- Molecular Microbiology, Groningen Biomolecular Sciences and Biotechnology, University of Groningen, Groningen, Netherlands
Lignocellulosic biomass yields after hydrolysis, besides the hexose D-glucose, D-xylose, and L-arabinose as main pentose sugars. In second generation bioethanol production utilizing the yeast Saccharomyces cerevisiae, it is critical that all three sugars are co-consumed to obtain an economically feasible and robust process. Since S. cerevisiae is unable to metabolize pentose sugars, metabolic pathway engineering has been employed to introduce the respective pathways for D-xylose and L-arabinose metabolism. However, S. cerevisiae lacks specific pentose transporters, and these sugars enter the cell with low affinity via glucose transporters of the Hxt family. Therefore, in the presence of D-glucose, utilization of D-xylose and L-arabinose is poor as the Hxt transporters prefer D-glucose. To solve this problem, heterologous expression of pentose transporters has been attempted but often with limited success due to poor expression and stability, and/or low turnover. A more successful approach is the engineering of the endogenous Hxt transporter family and evolutionary selection for D-glucose insensitive growth on pentose sugars. This has led to the identification of a critical and conserved asparagine residue in Hxt transporters that, when mutated, reduces the D-glucose affinity while leaving the D-xylose affinity mostly unaltered. Likewise, mutant Gal2 transporter have been selected supporting specific uptake of L-arabinose. In fermentation experiments, the transporter mutants support efficient uptake and consumption of pentose sugars, and even co-consumption of D-xylose and D-glucose when used at industrial concentrations. Further improvements are obtained by interfering with the post-translational inactivation of Hxt transporters at high or low D-glucose concentrations. Transporter engineering solved major limitations in pentose transport in yeast, now allowing for co-consumption of sugars that is limited only by the rates of primary metabolism. This paves the way for a more economical second-generation biofuels production process.
Introduction
Lignocellulosic biomass, from hardwood, softwood, and agricultural residues, is generally regarded as a promising feedstock for the production of sustainable energy fuels. Also because the readily fermentable agricultural feedstocks like sugar cane and corn interfere in the world food demand (Zaldivar et al., 2002; Solomon, 2010). Lignocellulosic biomass is mainly composed of the carbohydrate polymers cellulose, hemicellulose, and lignin in which the first two are substrates for bioethanol production (Lynd et al., 2005). To release the sugars from the lignocellulosic biomass, hydrolysis is applied which yields a mixture of hexose (mainly from cellulose but also from hemicellulose) and pentose sugars (from hemicellulose). The majority of the hexose and pentose sugars in the hemicellulose fraction is D-glucose and D-xylose, respectively, in a typical mass ratio of 2:1 (Lee, 1997; Carroll and Somerville, 2009; Gírio et al., 2010). However, also L-arabinose (pentose) contributes up to 4.5% of the total amount of carbohydrates in for instance rice straw (Lee, 1997). In industrial fermentation processes, the yeast Saccharomyces cerevisiae is generally used for bioethanol production from lignocellulosic biomass. Since S. cerevisiae cannot naturally ferment pentose sugars like D-xylose and L-arabinose, specific pentose metabolism pathways have been introduced.
Pentose Metabolism
S. cerevisiae has been engineered into a D-xylose-fermenting strain via either the introduction of a xylose reductase and xylitol dehydrogenase (Kotter and Ciriacy, 1993; Tantirungkij et al., 1994; Jeffries, 2006; Hahn-Hagerdal et al., 2007; Hou et al., 2009) or a fungal (Kuyper et al., 2003, 2004, 2005b; Hector et al., 2008) or bacterial (Brat et al., 2009; de Figueiredo Vilela et al., 2013; Demeke et al., 2013a,b) xylose isomerase (Figure 1). The xylose reductase and xylitol dehydrogenase pathway both requires co-factors (NADPH and NAD+, respectively) which potentially cause an redox imbalance and accumulation of by-products such as xylitol and glycerol. However, re-balancing the intracellular redox cofactor levels or change the cofactor specificities of XR or XDH has significantly improved the D-xylose consumption rate (Verho et al., 2003; Watanabe et al., 2007a,b; Matsushika et al., 2008; Hou et al., 2009, 2014; Zhou et al., 2012). In the xylose reductase and xylitol dehydrogenase pathway as well as in the xylose isomerase pathway, xylulose is phosphorylated to xylulose-5-phosphate, which is further metabolized through the pentose phosphate pathway (PPP). In the PPP, xylulose-5-phospate is converted into fructose-6-phosphate and glyceraldehyde-3-phosphate in a molar ratio of 2:1, and these phosphorylated compounds are subsequently metabolized via glycolysis. Various additional genetic modifications have been applied to both variants of these xylose-fermenting strains e.g., overexpression of the pentose phosphate pathway (Johansson and Hahn-Hägerdal, 2002; Karhumaa et al., 2005, 2006; Kuyper et al., 2005a; Bera et al., 2011), deletion of the gre3 gene (Traff et al., 2001; Karhumaa et al., 2005, 2006; Kuyper et al., 2005a), deletion of the pmr1 gene (Verhoeven et al., 2017) and many other genes as reviewed by Moyses et al. (2016).
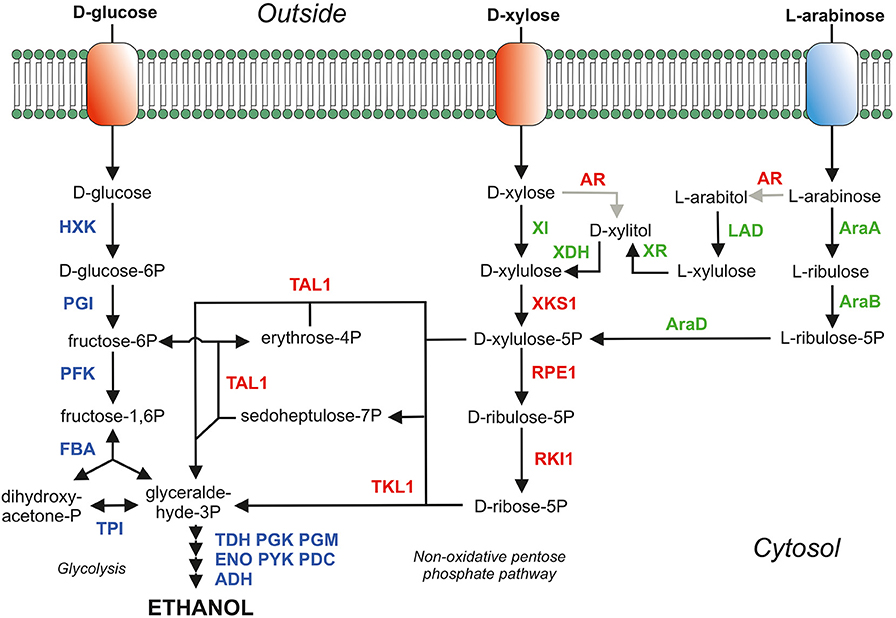
Figure 1. Main sugar consumption pathways in S. cerevisiae. The proteins depicted in blue belong the glycolysis and ethanol production (fermentation). Proteins in red are homologous over-expressed proteins of the pentose phosphate pathway (PPP) and AR; the aldose/xylose/arabinose reductase (EC:1.1.1.21) which is over-expressed in the yeast strains expressing XDH (xylitol dehydrogenase; EC:1.1.1.9) but is deleted in yeast strains expressing XI (xylose isomerase; EC:5.3.1.5). In green are depicted the heterologously over-expressed proteins to metabolize D-xylose (XI and XDH) and the proteins for L-arabinose metabolism [AraA (isomerase; EC:5.3.1.4), AraB (ribulokinase; EC:2.7.1.16) and AraD (epimerase; EC:5.1.3.4)]. To prevent arabitol formation in an L-arabinose consuming yeast strain expressing the AraBAD pathway the AR (aldose reductase; EC:1.1.1.21; gre3 gene) was deleted. The AR was, however, over-expressed in the L-arabinose pathway expressing LAD (L-arabinitol 4-dehydrogenase; EC:1.1.1.12) and XR (L-xylulose reductase; EC:1.1.1.10). Adapted from De Waal P.P., patent WO2003/062430 and WO2008/041840, with permission.
