According to previous reviews (see the section on Particulate matter and respiratory system and Particulate matter and cardiovascular effects), there are several clues worthy of attention such as DNA damage, inflammatory injury and PM translocation. For these research topics, it is believed that there is a great opportunity to obtain more undiscovered information by applying current organ-on-chips and multi-organ chips technology. For example, DNA damage and inflammatory injury could refer to related research on drug toxicity testing, translocation of PM could refer to related research on nanoparticle drug delivery, and further research on chronic toxicology could refer to the multi-organ chips model with PKs and PDs parameters. On the other hand, organ-on-a-chip systems have been shown to be closer to clinical physiology and inflammatory response, compared to traditional experimental model approaches. It has the potential to be a useful in vitro model for investigating the relationship between PM and related diseases. Therefore, the successful development of in vitro chips for simulating organs is a necessary avenue toward modern assessment of the health effects of air pollution. Its rapid and efficient screening capabilities are expected to help governmental agencies and the clinical sector move toward the correct policy and drug development routes, reduce costs, and significantly shorten the process of drug and foreign substance toxicity testing (Ronaldson-Bouchard and Vunjak-Novakovic, 2018).
The technology is still evolving from single organ to multi-organ chips, it is expected to be realized as a long-term and highly active cardiopulmonary chip. Its 3D microenvironment and more biomimetic cyclic dynamic environment combined with fine PM are expected to be applied to health evaluation, physiological indicators, creation of cardiopulmonary disease models, and drug testing. This more precise experimental model is expected to replace existing cell culture or animal experiments and accelerate studies elucidating the effect of fine PM on the human body (Figure 5).
Figure 5. The potential value of organ-on-a-chip biomimetic technology for PM toxicity. Its 3D microenvironment and biomimetic circulating air/liquid dynamic environment are expected to be used for PM health assessment.
All authors contributed toward conceptualization, preparation, and validation of the manuscript.
G-YC would like to acknowledge financial support from National Chiao Tung University (108W204, 108W211), the Ministry of Science and Technology (MOST-109-2636-E-009-007-, MOST-108-2636-E-009-007-), the National Health Research Institutes (NHRI-EX109-10714EC), the Council of Agriculture, Executive Yuan (109AS-24.2.1-AD-U2) and the Higher Education Sprout Project of the National Chiao Tung University and Ministry of Education (MOE), Taiwan. H-EL would like to acknowledge financial support from the Ministry of Science and Technology (MOST 108-2218-E-080-001-).
The authors declare that the research was conducted in the absence of any commercial or financial relationships that could be construed as a potential conflict of interest.
Ahadian, S., Civitarese, R., Bannerman, D., Mohammadi, M. H., Lu, R., Wang, E., et al. (2018). Organ-on-a-chip platforms: a convergence of advanced materials, cells, and microscale technologies. Adv. Healthcare Mater. 7:1700506. doi: 10.1002/adhm.201700506
Al Hanai, A. H., Antkiewicz, D. S., Hemming, J. D. C., Shafer, M. M., Lai, A. M., Arhami, M., et al. (2019). Seasonal variations in the oxidative stress and inflammatory potential of PM2.5 in Tehran using an alveolar macrophage model: the role of chemical composition and sources. Environ. Int. 123, 417–427. doi: 10.1016/j.envint.2018.12.023
Alépée, N., Bahinski, A., Daneshian, M., De Wever, B., Fritsche, E., Goldberg, A., et al. (2014). State-of-the-art of 3D cultures (organs-on-a-chip) in safety testing and pathophysiology. ALTEX 31, 441–477. doi: 10.14573/altex1406111