L-arabinose utilization has been explored significantly less compared to D-xylose utilization due to the lower abundance of L-arabinose in lignocellulosic biomass. Like D-xylose, L-arabinose can be utilized by S. cerevisiae via two pathways: an isomerization pathway consisting of L-arabinose isomerase (AraA), L-ribulokinase (AraB), and L-ribulose-5-phosphate 4-epimerase (AraD) from bacteria such as Escherichia coli, Bacillus subtilis, and Lactobacillus plantarum (Sedlak and Ho, 2001; Becker and Boles, 2003; Wisselink et al., 2007) or a reduction/oxidation-based pathway consisting of an aldose reductase (AR), L-arabinitol 4-dehydrogenase (LAD), L-xylulose reductase (LXR), D-xylulose reductase (XDH), and a xylulokinase (XK) (Richard et al., 2003; Hahn-Hagerdal et al., 2007; Bettiga et al., 2009). L-arabinose metabolism continues, in both variants of L-arabinose metabolism, in the formation of D-xylulose-5-phosphate (Figure 1). Therefore, the PPP genes were also over-expressed yielding increased L-arabinose consumption rates (Becker and Boles, 2003; Hahn-Hagerdal et al., 2007; Wisselink et al., 2007; Bettiga et al., 2009).
Monosaccharide Transport
In yeast, sugar transport is facilitated by the sugar porter family which is the largest within the major facilitator superfamily (MFS), and includes proteins from bacteria, archaea and eukaryotes, with a high level of structural and functional similarity (Maiden et al., 1987; Baldwin and Henderson, 1989; Henderson and Maiden, 1990). Although the proteins belonging to the MFS family exhibit strong structural conservation, they may share little sequence similarity (Vardy et al., 2004). These permeases consist of two sets of six hydrophobic transmembrane-spanning (TMS) α-helices connected by a hydrophilic loop. In yeast, many monosaccharide transporters operate by facilitated diffusion, an energy-independent mechanism that equilibrates the monosaccharide concentration in the cytoplasm with that of the extracellular medium. In sugar transporters six conserved motifs (K/RXGRR, RX3GX3G/A, PESPR, PETK, SVQWR, and GRXLXGXGXGX6PXYXSEXAPX3RG XLX4QLXITXGX3A) have now been found, irrespective of their mechanism or substrate specificity (Maiden et al., 1987; Henderson and Maiden, 1990; Griffith et al., 1992; Horák, 1997; Pao et al., 1998; Young et al., 2014). D-glucose transport in S. cerevisiae is mediated by the hexose transporter (Hxt) family of sugar transporters (Kruckeberg, 1996; Boles and Hollenberg, 1997). These transporters mediate facilitated diffusion of the hexose sugars and are usually of low affinity but high capacity. A strain, in which the main sugar transporter genes hxt1-17 and gal2 were deleted, was found to be unable to grow on D-glucose (Wieczorke et al., 1999; Hamacher et al., 2002). A similar deletion strain, lacking only the main expressed hexose transporters (hxt1-7 and gal2) shows minor growth rates on D-glucose (Reifenberger et al., 1995).
S. cerevisiae lacks specific D-xylose or L-arabinose transporters, but still is capable of transporting these pentoses via the endogenous Hxt family of D-glucose transporters and the galactose permease, Gal2, respectively (Kou et al., 1970). This transport is of low affinity, and D-xylose transport is readily outcompeted by D-glucose. This implies that cells only start to transport and metabolize D-xylose once D-glucose is depleted which is undesirable for a robust industrial bioethanol process that must rely on the co-consumption of the sugars available in the growth medium. To circumvent the problem of poor D-xylose and L-arabinose transport, various studies have attempted to provide S. cerevisiae with a specific pentose transporter from a heterologous source which is discussed in the next section.
Heterologous Expression of Pentose Transporters
Because S. cerevisiae lacks specific pentose transporters, one important strategy in the engineering of pentose metabolism is the introduction of specific pentose transporters from heterologous hosts, i.e., other yeast or fungi, bacterial and transporters of plants. The following section summarizes the main approaches and achievements.
Heterologous Xylose Transporters
CiGxf1 and CiGxs1—Two transporters have been isolated from Candida intermedia PYCC 4715: the high-capacity and low-affinity D-glucose/D-xylose facilitated diffusion transporter CiGxf1 and the high-affinity D-xylose-proton symporter CiGxs1. Both genes were expressed in S. cerevisiae and the kinetic parameters of D-glucose and D-xylose transport were determined (Leandro et al., 2006; Young et al., 2014) (Table 1). CiGxf1 was expressed in the various recombinant xylose-fermenting S. cerevisiae strains in which it displayed approximately a 2-fold lower Km value for D-xylose transport compared to a control strain that relies on the endogenous set of Hxt transporters. In aerobic batch cultivation, the specific growth rate was significantly higher at low D-xylose concentration (4 g/L), when CiGxf1 was expressed, whereas it remained unchanged at high D-xylose concentration (40 g/L). These results suggest recombinant xylose-utilizing S. cerevisiae only benefit from such specific transporters at low D-xylose concentrations (Runquist et al., 2009; Fonseca et al., 2011; Tanino et al., 2012; Diao et al., 2013). Furthermore, CiGxf1 was used in various S. cerevisiae strains and contributed to increased growth and D-xylose consumption rate (Fonseca et al., 2011; Olofsson et al., 2011; Diao et al., 2013).
CiGxs1 is the first yeast D-xylose/D-glucose-H+ symporter to be characterized at the molecular level (Leandro et al., 2006). Unlike facilitated diffusion, coupling to the proton motive force allows cells to accumulate sugars against their concentration gradient even at low extracellular sugar concentrations. Such conditions can be favorable in order to saturate the intracellular metabolic enzymes such as the xylose isomerase that exhibits a low affinity for its substrates (Lee et al., 2017). Overexpression of CiGxs1 improved D-xylose consumption and ethanol production in a yeast harboring an XI-based xylose metabolic pathway (Tanino et al., 2012) but transport occurred at low rates, i.e., Vmax is 5 nmol/mgDW.min. Selection for improved growth on D-xylose yielded transporter mutants with vastly improved Vmax values (Table 1) and these displayed an increase in high cell density sugar consumption rates. Analysis of the mutations highlighted several important residues influencing transporter function including a point mutation at F40 of CiGxs1 (Young et al., 2012). Several other mutations (M40V, I117F, and N326H), obtained via error-prone PCR, yielded a CiGxs1 transporter with substantially improved D-xylose transport rates in the presence of D-glucose and even enabled co-utilization of D-glucose and D-xylose (Li et al., 2016). Young et al. rewired the CiGxs1 transporter into a more specific xylose transporter via mutagenesis of a conserved motif (G-G/F-X-X-X-G) yielding a mutant (CiGxs1 V38F L39I F40M) that abrogated the D-glucose uptake and slightly increased the D-xylose uptake rate although D-glucose still inhibited growth on D-xylose (Young et al., 2014).
At5g59250/At5g17010—These genes encode sugar transporters from Arabidopsis thaliana, and were classified as xylose transporter homologs based on sequence similarity to known xylose–H+ symporters (Johnson and Thomas, 2007). These proteins were heterologously expressed in S. cerevisiae and found to correctly localize at the cell periphery using GFP fusion proteins. The respective stains showed increased levels of D-xylose accumulation compared to the control strain, but also accumulation of D-glucose was improved (Hector et al., 2008). In a comparative analysis of various heterologously expressed sugar transporters, At5g59250 showed only a slight improvement in the D-xylose uptake kinetics employing a strain harboring the native Hxt landscape, but this result was not statistically significant. In contrast, the aforementioned CiGxf1 transporter showed the most significant improvement of D-xylose uptake and growth on D-xylose (Runquist et al., 2010).
PsSut1, PsSut2, PsSut3, SsXut1, SsXut3, SsHxt2.6, and SsQup2—Pichia stipitis (also known as Scheffersomyces stipitis) is an excellent xylose-fermenting organism (Does and Bisson, 1989; Grootjen et al., 1991) and is frequently used as a source for heterologous xylose transporters. PsSut1, PsSut2, and PsSut3 encoding glucose transporters were expressed in a S. cerevisiae hxt null mutant strain, and all three proteins restored growth on D-glucose. The individual PsSut proteins show Km values for D-glucose in the millimolar range whereas the affinity for D-xylose was considerably lower. PsSut2 showed the best affinity for D-xylose (49 mM) but PsSut1 exhibits a higher Vmax of 132 nmol/mgDW.min (Table 1) (Weierstall et al., 1999). Furthermore, expression of PsSut1 in a xylose metabolizing S. cerevisiae strain increased both the D-xylose uptake ability and ethanol productivity during D-xylose fermentation. A similar effect was observed for D-glucose using this transporter (Katahira et al., 2008). Improved D-xylose uptake and utilization was demonstrated with a strain that expressed PsSut1 while still harboring the native Hxt landscape, but this was only observed when cells were grown on D-xylose (Runquist et al., 2010). SsXut1 and SsXut3, isolated from Scheffersomyces (Pichia) stipitis, exhibit moderate sugar transport rates but with a preference for D-xylose. When expressed in a S. cerevisiae hxt null strain these transporters also supported growth on D-glucose (Young et al., 2011). D-xylose uptake via SsXut1 was confirmed by high density fermentations on solely D-xylose or D-glucose/D-xylose (de Sales et al., 2015). The E538K mutation in SsXut3 improved both the affinity and Vmax for D-xylose (see Table 1), and also allowed better growth on low concentrations of D-xylose (Young et al., 2012). In another approach, the hxt null strain (Δhxt1-7 and Δgal2) of xylose fermenting S. cerevisiae strain was transformed with a genomic DNA library from S. stipitis and screened for sustaining growth on D-xylose. This led to the identification of three transporter genes, i.e., the previously identified SsXut1 permease and two new transporter genes, SsHXT2.6 and SsQUP2. High cell density fermentations using D-glucose and D-xylose showed that SsXut1 consumed the highest amount of D-xylose as compared to SsHxt2.6 and SsQup2. Although no direct uptake studies were performed with these transporters, they also transport D-glucose and thus are not selective for D-xylose only (de Sales et al., 2015).
Mgt05196—The transporter Mgt05196 was isolated from Meyerozyma (Pichia) guilliermondii, an anaerobic xylose metabolizing yeast that is equipped with high D-xylose transport rates. Several key amino acid residues of Mgt05196 were analyzed by site-directed mutagenesis for improved D-xylose transport. The F432A and N360S mutations enhanced the D-xylose transport activities of Mgt05196. Furthermore, the N360F mutation corresponding to the conserved asparagine 376 in Gal2 (Farwick et al., 2014), rendered transport of D-xylose insensitive to D-glucose inhibition. Although this transporter was expressed heterologously in S. cerevisiae, growth rates on D-xylose were comparable, albeit slightly lower, to Gal2 supported growth on D-xylose (Wang et al., 2015). No kinetic data was recorded for Mgt05196.
AnHxtB—This is a glucose transporter from the filamentous fungus Aspergillus nidulans (dos Reis et al., 2013). When expressed in an xylose fermenting S. cerevisiae hxt-null strain, AnHxtB supported growth on D-xylose, but growth was slow in line with the low Vmax (19 nmol/mgDW.min) of this transporter. AnHxtB however shows a high affinity for D-xylose, 0.54 mM (Table 1) however its performance on D-glucose was not tested (Colabardini et al., 2014).
BsAraE—In order to facilitate D-xylose transport and hence increase xylitol production, an arabinose:H+ symporter (BsAraE) from the bacterium Bacillus subtilis was expressed in the hxt null strain of S. cerevisiae. This resulted in a 4-fold increase in D-xylose consumption. When BsAraE was overexpressed in a S. cerevisiae strain with the full hxt transporter landscape, D-xylose consumption and xylitol production was increased considerably. These experiments were carried out under D-glucose limiting conditions, thus the competitive effect of D-glucose was not tested (Kim et al., 2017).
Heterologous Arabinose Transporters
AmLat1 and AmLat2—These are specific L-arabinose transporters from the fermenting yeast Ambrosiozyma monospora. When AmLat1 and AmLat2 were expressed in a S. cerevisiae mutant in which the main hexose transporters were deleted, L-arabinose transport was observed. These transporters could not restore growth on D-glucose, D-fructose, D-mannose or D-galactose suggesting a high specificity for L-arabinose. At 100 mM L-arabinose, AmLat1 and AmLat2 showed uptake rates of 0.2 and 4 nmol/mgDW.min, respectively (Table 2), which is much lower than the rate of L-arabinose uptake observed in A. monospora (Verho et al., 2011) suggesting expression problems. An AmLat1-mCherry fusion transport protein was found to transport L-arabinose with high affinity (Km ≈ 0.03 mM) (Londesborough et al., 2014).
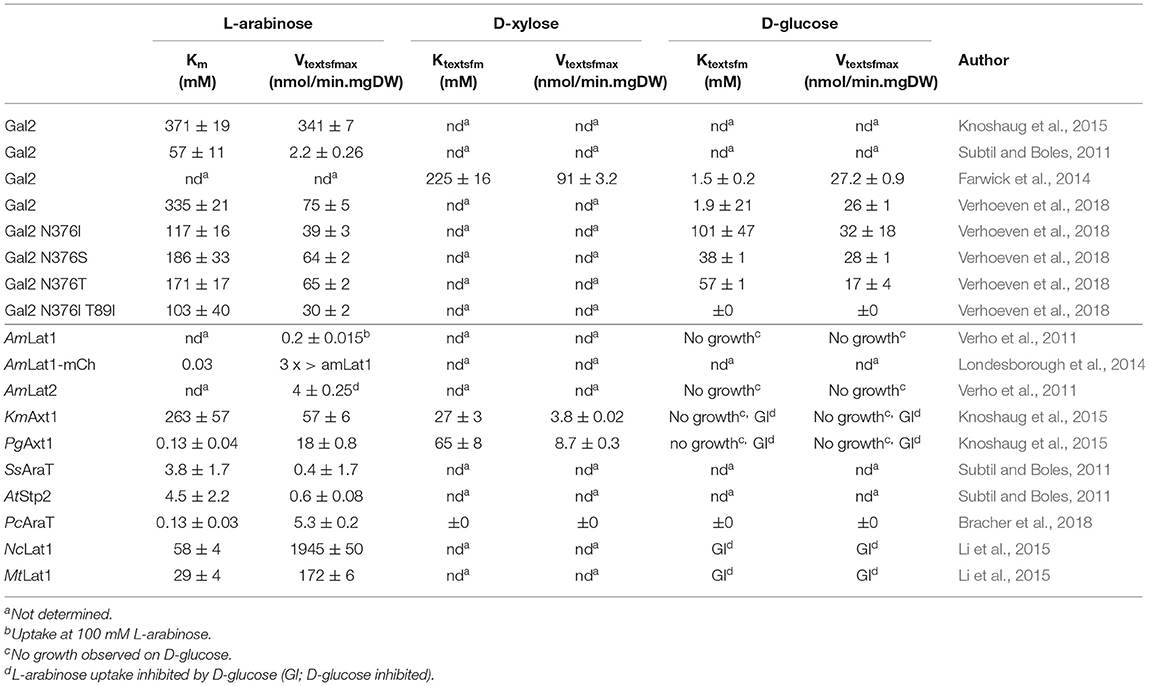
Table 2. Kinetics of endogenous and heterologous expressed L-arabinose transporters in S. cerevisiae.