Analitis, A., Katsouyanni, K., Dimakopoulou, K., Samoli, E., Nikoloulopoulos, A. K., Petasakis, Y., et al. (2006). Short-term effects of ambient particles on cardiovascular and respiratory mortality. Epidemiology 17, 230–233. doi: 10.1097/01.ede.0000199439.57655.6b
Anderson, J. O., Thundiyil, J. G., and Stolbach, A. (2012). Clearing the air: a review of the effects of particulate matter air pollution on human health. J. Med. Toxicol. 8, 166–175. doi: 10.1007/s13181-011-0203-1
Batterman, S., Xu, L., Chen, F., Chen, F., and Zhong, X. (2016). Characteristics of PM(2.5) concentrations across Beijing during 2013-2015. Atmos. Environ. 145, 104–114. doi: 10.1016/j.atmosenv.2016.08.060
Benam, K. H., Novak, R., Nawroth, J., Hirano-Kobayashi, M., Ferrante, T. C., Choe, Y., et al. (2016a). Matched-comparative modeling of normal and diseased human airway responses using a microengineered breathing lung chip. Cell Syst. 3, 456–466.e454. doi: 10.1016/j.cels.2016.10.003
Benam, K. H., Villenave, R., Lucchesi, C., Varone, A., Hubeau, C., Lee, H.-H., et al. (2016b). Small airway-on-a-chip enables analysis of human lung inflammation and drug responses in vitro. Nat. Methods 13, 151–157. doi: 10.1038/nmeth.3697
Bennett, B. A., Spannhake, E. W., Rule, A. M., Breysse, P. N., and Tankersley, C. G. (2018). The acute effects of age and particulate matter exposure on heart rate and heart rate variability in Mice. Cardiovasc. Toxicol. 18, 507–519. doi: 10.1007/s12012-018-9461-3
Boos, J. A., Misun, P. M., Michlmayr, A., Hierlemann, A., and Frey, O. (2019). Microfluidic multitissue platform for advanced embryotoxicity testing in vitro. Adv. Sci. 6:1900294. doi: 10.1002/advs.201900294
Brown, J. S., Gordon, T., Price, O., and Asgharian, B. (2013). Thoracic and respirable particle definitions for human health risk assessment. Part. Fibre Toxicol. 10:12. doi: 10.1186/1743-8977-10-12
Carvalho, M. R., Barata, D., Teixeira, L. M., Giselbrecht, S., Reis, R. L., et al. (2019). Colorectal tumor-on-a-chip system: a 3D tool for precision onco-nanomedicine. Sci. Adv. 5:eaaw1317. doi: 10.1126/sciadv.aaw1317
Choi, H. S., Ashitate, Y., Lee, J. H., Kim, S. H., Matsui, A., Insin, N., et al. (2010). Rapid translocation of nanoparticles from the lung airspaces to the body. Nat. Biotechnol. 28, 1300–1303. doi: 10.1038/nbt.1696
Cochrane, A., Albers, H. J., Passier, R., Mummery, C. L., van den Berg, A., Orlova, V. V., et al. (2019). Advanced in vitro models of vascular biology: human induced pluripotent stem cells and organ-on-chip technology. Adv. Drug Deliv. Rev. 140, 68–77. doi: 10.1016/j.addr.2018.06.007
Costa, J., and Ahluwalia, A. (2019). Advances and current challenges in intestinal in vitro model engineering: a digest. Front. Bioeng. Biotechnol. 7:144. doi: 10.3389/fbioe.2019.00144
Curbani, F., de Oliveira Busato, F., Marcarini do Nascimento, M., Olivieri, D. N., and Tadokoro, C. E. (2019). Inhale, exhale: why particulate matter exposure in animal models are so acute? Data and facts behind the history. Data Brief. 25:104237. doi: 10.1016/j.dib.2019.104237
de Oliveira, A. A. F., de Oliveira, T. F., Dias, M. F., Medeiros, M. H. G., Di Mascio, P., Veras, M., et al. (2018). Genotoxic and epigenotoxic effects in mice exposed to concentrated ambient fine particulate matter (PM2.5) from São Paulo city, Brazil. Part. Fibre Toxicol. 15:40. doi: 10.1186/s12989-018-0276-y
de Oliveira, B. F. A., Ignotti, E., Artaxo, P., do Nascimento Saldiva, P. H., Junger, W. L., and Hacon, S. (2012). Risk assessment of PM2.5 to child residents in Brazilian Amazon region with biofuel production. Environ. Health 11:64. doi: 10.1186/1476-069X-11-64
Donaldson, K., Beswick, P. H., and Gilmour, P. S. (1996). Free radical activity associated with the surface of particles: a unifying factor in determining biological activity? Toxicol. Lett. 88, 293–298. doi: 10.1016/0378-4274(96)03752-6