KmAxt1 and PgAxt1—These are L-arabinose transporters from Kluyveromyces marxianus and Pichia (Meyerozyma) guilliermondii, respectively. Both transporters are also capable of transporting D-xylose. The affinity of KmAxt1 and PgAxt1 for L-arabinose is 263 and 0.13 mM, respectively. The high affinity L-arabinose transporter PgAxt1 showed 30-fold lower transports rates compared to KmAxt1 (Table 2). Both D-glucose and D-xylose significantly inhibited L-arabinose transport by both transporters, which is an unexpected result as growth on D-glucose could not be restored by either of these transporters. KmAxt1 showed a better affinity for D-xylose than for L-arabinose, i.e., 27 vs. 263 mM, respectively. PgAxt1 however is highly specific for L-arabinose as only a low affinity was recorded for D-xylose, i.e., 65 mM (Knoshaug et al., 2015).
SsAraT—This transporter is of the yeast S. stipitis was cloned and identified as L-arabinose transporter allowing growth of S. cerevisiae at low L-arabinose concentrations. This was confirmed in uptake experiments yielding an affinity of L-arabinose of 3.8 mM but a low Vmax of 0.4 nmol/mgDW.min (Table 2). SsAraT appears specific as only poor growth was observed with D-glucose while no growth could be detected on D-xylose (Subtil and Boles, 2011).
AtStp2—The sugar transporter AtStp2 from Arabidopsis thaliana is a H+ symporter with a high affinity for D-galactose (Truernit et al., 1999). When expressed in the S. cerevisiae hxt null strain, AtStp2 proved to be a high affinity L-arabinose transporter (4.5 mM, Table 2) while it barely supported D-glucose uptake. Nevertheless, L-arabinose uptake by AtStp2 was strongly impaired by D-glucose, however, the transporter does not support substantial growth in medium containing 2% D-glucose (Subtil and Boles, 2011). AtStp2 had been reported to support uptake of D-glucose when expressed in Schizosaccharomyces pombe (Truernit et al., 1999). Failure of AtStp2 to enable D-glucose uptake in S. cerevisiae might therefore be due to a D-glucose-mediated post-transcriptional inhibitory. The combined data suggests that AtStp2 is not specific for L-arabinose.
NcLat1 and MtLat1—Two novel H+-coupled L-arabinose transporters, NcLAT1 from Neurospora crassa and MtLAT1 from Myceliophthora thermophile, were identified that exhibit 83% identity but appear to be equipped with different substrate specificities. NcLAT1 has a broad substrate specificity whereas MtLAT1 appears more specific for L-arabinose. The Km values of NcLAT1 and MtLAT1 for L-arabinose were 58 and 29 mM, respectively, with Vmax values of 1945 and 1729 nmol/mgDW.min, respectively. Transport was only partially inhibited by D-glucose. Upon overexpression of NcLAT1 and MtLAT1 in a L-arabinose metabolizing S. cerevisiae strain, L-arabinose utilization, growth and ethanol production was improved. Sequence alignment showed that a conserved asparagine, i.e., N376 of Gal2 (Farwick et al., 2014; Verhoeven et al., 2018), has been replaced into phenylalanine in both NcLAT1 and MtLAT1 (Li et al., 2015). This residue is very critical for the specificity of Hxt transporters for D-glucose, and its substitution in the aforementioned transporters might explain why L-arabinose transport is relatively insensitive to D-glucose inhibition which will be discussed in more detail in the engineering section.
PcAraT—The putative sugar transporter PcAraT (Pc20g01790) of the filamentous fungus Penicillium chrysogenum, is upregulated in L-arabinose-limited cultures compared to D-glucose growth. When expressed in a L-arabinose-fermenting S. cerevisiae strain in which GAL2 was deleted, growth on L-arabinose could be restored. PcAraT is a H+:L-arabinose symporter with high-affinity for L-arabinose (Km = 0.13 mM) while D-xylose and D-glucose are not transported (Bracher et al., 2018).
Despite the many attempts to express heterologous transporters in pentose-utilizing S. cerevisiae, this approach did not yield the solution sought for. The general picture that emerged from those studies are problems with low activity and stability of such foreign transporters likely caused by protein misfolding and subsequent degradation by the yeast endogenous protein quality control mechanisms (Steffensen and Pedersen, 2006; Nikko and Pelham, 2009; Subtil and Boles, 2011; Emmerstorfer et al., 2014; Sen et al., 2016). Also, the high specificity equipped with some of these systems often occurs at the expense of catalytic turnover, while it is essential that these transporters can maintain high rates of transport to keep up with sugar metabolism. Another major disadvantage of the heterologously expressed pentose transporters is that (nearly) all are inhibited by D-glucose therefore preventing co-consumption of D-xylose and D-glucose. Therefore, alternative methods have been explored among which utilizing a mutated endogenous transporter landscape as discussed below.
Engineering of Endogenous Yeast Hexose Transporters
One potential solution to the stability and turnover issue is the engineering of endogenous Hxt transporters for an improved selectivity toward pentose sugars. The main advantages of endogenous transporters is that they are well-expressed and smoothly integrated in the yeast regulatory systems that control the expression and posttranslational degradation under conditions of high and low extracellular D-glucose concentration. Rational engineering of the substrate specificity is complicated by the lack of an atomic structure of these transporters and thus solely relies on structural modeling. Evolutionary engineering of the yeast sugar transporters turned out to be a very effective method to obtain specificity mutants that could be further tailored by directed mutagenesis as discussed in this section.
D-Xylose Transport
D-xylose transport in S. cerevisiae is mediated by proteins of the Hxt family (Hamacher et al., 2002) and more specifically Hxt1 (Nijland et al., 2016), Hxt2 (Saloheimo et al., 2007; Shin et al., 2015), Hxt3/Hxt36 (Nijland et al., 2014), Hxt4, Hxt5, Hxt7 (Saloheimo et al., 2007), Hxt11 (Shin et al., 2015), and Gal2 (Farwick et al., 2014). A major drawback of all of the endogenous Hxt transporters is their low affinity for D-xylose as compared to D-glucose, which results in D-glucose being the preferred substrate for uptake and metabolism in mixed sugar fermentations (Kotter and Ciriacy, 1993; Lagunas, 1993; van Zyl et al., 1999; Subtil and Boles, 2012). Various techniques have been used to increase D-xylose transport in S. cerevisiae focusing on the endogenous Hxt transporters. This involved evolutionary engineering, error-prone PCR, gene shuffling, over-expression, and interference in the endogenous regulatory network. In these experimental approaches two different pentose utilizing yeast strains were employed: (1) a multiple hexokinase deletion strain which is unable to phosphorylate D-glucose and thus cannot grow on D-glucose. Such strains can be used to select for improved D-xylose transport in the presence of high concentrations of D-glucose (Farwick et al., 2014; Nijland et al., 2014, 2017; Reznicek et al., 2015; Shin et al., 2015; Li et al., 2016) and (2) a hexose transporter deletion strain in which the main, or even all, Hxt transporters have been deleted. This strain cannot grow on D-xylose because of an inability to transport this pentose. However, it can be used to express specific sugar transporters and for the selection of mutants that show an enhanced growth on D-xylose (Gonçalves et al., 2014; Reznicek et al., 2015; Nijland et al., 2018). Both approaches yielded various mutations in endogenous sugar transporters that either contribute to an improved D-xylose transport or to a reduced sensitivity toward competitive inhibition by D-glucose. Although several mutations were found in a variety of Hxt transporters that contribute to the specificity change, the most important residue identified is a conserved asparagine present in all Hxt transporters and that fulfills a pivotal role in D-glucose recognition. This asparagine at position 366, 367, 370, and 376 in Hxt11 (Shin et al., 2015), Hxt36 (Nijland et al., 2014), Hxt7 and Gal2 (Farwick et al., 2014), respectively, was mutated to different amino acids, all causing a reduced D-glucose affinity and in some cases improving the D-xylose affinity. In Gal2, the N376F mutation completely abolished the uptake of D-glucose while the affinity for D-xylose increased from 225 to 91 mM (Farwick et al., 2014). The N367A mutation in Hxt36 yielded a transporter with a higher affinity for D-xylose (25 mM) but with a lower Vmax of 29 nmol/mgDW.min as compared to 37 nmol/mgDW.min for the Gal2 N367F mutant. The same error PCR strategy yielded the Gal2 N376Y mutant (Farwick et al., 2014) which, upon the addition of a M435I mutation, showed an improved Vmax for D-xylose. This improved the growth rate on D-xylose in the presence of a 4-fold excess of D-glucose. The additional benefit of the M435I mutations was, however, not observed in the Gal2 N376F M435I double mutant (Farwick et al., 2016).
In an evolutionary approach using a xylose metabolizing yeast strain lacking the main Hxt transporter, selection for improved growth on D-xylose resulted in the expression of the normally cryptic hxt11 gene (Shin et al., 2015). Indeed, over-expression of Hxt11 in the transporter-deficient strain resulted in improved growth on D-xylose. Further selection for glucose-insensitive xylose transport employing a quadruple hexokinase deletion yielded mutations at N366 of Hxt11 that reversed the transporter specificity for D-glucose into D-xylose while maintaining high D-xylose transport rates. In particular because of the high xylose transport rates, the Hxt11 N366T mutant enabled the efficient co-fermentation of D-xylose and D-glucose at industrially relevant sugar concentrations when expressed in a strain lacking the hxt1–7 and gal2 genes. Among the various Hxt transporters, the Hxt11 N366T mutant is equipped with the most favorable D-xylose uptake characteristics, i.e., a Km of 46 mM and a Vmax of 76 nmol/mgDW.min (Table 1) (Shin et al., 2015). Another advantage of Hxt11 is that this normally cryptic transporter is stably expressed at high D-glucose concentrations at the plasma membrane without any sign of degradation (Shin et al., 2015, 2017) in contrast to for instance Hxt2 (Kruckeberg et al., 1999; Shin et al., 2017) and Hxt7 (Krampe et al., 1998; Snowdon and van der Merwe, 2012). The structure of Hxt36 (Nijland et al., 2014) (Figure 2) and Gal2 (Farwick et al., 2014) were modeled according to the crystal structure of XylE, a MFS transporter of Escherichia coli with D-xylose in the binding site (Sun et al., 2012). Both models clearly show that in the sugar binding site the CH2-OH group of D-glucose is in close proximity to N367 or N376, respectively. Any other amino acid that is bulkier or more hydrophobic interfered with the binding of D-glucose. This particular CH2-OH group is absent in D-xylose, and thus the mutations leave D-xylose binding mostly unaltered.
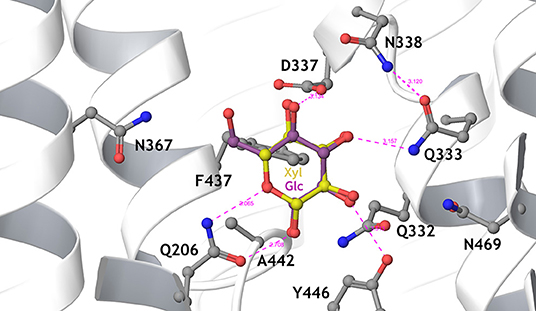
Figure 2. Detailed view of the sugar-binding pocket of the Hxt36 homology model, showing the first shell amino acid side chains that interact with bound glucose (cyan) and xylose (yellow). N367 is located to the left, pointing the side chain toward the 6-OH and 6-CH2 of glucose. Most residues in this pocket are strictly conserved between Hxt36 and XylE, apart from D337 (I in XylE), A442 (G in XylE), Y446 (W in XylE), and N469 (Q in XylE). Reproduced from Nijland et al. (2014) with permission.
Using Gal2 as template, random mutagenesis was used to obtain mutants with an increased affinity for D-xylose. This yielded various mutations but the threonine at position 386 in TMS 8 stands out as the respective mutants allow increased growth rates at low concentrations of D-xylose. This allowed for D-xylose and D-glucose co-consumption at very low sugar concentrations (Reznicek et al., 2015). A similar improvement in D-xylose affinity was observed with mutants of Hxt2 that were obtained via gene shuffling with other Hxt proteins. A mutation of a cysteine to proline at position 505 yielded a 3-fold improvement in D-xylose affinity (Nijland et al., 2018). In another study (Young et al., 2014), mutagenesis of conserved motifs was used to convert Hxt7 into a xylose transporter. These mutations localize to the GGFVFG motif (residues 75-80 and not 36-41 as cited) that is part of TMS 1. The V78I and F79M mutations caused improved growth rates on D-xylose. Like with the other Hxt transporters, mutations at N340 can eliminate D-glucose transport in Hxt7 (Kasahara et al., 2007) and when coupled to the V78I, F79M double mutant, improved growth on D-xylose occurred while growth on D-glucose was defective (Young et al., 2014). In another evolutionary engineering study, a F79S mutation in Hxt7 was obtained that improved D-xylose uptake (Reider Apel et al., 2016).
Remarkably, another mechanism to improve D-xylose metabolism is via the overexpression of Hxt transporters as shown for Hxt1 (Tanino et al., 2012; Gonçalves et al., 2014), Hxt2 and Hxt7 (Gonçalves et al., 2014), with is consistent with the notion that the uptake of D-xylose is the limiting step in metabolism. Although different strain backgrounds were used, in mixed sugar fermentations, over-expression of Hxt1 resulted in fastest sugar consumption (Tanino et al., 2012) while Hxt2 supported the best co-consumption but only at low sugar concentrations (Gonçalves et al., 2014). Evolutionary engineering aimed at improving growth on D-xylose led to the upregulation of Hxt2 (de Vilela et al., 2015). Similar results were obtained via a mutation of the glucose sensitive co-repressor Cyc8 causing the generic upregulation of virtually all hxt genes concomitantly with improved D-xylose transport (Nijland et al., 2017). Importantly, all of the abovementioned approaches indeed elevated the D-xylose flux into the cells but, as expected, do not result in sugar co-consumption as D-glucose remains to be the preferred substrate. In a recent study, in which xylitol was produced from lignocellulosic biomass by a recombinant xylose fermenting S. cerevisiae strain, improved xylose consumption was achieved by overexpression of the maltose transporter ScMal11 (Guirimand et al., 2019). Although it was suggested that this phenomenon was due to ScMal11 supported D-xylose uptake, this was not directly shown in transport assays. Intriguingly, the improved D-xylose consumption was restricted to co-utilization experiments using cellobiose and xylose, and was not observed with a mixture of glucose and xylose.