Du, Y., Xu, X., Chu, M., Guo, Y., and Wang, J. (2016). Air particulate matter and cardiovascular disease: the epidemiological, biomedical and clinical evidence. J. Thorac. Dis. 8, E8–E19. doi: 10.3978/j.issn.2072-1439.2015.11.37
Esch, E. W., Bahinski, A., and Huh, D. (2015). Organs-on-chips at the frontiers of drug discovery. Nat. Rev. Drug Discov. 14, 248–260. doi: 10.1038/nrd4539
Fishler, R., Hofemeier, P., Etzion, Y., Dubowski, Y., and Sznitman, J. (2015). Particle dynamics and deposition in true-scale pulmonary acinar models. Sci. Rep. 5:14071. doi: 10.1038/srep14071
Fishler, R., and Sznitman, J. (2016). A microfluidic model of biomimetically breathing pulmonary acinar airways. J. Vis. Exp. 9:53588. doi: 10.3791/53588
Fröhlich, E., and Salar-Behzadi, S. (2014). Toxicological assessment of inhaled nanoparticles: role of in vivo, ex vivo, in vitro, and in silico studies. Int. J. Mol. Sci. 15, 4795–4822. doi: 10.3390/ijms15034795
Fu, P., Guo, X., Cheung, F. M. H., and Yung, K. K. L. (2019). The association between PM2.5 exposure and neurological disorders: a systematic review and meta-analysis. Sci. Total Environ. 655, 1240–1248. doi: 10.1016/j.scitotenv.2018.11.218
Fuentes-Mattei, E., Rivera, E., Gioda, A., Sanchez-Rivera, D., Roman-Velazquez, F. R., and Jimenez-Velez, B. D. (2010). Use of human bronchial epithelial cells (BEAS-2B) to study immunological markers resulting from exposure to PM(2.5) organic extract from Puerto Rico. Toxicol. Appl. Pharmacol. 243, 381–389. doi: 10.1016/j.taap.2009.12.009
Geraili, A., Jafari, P., Hassani, M. S., Araghi, B. H., Mohammadi, M. H., Ghafari, A. M., et al. (2018). Controlling differentiation of stem cells for developing personalized organ-on-chip platforms. Adv. Healthc. Mater. 7:1700426. doi: 10.1002/adhm.201700426
Gokaltun, A., Yarmush, M. L., Asatekin, A., and Usta, O. B. (2017). Recent advances in nonbiofouling PDMS surface modification strategies applicable to microfluidic technology. Tech. Singap. World Sci. 5, 1–12. doi: 10.1142/S2339547817300013
Guo, C., Zhang, Z., Lau, A. K. H., Lin, C. Q., Chuang, Y. C., Chan, J., et al. (2018). Effect of long-term exposure to fine particulate matter on lung function decline and risk of chronic obstructive pulmonary disease in Taiwan: a longitudinal, cohort study. Lancet Planet. Health 2, e114–e125. doi: 10.1016/S2542-5196(18)30028-7
Halldorsson, S., Lucumi, E., Gómez-Sjöberg, R., and Fleming, R. M. T. (2015). Advantages and challenges of microfluidic cell culture in polydimethylsiloxane devices. Biosens. Bioelectro. 63, 218–231. doi: 10.1016/j.bios.2014.07.029
Hamanaka, R. B., and Mutlu, G. M. (2018). Particulate matter air pollution: effects on the cardiovascular system. Front. Endocrinol. 9:680. doi: 10.3389/fendo.2018.00680
He, F., Liao, B., Pu, J., Li, C., Zheng, M., Huang, L., et al. (2017). Exposure to ambient particulate matter induced COPD in a rat model and a description of the underlying mechanism. Sci. Rep. 7:45666. doi: 10.1038/srep45666
Henry, O. Y. F., Villenave, R., Cronce, M. J., Leineweber, W. D., Benz, M. A., and Ingber, D. E. (2017). Organs-on-chips with integrated electrodes for trans-epithelial electrical resistance (TEER) measurements of human epithelial barrier function. Lab Chip. 17, 2264–2271. doi: 10.1039/C7LC00155J
Herland, A., Maoz, B. M., Das, D., Somayaji, M. R., Prantil-Baun, R., Novak, R., et al. (2020). Quantitative prediction of human pharmacokinetic responses to drugs via fluidically coupled vascularized organ chips. Nat. Biomed. Eng. 4, 421–436. doi: 10.1038/s41551-019-0498-9
Hong, Z., Guo, Z., Zhang, R., Xu, J., Dong, W., Zhuang, G., et al. (2016). Airborne fine particulate matter induces oxidative stress and inflammation in human nasal epithelial cells. Tohoku J. Exp. Med. 239, 117–125. doi: 10.1620/tjem.239.117
Hopke, P. K., Croft, D., Zhang, W., Lin, S., Masiol, M., Squizzato, S., et al. (2019). Changes in the acute response of respiratory diseases to PM2.5 in New York State from 2005 to 2016. Sci. Total Environ. 677, 328–339. doi: 10.1016/j.scitotenv.2019.04.357
Huang, W., Wang, L., Li, J., Liu, M., Xu, H., Liu, S., et al. (2018). Short-term blood pressure responses to ambient fine particulate matter exposures at the extremes of global air pollution concentrations. Am. J. Hypertens. 31, 590–599. doi: 10.1093/ajh/hpx216
Huh, D., Hamilton, G. A., and Ingber, D. E. J. T. (2011). From 3D cell culture to organs-on-chips. Trends Cell Biol. 21, 745–754. doi: 10.1016/j.tcb.2011.09.005
Huh, D., Leslie, D. C., Matthews, B. D., Fraser, J. P., Jurek, S., Hamilton, G. A., et al. (2012). A human disease model of drug toxicity–induced pulmonary edema in a lung-on-a-chip microdevice. Sci. Transl. Med. 4:159ra147. doi: 10.1126/scitranslmed.3004249
Huh, D., Matthews, B. D., Mammoto, A., Montoya-Zavala, M., Hsin, H. Y., and Ingber, D. E. (2010). Reconstituting organ-level lung functions on a chip. Science 328, 1662–1668. doi: 10.1126/science.1188302
Humayun, M., Chow, C.-W., and Young, E. W. K. (2018). Microfluidic lung airway-on-a-chip with arrayable suspended gels for studying epithelial and smooth muscle cell interactions. Lab Chip. 18, 1298–1309. doi: 10.1039/C7LC01357D
Isoherranen, N., Madabushi, R., and Huang, S.-M. (2019). Emerging role of organ-on-a-chip technologies in quantitative clinical pharmacology evaluation. Clin. Transl. Sci. 12, 113–121. doi: 10.1111/cts.12627
Jain, A., Barrile, R., van der Meer, A., Mammoto, A., Mammoto, T., De Ceunynck, K., et al. (2018). Primary human lung alveolus-on-a-chip model of intravascular thrombosis for assessment of therapeutics. Clin. Pharmacol. Ther. 103, 332–340. doi: 10.1002/cpt.742
Jia, Y.-Y., Wang, Q., and Liu, T. (2017). Toxicity Research of PM(2.5) Compositions in vitro. Int. J. Environ. Res. Public Health. 14:232. doi: 10.3390/ijerph14030232
Jodat, Y. A., Kang, M. G., Kiaee, K., Kim, G. J., Martinez, A. F. H., Rosenkranz, A., et al. (2018). Human-derived organ-on-a-chip for personalized drug development. Curr. Pharm. Des. 24, 5471–5486. doi: 10.2174/1381612825666190308150055
Khalid, M. A. U., Kim, Y. S., Ali, M., Lee, B. G., Cho, Y.-J., and Choi, K. H. (2020). A lung cancer-on-chip platform with integrated biosensors for physiological monitoring and toxicity assessment. Biochem. Eng. J. 15:107469. doi: 10.1016/j.bej.2019.107469
Khaniabadi, Y. O., Sicard, P., Takdastan, A., Hopke, P. K., Taiwo, A. M., Khaniabadi, F. O., et al. (2019). Mortality and morbidity due to ambient air pollution in Iran. Clin. Epidemiol. Glob. Health 7, 222–227. doi: 10.1016/j.cegh.2018.06.006
Kido, T., Tamagawa, E., Bai, N., Suda, K., Yang, H.-H. C., Li, Y., et al. (2011). Particulate matter induces translocation of IL-6 from the lung to the systemic circulation. Am. J. Respir. Cell Mol. Biol. 44, 197–204. doi: 10.1165/rcmb.2009-0427OC
Kim, D., Chen, Z., Zhou, L.-F., and Huang, S.-X. (2018). Air pollutants and early origins of respiratory diseases. Chronic Dis. Transl. Med. 4, 75–94. doi: 10.1016/j.cdtm.2018.03.003
Kim, K.-H., Kabir, E., and Kabir, S. (2015). A review on the human health impact of airborne particulate matter. Environ. Int. 74, 136–143. doi: 10.1016/j.envint.2014.10.005
Lee, Y., Choi, J. W., Yu, J., Park, D., Ha, J., Son, K., et al. (2018). Microfluidics within a well: an injection-molded plastic array 3D culture platform. Lab Chip. 18, 2433–2440. doi: 10.1039/C8LC00336J
Li, G., Fang, C., Wang, S., and Sun, S. (2016). The effect of economic growth, urbanization, and industrialization on fine particulate matter (PM2.5) concentrations in China. Environ. Sci. Technol. 50, 11452–11459. doi: 10.1021/acs.est.6b02562
Li, R., Kou, X., Geng, H., Xie, J., Yang, Z., Zhang, Y., et al. (2015). Effect of ambient PM2.5 on lung mitochondrial damage and fusion/fission gene expression in rats. Chem. Res. Toxicol. 28, 408–418. doi: 10.1021/tx5003723