L-arabinose Transport
L-arabinose uptake has been studied in less detail compared to D-xylose, likely because of its lesser abundance in lignocellulosic biomass and thus limited economic significance. However, in corn fiber hydrolysates and sugar beet pulp, L-arabinose can account for 26% of the total sugar content (Lynd, 1996; Grohmann and Bothast, 1997). Gal2, the S. cerevisiae galactose permease, is capable of transporting arabinose (Kou et al., 1970). Overexpression of Gal2 indeed results in improved L-arabinose uptake (Becker and Boles, 2003; Wang et al., 2013), while a Gal2 deletion strain is unable to metabolize L-arabinose (Wisselink et al., 2009). L-arabinose uptake is inhibited by D-glucose, likely because of competition for transport (Subtil and Boles, 2012). To improve L-arabinose transport, mutations were introduced in Gal2 based on molecular modeling of the substrate binding site. The F85S mutation significantly enhanced the growth rate on L-arabinose and on D-xylose. Furthermore, the F85G mutation increased the L-arabinose transport activity and reduced the specificity of the transporter for D-glucose and D-xylose (Wang et al., 2017). Previously, the corresponding F79S mutation in Hxt7 was reported to improve D-xylose uptake (Reider Apel et al., 2016). This phenylalanine residue is part of the conserved motif “GG/FXXXG” that localizes to TMS1 (Trans membrane spanning domain), and mutations in this region affect the D-xylose and D-glucose transport activity (Young et al., 2014; Knoshaug et al., 2015).
By means of evolutionary engineering of a D-glucose-phosphorylation-negative S. cerevisiae strain on mixed sugar feedstocks (glucose-xylose-arabinose), a strain was evolved that grows on L-arabinose in the presence of D-glucose and D-xylose (Verhoeven et al., 2018). Genome sequencing revealed a mutation of the conserved N376 of Gal2 that corresponds to the key residue in Hxt transporters that determines specificity. In Gal2, this residue was mutated into either a serine, threonine or isoleucine. The Gal2 mutants all showed a decreased D-glucose sensitivity of L-arabinose transport which was accompanied by a reduction of the affinity and Vmax of D-glucose transport (Wisselink et al., 2012; Verhoeven et al., 2018). Reported L-arabinose transport affinities range from 57 mM (Subtil and Boles, 2011) and 371 mM (Knoshaug et al., 2015) (Table 2). Interestingly, in a L-arabinose fermenting S. cerevisiae strain that lacks the main Hxt transporters, also Hxt9 and Hxt10 were found to support the uptake of L-arabinose, albeit less efficient than Gal2 (Subtil and Boles, 2011).
Regulation and Degradation
In S. cerevisiae, like in many other yeast species, D-glucose ensures its own efficient metabolism by serving as an environmental stimulus that regulates the quantity, types, and activity of the Hxt transporters, both at the transcriptional and posttranslational level. The Hxt transporters differ in their affinity for D-glucose (Reifenberger et al., 1997), and correspondingly their expression is regulated by different levels of D-glucose (Diderich et al., 1999; Ozcan and Johnston, 1999). Two regulatory pathways exist that involve the transcriptional repressors Mig1 and Rgt1. These pathways interact to ensure that yeast cells expresses the D-glucose transporters best suited at a given concentration of D-glucose in the medium (Nehlin and Ronne, 1990; Ozcan and Johnston, 1995; Kim et al., 2003, 2006; Roy et al., 2013). Furthermore, also degradation of Hxt transporters is tightly linked to the external D-glucose concentration. Hxt1 and Hxt3 are low-affinity D-glucose transporters (Reifenberger et al., 1997), and are expressed at high D-glucose concentrations (Ozcan and Johnston, 1999; Buziol et al., 2008) while they are degraded via ubiquitination at lower D-glucose concentrations (Snowdon and van der Merwe, 2012; Roy et al., 2014; Nijland et al., 2016). Hxt2 and Hxt6/7 are high-affinity D-glucose transporters (Reifenberger et al., 1997) and are expressed when there is no, or only limited amounts of D-glucose present in the medium (Ozcan and Johnston, 1999; Buziol et al., 2008). Concurrently, these transporters are degraded at high D-glucose concentrations (Snowdon and van der Merwe, 2012; Shin et al., 2017). Hxt4 and Hxt5 exhibit an intermediate affinity for D-glucose although Hxt4 is expressed at lower D-glucose concentrations (Ozcan and Johnston, 1999) while Hxt5 is mainly induced when cells are grown on non-fermentable carbon sources and when the growth rate decreases (Verwaal et al., 2002). Little is known about the degradation of Hxt4 but Hxt5 is stably expressed at the membrane at low D-glucose concentrations (Nijland et al., 2016).
Low-affinity D-glucose Hxt transporters all show increased protein degradation at low D-glucose concentrations and are therefore unsuitable to support growth on D-xylose only. Recently it was shown that the degradation of the low-affinity D-glucose transporters Hxt1 and Hxt3 is too fast to enable growth on D-xylose, despite the fact that these transporters support D-xylose transport with high Vmax values (Nijland et al., 2016). To improve D-xylose and L-arabinose consumption based on these low-affinity D-glucose transporters, protein degradation needs to be prevented, especially at the end of a fermentation when the levels of D-glucose are low. Since protein degradation occurs through ubiquitination of these transporters, the amino-terminal lysine residues of Hxt1, Hxt5 and Hxt36 were mutated into arginine residues (Nijland et al., 2016) or overall protein degradation was reduced in a Rsp5-1ts mutant of the essential E3 ubiquitin ligase, Rsp5 (Snowdon and van der Merwe, 2012). Both studies showed a decreased degradation of the Hxt transporters at low D-glucose concentrations but for only with the respective mutants of Hxt36 (Nijland et al., 2016) improved D-xylose fermentation was observed that could be associated to the improved stability.