Li, T., Hu, R., Chen, Z., Li, Q., Huang, S., Zhu, Z., et al. (2018). Fine particulate matter [PM(2.5)]: the culprit for chronic lung diseases in China. Chronic Dis. Transl. Med. 4, 176–186. doi: 10.1016/j.cdtm.2018.07.002
Lin, Y., Zou, J., Yang, W., and Li, C.-Q. (2018). A review of recent advances in research on PM2.5 in China. Int. J. Environ. Res. Public Health. 15:438. doi: 10.3390/ijerph15030438
Liu, H., Bolonduro, O. A., Hu, N., Ju, J., Rao, A. A., Duffy, B. M., et al. (2020). Heart-on-a-chip model with integrated extra- and intracellular bioelectronics for monitoring cardiac electrophysiology under acute hypoxia. Nano Lett. 20, 2585–2593. doi: 10.1021/acs.nanolett.0c00076
Lodovici, M., and Bigagli, E. (2011). Oxidative stress and air pollution exposure. J. Toxicol. 2011:487074. doi: 10.1155/2011/487074
Löndahl, J., Pagels, J., Swietlicki, E., Zhou, J., Ketzel, M., Massling, A., et al. (2006). A set-up for field studies of respiratory tract deposition of fine and ultrafine particles in humans. J. Aerosol Sci. 37, 1152–1163. doi: 10.1016/j.jaerosci.2005.11.004
Low, L. A., and Tagle, D. A. (2017). Organs-on-chips: progress, challenges, and future directions. Exp. Biol. Med. (Maywood) 242, 1573–1578. doi: 10.1177/1535370217700523
Marsano, A., Conficconi, C., Lemme, M., Occhetta, P., Gaudiello, E., Votta, E., et al. (2016). Beating heart on a chip: a novel microfluidic platform to generate functional 3D cardiac microtissues. Lab Chip. 16, 599–610. doi: 10.1039/C5LC01356A
Maschmeyer, I., Lorenz, A. K., Schimek, K., Hasenberg, T., Ramme, A. P., Hübner, J., et al. (2015). A four-organ-chip for interconnected long-term co-culture of human intestine, liver, skin and kidney equivalents. Lab Chip. 15, 2688–2699. doi: 10.1039/C5LC00392J
Mastrangeli, M., Millet, S., and van den Eijnden-van Raaij, J. (2019). Organ-on-chip in development: towards a roadmap for organs-on-chip. ALTEX 36, 650–668. doi: 10.14573/altex.1908271
Miller, M. R., Raftis, J. B., Langrish, J. P., McLean, S. G., Samutrtai, P., Connell, S. P., et al. (2017). Inhaled nanoparticles accumulate at sites of vascular disease. ACS Nano. 11, 4542–4552. doi: 10.1021/acsnano.6b08551
Nadeau, K., McDonald-Hyman, C., Noth, E. M., Pratt, B., Hammond, S. K., Balmes, J., et al. (2010). Ambient air pollution impairs regulatory T-cell function in asthma. J. Allergy Clin. Immunol. 126, 845–852.e810. doi: 10.1016/j.jaci.2010.08.008
Nawroth, J. C., Barrile, R., Conegliano, D., van Riet, S., Hiemstra, P. S., and Villenave, R. (2019). Stem cell-based lung-on-chips: the best of both worlds? Adv. Drug Deliv. Rev. 140, 12–32. doi: 10.1016/j.addr.2018.07.005
Nemmar, A., Hoet, P. H. M., Dinsdale, D., Vermylen, J., Hoylaerts, M. F., and Nemery, B. (2003). Diesel exhaust particles in lung acutely enhance experimental peripheral thrombosis. Circulation 107, 1202–1208. doi: 10.1161/01.CIR.0000053568.13058.67
Nemmar, A., Hoet, P. H. M., Vanquickenborne, B., Dinsdale, D., Thomeer, M., Hoylaerts, M. F., et al. (2002). Passage of inhaled particles into the blood circulation in humans. Circulation 105, 411–414. doi: 10.1161/hc0402.104118
Nemmar, A., Holme, J. A., Rosas, I., Schwarze, P. E., and Alfaro-Moreno, E. (2013). Recent advances in particulate matter and nanoparticle toxicology: a review of the in vivo and in vitro studies. BioMed. Res. Int. 2013:279371. doi: 10.1155/2013/279371
Ngoc, L. T. N., Kim, M., Bui, V. K. H., Park, D., and Lee, Y.-C. (2018). Particulate matter exposure of passengers at bus stations: a review. Int. J. Environ. Res. Public Health. 15:E2886. doi: 10.3390/ijerph15122886
Nguyen, T., Jung, S. H., Lee, M. S., Park, T.-E., Ahn, S. K., and Kang, J. H. (2019). Robust chemical bonding of PMMA microfluidic devices to porous PETE membranes for reliable cytotoxicity testing of drugs. Lab Chip. 19, 3706–3713. doi: 10.1039/C9LC00338J
Novak, R., Ingram, M., Marquez, S., Das, D., Delahanty, A., Herland, A., et al. (2020). Robotic fluidic coupling and interrogation of multiple vascularized organ chips. Nat. Biomed. Eng.4, 407–420.
Oleaga, C., Bernabini, C., Smith, A. S. T., Srinivasan, B., Jackson, M., McLamb, W., et al. (2016). Multi-organ toxicity demonstration in a functional human in vitro system composed of four organs. Sci. Rep. 6:20030. doi: 10.1038/srep20030
Oleaga, C., Riu, A., Rothemund, S., Lavado, A., McAleer, C. W., Long, C. J., et al. (2018). Investigation of the effect of hepatic metabolism on off-target cardiotoxicity in a multi-organ human-on-a-chip system. Biomaterials 182, 176–190. doi: 10.1016/j.biomaterials.2018.07.062
Ongaro, A. E., Giuseppe, D. D., Kermanizadeh, A., Crespo, A. M., Mencatti, A., Ghibelli, L., et al. (2020). Polylactic is a sustainable, low absorption, low autofluorescence alternative to other plastics for microfluidic and organ-on-chip applications. Anal. Chem. 92, 6693–6701. doi: 10.1021/acs.analchem.0c00651
Øvrevik, J., Refsnes, M., Låg, M., Holme, J. A., and Schwarze, P.E. (2015). Activation of proinflammatory responses in cells of the airway mucosa by particulate matter: oxidant- and non-oxidant-mediated triggering mechanisms. Biomolecules 5, 1399–1440. doi: 10.3390/biom5031399
Park, M., Joo, H. S., Lee, K., Jang, M., Kim, S. D., Kim, I., et al. (2018). Differential toxicities of fine particulate matters from various sources. Sci. Rep. 8:17007. doi: 10.1038/s41598-018-35398-0
Peixoto, M. S., de Oliveira Galvão, M. F., and de Batistuzzo Medeiros, S. R. (2017). Cell death pathways of particulate matter toxicity. Chemosphere 188, 32–48. doi: 10.1016/j.chemosphere.2017.08.076
Raabe, O. G. (1976). Aerosol aerodynamic size conventions for inertia! sampler calibration. J. Air Pollut. Control Assoc. 26, 856–860. doi: 10.1080/00022470.1976.10470329
Raaschou-Nielsen, O., Beelen, R., Wang, M., Hoek, G., Andersen, Z. J., Hoffmann, B., et al. (2016). Particulate matter air pollution components and risk for lung cancer. Environ. Int. 87, 66–73. doi: 10.1016/j.envint.2015.11.007
Ronaldson-Bouchard, K., and Vunjak-Novakovic, G. (2018). Organs-on-a-chip: a fast track for engineered human tissues in drug development. Cell Stem Cell. 22, 310–324. doi: 10.1016/j.stem.2018.02.011
Rothbauer, M., Zirath, H., and Ertl, P. (2018). Recent advances in microfluidic technologies for cell-to-cell interaction studies. Lab Chip. 18, 249–270. doi: 10.1039/C7LC00815E
Ryu, Y. S., Kang, K. A., Piao, M. J., Ahn, M. J., Yi, J. M., Hyun, Y.-M., et al. (2019). Particulate matter induces inflammatory cytokine production via activation of NFκB by TLR5-NOX4-ROS signaling in human skin keratinocyte and mouse skin. Redox Biol. 21:101080. doi: 10.1016/j.redox.2018.101080
Shadie, A. M., Herbert, C., and Kumar, R. K. (2014). Ambient particulate matter induces an exacerbation of airway inflammation in experimental asthma: role of interleukin-33. Clin. Exp. Immunol. 177, 491–499. doi: 10.1111/cei.12348
Shiraishi, K., Nakajima, T., Shichino, S., Deshimaru, S., Matsushima, K., and Ueha, S. (2019). In vitro expansion of endogenous human alveolar epithelial type II cells in fibroblast-free spheroid culture. Biochem. Biophys. Res. Commun. 515, 579–585. doi: 10.1016/j.bbrc.2019.05.187
Sidorov, V. Y., Samson, P. C., Sidorova, T. N., Davidson, J. M., Lim, C. C., and Wikswo, J. P. (2017). I-wire heart-on-a-chip i: three-dimensional cardiac tissue constructs for physiology and pharmacology. Acta Biomater. 48, 68–78. doi: 10.1016/j.actbio.2016.11.009
Sin, A., Chin, K. C., Jamil, M. F., Kostov, Y., Rao, G., and Shuler, M. L. (2004). The design and fabrication of three-chamber microscale cell culture analog devices with integrated dissolved oxygen sensors. Nat. Rev. Mol. Cell Biol. 20, 338–345. doi: 10.1021/bp034077d