Outlook and Perspective
During the last decade, pentose transport has been extensively studied in the yeast S. cerevisiae in order to allow the consumption of the pentose sugars L-arabinose and D-xylose that are present in lignocellulosic biomass. A further goal was to improve co-consumption of hexoses and pentoses. Herein different strategies were investigated: (1) A single Hxt transporter where an endogenous Hxt transporter with improved specificity for D-xylose but that is still able to transport D-glucose, is expressed in a hexose transporter deletion strain (Reznicek et al., 2015; Shin et al., 2015; Farwick et al., 2016; Nijland et al., 2016). Although, co-consumption can be achieved, the total sugar consumption rates remain low, mainly due to D-glucose dependent degradation at either low (e.g., Hxt3) or high (e.g., Hxt7) D-glucose concentrations. (2) Down-regulation of D-glucose consumption via hexokinase mutations yielded an improved co-consumption of D-xylose and D-glucose via an adaptive engineering strategy. This yielded various mutations in D-glucose phosphorylating enzymes (Hxk1, Hxk2, and Glk1). The same phenotype could be obtained by down regulation of the afore mentioned hexokinases which suggests that the rate of intracellular glucose phosphorylation is a decisive factor for metabolic regulations of mixed sugar utilization (Lane et al., 2018). (3) Forced co-consumption in which simultaneous utilization of D-xylose and D-glucose was achieved by deleting the genes encoding glucose-6-phosphate isomerase (Pgi1) and ribulose-5-phosphate epimerase (Rpe1) in a xylose-isomerase-based xylose-fermenting strain. Evolutionary engineering yielded mutations in Hxk2 which improved the rate of the forced co-consumption of D-xylose and D-glucose (Papapetridis et al., 2018). The latter strategy, however, requires stoichiometric mixtures of these two sugars. Apart from these metabolic strategies, the quest for co-consumption was mainly focused on the transport of pentoses which yielded a wealth of information on foreign and endogenous transporters and led to the identification of mutations that either modify the hexose selectivity or transporter stability. The advantage of employing endogenous Hxt transporters is their stable expression and low protein turnover. These transporters are well-integrated in the yeast regulatory network, although protein degradation can be a bottleneck. The disadvantage of these transporters is their low affinity for D-xylose as compared to some of the heterologously expressed xylose transporters, but the latter support D-xylose transport usually with only low uptake rates. Given the wealth of kinetic information available in literature, benchmarking of these transporters based on maximal uptake rates is difficult as this depends on variables such as expression level and the strain, culture and uptake conditions. Through transporter engineering, co-consumption of D-xylose and D-glucose at industrial concentrations has been realized based on single transporter solutions. Although this is a main achievement, to translate this method to real industrial conditions will still be a challenge. The main bottleneck now is that the sugar metabolism flux is distributed over multiple sugars, as the maximal capacity of primary metabolism is exploited. Under such conditions, transport is no longer limiting. Rather primary metabolism represents the main limiting step for faster sugar consumption and shorter fermentation times. Importantly, D-xylose utilization and growth rates are still significantly lower as compared to D-glucose, and thus a careful balance is necessary throughout fermentation. In an industrial setting, obviously robustness remains a main challenge including resistance to toxic byproducts in pretreated feedstocks, and acetic acid that accumulates during fermentation. To make the process industrial ready, a combination of various transporters that will be needed to accommodate the various concentrations of sugar during the entire fermentation and maintain an optimal flux.
Author Contributions
Both authors designed and conceived the study. JN wrote the initial draft of the manuscript, which was edited and corrected by AD.
Funding
This work was performed within the BE-Basic R&D Program (http://www.be-basic.org), which was financially supported by the Dutch Ministry of Economic Affairs, Agriculture and Innovation (EL&I).
Conflict of Interest
The authors declare that the research was conducted in the absence of any commercial or financial relationships that could be construed as a potential conflict of interest.
Acknowledgments
We thank Paul de Waal (DSM) for discussions and continuous support.
References
Baldwin, S. A., and Henderson, P. J. F. (1989). Homologies between sugar transporters from Eukaryotes and Prokaryotes. Annu. Rev. Physiol. 51, 459–471. doi: 10.1146/annurev.ph.51.030189.002331
Becker, J., and Boles, E. (2003). A modified Saccharomyces cerevisiae strain that consumes L-Arabinose and produces ethanol. Appl. Environ. Microbiol. 69, 4144–4150. doi: 10.1128/AEM.69.7.4144-4150.2003
Bera, A. K., Ho, N. W. Y., Khan, A., and Sedlak, M. (2011). A genetic overhaul of Saccharomyces cerevisiae 424A(LNH-ST) to improve xylose fermentation. J. Ind. Microbiol. Biotechnol. 38, 617–626. doi: 10.1007/s10295-010-0806-6
Bettiga, M., Bengtsson, O., Hahn-Hagerdal, B., and Gorwa-Grauslund, M. F. (2009). Arabinose and xylose fermentation by recombinant Saccharomyces cerevisiae expressing a fungal pentose utilization pathway. Microb. Cell Fact. 8:40. doi: 10.1186/1475-2859-8-40
Boles, E., and Hollenberg, C. P. (1997). The molecular genetics of hexose transport in yeasts. FEMS Microbiol. Rev. 21, 85–111. doi: 10.1111/j.1574-6976.1997.tb00346.x
Bracher, J. M., Verhoeven, M. D., Wisselink, H. W., Crimi, B., Nijland, J. G., Driessen, A. J. M., et al. (2018). The Penicillium chrysogenum transporter PcAraT enables high-affinity, glucose-insensitive l-arabinose transport in Saccharomyces cerevisiae. Biotechnol. Biofuels 11:63. doi: 10.1186/s13068-018-1047-6
Brat, D., Boles, E., and Wiedemann, B. (2009). Functional expression of a bacterial xylose isomerase in Saccharomyces cerevisiae. Appl. Environ. Microbiol. 75, 2304–2311. doi: 10.1128/AEM.02522-08
Buziol, S., Warth, L., Magario, I., Freund, A., Siemannherzberg, M., and Reuss, M. (2008). Dynamic response of the expression of hxt1, hxt5 and hxt7 transport proteins in Saccharomyces cerevisiae to perturbations in the extracellular glucose concentration. J. Biotechnol. 134, 203–210. doi: 10.1016/j.jbiotec.2008.02.002
Carroll, A., and Somerville, C. (2009). Cellulosic biofuels. Annu. Rev. Plant Biol. 60, 165–182. doi: 10.1146/annurev.arplant.043008.092125
Colabardini, A., Ries, L. N., Brown, N., dos Reis, T., Savoldi, M., Goldman, M. H. S., et al. (2014). Functional characterization of a xylose transporter in Aspergillus nidulans. Biotechnol. Biofuels 7:46. doi: 10.1186/1754-6834-7-46
de Figueiredo Vilela, L., de Mello, V. M., Reis, V. C. B., Bon, E. P., da Gonçalves Torres, F. A., Neves, B. C., et al. (2013). Functional expression of Burkholderia cenocepacia xylose isomerase in yeast increases ethanol production from a glucose-xylose blend. Bioresour. Technol. 128, 792–796. doi: 10.1016/j.biortech.2012.10.014