Skardal, A., Murphy, S. V., Devarasetty, M., Mead, I., Kang, H.-W., Seol, Y.-J., et al. (2017). Multi-tissue interactions in an integrated three-tissue organ-on-a-chip platform. Sci. Rep. 7:8837. doi: 10.1038/s41598-017-08879-x
Snow, S. J., Henriquez, A. R., Costa, D. L., and Kodavanti, U. P. (2018). Neuroendocrine regulation of air pollution health effects: emerging insights. Toxicol. Sci. 164, 9–20. doi: 10.1093/toxsci/kfy129
Stucki, J. D., Hobi, N., Galimov, A., Stucki, A. O., Schneider-Daum, N., Lehr, C.-M., et al. (2018). Medium throughput breathing human primary cell alveolus-on-chip model. Sci. Rep. 8:14359. doi: 10.1038/s41598-018-32523-x
Sung, J. H., Wang, Y. I., Narasimhan Sriram, N., Jackson, M., Long, C., Hickman, J. J., et al. (2019). Recent advances in body-on-a-chip systems. Anal. Chem. 91, 330–351. doi: 10.1021/acs.analchem.8b05293
Suwa, T., Hogg, J. C., Quinlan, K. B., Ohgami, A., Vincent, R., and van Eeden, S. F. (2002). Particulate air pollution induces progression of atherosclerosis. J. Am. Coll. Cardiol. 39, 935–942. doi: 10.1016/S0735-1097(02)01715-1
Tsai, D.-H., Amyai, N., Marques-Vidal, P., Wang, J.-L., Riediker, M., Mooser, V., et al. (2012). Effects of particulate matter on inflammatory markers in the general adult population. Part. Fibre Toxicol. 9:24. doi: 10.1186/1743-8977-9-24
Tsuda, A., Henry, F. S., and Butler, J. P. (2013). Particle transport and deposition: basic physics of particle kinetics. Compr. Physiol. 3, 1437–1471. doi: 10.1002/cphy.c100085
Turner, M. C., Krewski, D. C, Arden Pope, I., Chen, Y., Gapstur, S. M., et al. (2011). Long-term ambient fine particulate matter air pollution and lung cancer in a large cohort of never-smokers. Am. J. Respir. Crit. Care Med. 184, 1374–1381. doi: 10.1164/rccm.201106-1011OC
Valavanidis, A., Fiotakis, K., Bakeas, E., and Vlahogianni, T. (2005). Electron paramagnetic resonance study of the generation of reactive oxygen species catalysed by transition metals and quinoid redox cycling by inhalable ambient particulate matter. Redox Rep. 10, 37–51. doi: 10.1179/135100005X21606
van den Berg, A., Mummery, C. L., Passier, R., and van der Meer, A. D. (2019). Personalised organs-on-chips: functional testing for precision medicine. Lab Chip. 19, 198–205. doi: 10.1039/C8LC00827B
Vanderburgh, J., Sterling, J. A., and Guelcher, S. A. (2017). 3D printing of tissue engineered constructs for in vitro modeling of disease progression and drug screening. Ann. Biomed. Eng. 45, 164–179. doi: 10.1007/s10439-016-1640-4
Vawda, S., Mansour, R., Takeda, A., Funnell, P., Kerry, S., Mudway, I., et al. (2013). Associations between inflammatory and immune response genes and adverse respiratory outcomes following exposure to outdoor air pollution: a HuGE systematic review. Am. J. Epidemiol. 179, 432–442. doi: 10.1093/aje/kwt269
Wang, G., McCain, M. L., Yang, L., He, A., Pasqualini, F. S., Agarwal, A., et al. (2014). Modeling the mitochondrial cardiomyopathy of Barth syndrome with induced pluripotent stem cell and heart-on-chip technologies. Nat. Med. 20, 616–623. doi: 10.1038/nm.3545
Wang, H., Song, L., Ju, W., Wang, X., Dong, L., Zhang, Y., et al. (2017). The acute airway inflammation induced by PM2.5 exposure and the treatment of essential oils in Balb/c mice. Sci. Rep. 7:44256. doi: 10.1038/srep44256
Wang, J., Huang, J., Wang, L., Chen, C., Yang, D., Jin, M., et al. (2017). Urban particulate matter triggers lung inflammation via the ROS-MAPK-NF-κB signaling pathway. J. Thorac. Dis. 9, 4398–4412. doi: 10.21037/jtd.2017.09.135
Weiner, A. I., Jackson, S. R., Zhao, G., Quansah, K. K., Farshchian, J. N., Neupauer, K. M., et al. (2019). Mesenchyme-free expansion and transplantation of adult alveolar progenitor cells: steps toward cell-based regenerative therapies. NPJ Regen. Med. 4:17. doi: 10.1038/s41536-019-0080-9
Wong, I., and Ho, C.-M. (2009). Surface molecular property modifications for poly(dimethylsiloxane) (PDMS) based microfluidic devices. Microfluid. Nanofluidics. 7, 291–306. doi: 10.1007/s10404-009-0443-4
Wu, Q., Liu, J., Wang, X., Feng, L., Wu, J., Zhu, X., et al. (2020). Organ-on-a-chip: recent breakthroughs and future prospects. Biomed. Eng. Online 19:9. doi: 10.1186/s12938-020-0752-0
Xiao, Y., Zhang, B., Liu, H., Miklas, J. W., Gagliardi, M., Pahnke, A., et al. (2014). Microfabricated perfusable cardiac biowire: a platform that mimics native cardiac bundle. Lab Chip. 14, 869–882. doi: 10.1039/C3LC51123E
Xing, Y. F., Xu, Y. H., Shi, M. H., and Lian, Y. X. (2016). The impact of PM2.5 on the human respiratory system. J. Thorac. Dis. 8, E69–E74. doi: 10.3978/j.issn.2072-1439.2016.01.19
Xu, X., Jiang, S. Y., Wang, T.-Y., Bai, Y., Zhong, M., Wang, A., et al. (2013). Inflammatory response to fine particulate air pollution exposure: neutrophil versus monocyte. PLoS ONE 8:e71414. doi: 10.1371/journal.pone.0071414
Yang, Y., Qin, Z., Zeng, W., Yang, T., Cao, Y., Mei, C., et al. (2017). Toxicity assessment of nanoparticles in various systems and organs. Nanotechnol. Rev. 6:279. doi: 10.1515/ntrev-2016-0047
Yuancheng, L., Kai, Z., Xiao, L., and Yu Shrike, Z. (2018). Blood-vessel-on-a-chip platforms for evaluating nanoparticle drug delivery systems. Curr. Drug Metab. 19, 100–109. doi: 10.2174/1389200218666170925114636
Zanobetti, A., Franklin, M., Koutrakis, P., and Schwartz, J. (2009). Fine particulate air pollution and its components in association with cause-specific emergency admissions. Environ. Health 8:58. doi: 10.1186/1476-069X-8-58
Zhang, B., Korolj, A., Lai, B. F. L., and Radisic, M. (2018). Advances in organ-on-a-chip engineering. Nat. Rev. Mater. 3, 257–278. doi: 10.1038/s41578-018-0034-7
Zhang, Y. S., Zhang, Y.-N., and Zhang, W. (2017). Cancer-on-a-chip systems at the frontier of nanomedicine. Drug Discov. Today 22, 1392–1399. doi: 10.1016/j.drudis.2017.03.011
Keywords: particulate matter, air pollution, respiratory health, cardiovascular effects, organ-on-a-chip
Citation: Yang J-W, Shen Y-C, Lin K-C, Cheng S-J, Chen S-L, Chen C-Y, Kumar PV, Lin S-F, Lu H-E and Chen G-Y (2020) Organ-on-a-Chip: Opportunities for Assessing the Toxicity of Particulate Matter. Front. Bioeng. Biotechnol. 8:519. doi: 10.3389/fbioe.2020.00519
Received: 15 November 2019; Accepted: 01 May 2020;
Published: 29 May 2020.
Edited by:
Josue Sznitman, Technion Israel Institute of Technology, IsraelReviewed by:
Ben Meir Maoz, Tel Aviv University, IsraelCopyright © 2020 Yang, Shen, Lin, Cheng, Chen, Chen, Kumar, Lin, Lu and Chen. This is an open-access article distributed under the terms of the Creative Commons Attribution License (CC BY). The use, distribution or reproduction in other forums is permitted, provided the original author(s) and the copyright owner(s) are credited and that the original publication in this journal is cited, in accordance with accepted academic practice. No use, distribution or reproduction is permitted which does not comply with these terms.
*Correspondence: Huai-En Lu, aGVsQGZpcmRpLm9yZy50dw==; Guan-Yu Chen, Z3Vhbnl1QG5jdHUuZWR1LnR3
†These authors have contributed equally to this work
Disclaimer: All claims expressed in this article are solely those of the authors and do not necessarily represent those of their affiliated organizations, or those of the publisher, the editors and the reviewers. Any product that may be evaluated in this article or claim that may be made by its manufacturer is not guaranteed or endorsed by the publisher.
Research integrity at Frontiers
Learn more about the work of our research integrity team to safeguard the quality of each article we publish.