de Sales, B. B., Scheid, B., Gonçalves, D. L., Knychala, M. M., Matsushika, A., Bon, E. P. S., et al. (2015). Cloning novel sugar transporters from Scheffersomyces (Pichia) stipitis allowing d-xylose fermentation by recombinant Saccharomyces cerevisiae. Biotechnol. Lett. 37, 1973–1982. doi: 10.1007/s10529-015-1893-2
de Vilela, L. F., de Araujo, V. P. G., de Paredes, R. S., da Bon, E. P. S., Torres, F. A. G., Neves, B. C., et al. (2015). Enhanced xylose fermentation and ethanol production by engineered Saccharomyces cerevisiae strain. AMB Express 5:16. doi: 10.1186/s13568-015-0102-y
Demeke, M. M., Dietz, H., Li, Y., Foulquié-Moreno, M. R., Mutturi, S., Deprez, S., et al. (2013a). Development of a D-xylose fermenting and inhibitor tolerant industrial Saccharomyces cerevisiae strain with high performance in lignocellulose hydrolysates using metabolic and evolutionary engineering. Biotechnol. Biofuels 6:89. doi: 10.1186/1754-6834-6-89
Demeke, M. M., Dumortier, F., Li, Y., Broeckx, T., Foulquié-Moreno, M. R., and Thevelein, J. M. (2013b). Combining inhibitor tolerance and D-xylose fermentation in industrial Saccharomyces cerevisiae for efficient lignocellulose-based bioethanol production. Biotechnol. Biofuels 6:120. doi: 10.1186/1754-6834-6-120
Diao, L., Liu, Y., Qian, F., Yang, J., Jiang, Y., and Yang, S. (2013). Construction of fast xylose-fermenting yeast based on industrial ethanol-producing diploid Saccharomyces cerevisiae by rational design and adaptive evolution. BMC Biotechnol. 13:110. doi: 10.1186/1472-6750-13-110
Diderich, J. A., Schepper, M., van Hoek, P., Luttik, M. A., van Dijken, J. P., Pronk, J. T., et al. (1999). Glucose uptake kinetics and transcription of HXT genes in chemostat cultures of Saccharomyces cerevisiae. J. Biol. Chem. 274, 15350–15359. doi: 10.1074/jbc.274.22.15350
Does, A. L., and Bisson, L. F. (1989). Comparison of glucose uptake kinetics in different yeasts. J. Bacteriol. 171, 1303–1308. doi: 10.1128/jb.171.3.1303-1308.1989
dos Reis, T. F., de Lima, P. B. A., Parachin, N. S., Mingossi, F. B., de Castro Oliveira, J. V., Ries, et al. (2016). Identification and characterization of putative xylose and cellobiose transporters in Aspergillus nidulans. Biotechnol. Biofuels 9:204. doi: 10.1186/s13068-016-0611-1
dos Reis, T. F., Menino, J. F., Bom, V. L. P., Brown, N. A., Colabardini, A. C., Savoldi, M., et al. (2013). Identification of glucose transporters in Aspergillus nidulans. PLoS ONE 8:e81412. doi: 10.1371/journal.pone.0081412
Emmerstorfer, A., Wriessnegger, T., Hirz, M., and Pichler, H. (2014). Overexpression of membrane proteins from higher eukaryotes in yeasts. Appl. Microbiol. Biotechnol. 98, 7671–7698. doi: 10.1007/s00253-014-5948-4
Farwick, A., Bruder, S., Schadeweg, V., Oreb, M., and Boles, E. (2014). Engineering of yeast hexose transporters to transport D-xylose without inhibition by D-glucose. Proc. Natl. Acad. Sci. U.S.A. 111, 5159–5164. doi: 10.1073/pnas.1323464111
Farwick, A., Schadeweg, V., Oreb, M., and Boles, E. (2016). Variants of gal2 Transporter and Their Uses. Patent US10308692B2. WIPO/PCT.
Fonseca, C., Olofsson, K., Ferreira, C., Runquist, D., Fonseca, L. L., Hahn-Hägerdal, B., et al. (2011). The glucose/xylose facilitator Gxf1 from Candida intermedia expressed in a xylose-fermenting industrial strain of Saccharomyces cerevisiae increases xylose uptake in SSCF of wheat straw. Enzyme Microb. Technol. 48, 518–525. doi: 10.1016/j.enzmictec.2011.02.010
Gírio, F. M., Fonseca, C., Carvalheiro, F., Duarte, L. C., Marques, S., and Bogel-Łukasik, R. (2010). Hemicelluloses for fuel ethanol: a review. Bioresour. Technol. 101, 4775–4800. doi: 10.1016/j.biortech.2010.01.088
Gonçalves, D. L., Matsushika, A., de Sales, B. B., Goshima, T., Bon, E. P. S., and Stambuk, B. U. (2014). Xylose and xylose/glucose co-fermentation by recombinant Saccharomyces cerevisiae strains expressing individual hexose transporters. Enzyme Microb. Technol. 63, 13–20. doi: 10.1016/j.enzmictec.2014.05.003
Griffith, J. K., Baker, M. E., Rouch, D. A., Page, M. G., Skurray, R. A., Paulsen, I. T., et al. (1992). Membrane transport proteins: implications of sequence comparisons. Curr. Opin. Cell Biol. 4, 684–695. doi: 10.1016/0955-0674(92)90090-Y
Grohmann, K., and Bothast, R. J. (1997). Saccharification of corn fibre by combined treatment with dilute sulphuric acid and enzymes. Process Biochem. 32, 405–415. doi: 10.1016/S0032-9592(96)00095-7
Grootjen, D. R. J., van der Lans, R. G. J. M., and Luyben, K. C. A. M. (1991). Conversion of glucose/xylose mixtures by Pichia stipitis under oxygen-limited conditions. Enzyme Microb. Technol. 13, 648–654. doi: 10.1016/0141-0229(91)90079-P
Guirimand, G. G. Y., Bamba, T., Matsuda, M., Inokuma, K., Morita, K., Kitada, Y., et al. (2019). Combined cell surface display of β-D-glucosidase (BGL), maltose transporter (MAL11) and overexpression of cytosolic xylose reductase (XR) in Saccharomyces cerevisiae enhance cellobiose/xylose co-utilization for xylitol bio-production from lignocellulosic biomass. Biotechnol. J. 19:e1800704. doi: 10.1002/biot.201800704
Hahn-Hagerdal, B., Karhumaa, K., Jeppsson, M., and Gorwa-Grauslund, M. F. (2007). “Metabolic engineering for pentose utilization in Saccharomyces cerevisiae,” in Biofuels: Advances in Biochemical Engineering/Biotechnology, Vol. 108, ed L. Olsson (Berlin; Heidelberg: Springer), 147–177.
Hamacher, T., Becker, J., Gárdonyi, M., Hahn-Hägerdal, B., and Boles, E. (2002). Characterization of the xylose-transporting properties of yeast hexose transporters and their influence on xylose utilization. Microbiology 148, 2783–2788. doi: 10.1099/00221287-148-9-2783
Hector, R. E., Qureshi, N., Hughes, S. R., and Cotta, M. A. (2008). Expression of a heterologous xylose transporter in a Saccharomyces cerevisiae strain engineered to utilize xylose improves aerobic xylose consumption. Appl. Microbiol. Biotechnol. 80, 675–684. doi: 10.1007/s00253-008-1583-2
Henderson, P. J., and Maiden, M. C. (1990). Homologous sugar transport proteins in Escherichia coli and their relatives in both prokaryotes and eukaryotes. Philos. Trans. R. Soc. Lond. B. Biol. Sci. 326, 391–410. doi: 10.1098/rstb.1990.0020
Horák, J. (1997). Yeast nutrient transporters. Biochim. Biophys. Acta 1331, 41–79. doi: 10.1016/S0304-4157(96)00015-9
Hou, J., Suo, F., Wang, C., Li, X., Shen, Y., and Bao, X. (2014). Fine-tuning of NADH oxidase decreases byproduct accumulation in respiration deficient xylose metabolic Saccharomyces cerevisiae. BMC Biotechnol. 14:13. doi: 10.1186/1472-6750-14-13
Hou, J., Vemuri, G. N., Bao, X., and Olsson, L. (2009). Impact of overexpressing NADH kinase on glucose and xylose metabolism in recombinant xylose-utilizing Saccharomyces cerevisiae. Appl. Microbiol. Biotechnol. 82, 909–919. doi: 10.1007/s00253-009-1900-4
Jeffries, T. W. (2006). Engineering yeasts for xylose metabolism. Curr. Opin. Biotechnol. 17, 320–326. doi: 10.1016/j.copbio.2006.05.008
Johansson, B., and Hahn-Hägerdal, B. (2002). The non-oxidative pentose phosphate pathway controls the fermentation rate of xylulose but not of xylose in Saccharomyces cerevisiae TMB3001. FEMS Yeast Res. 2, 277–282. doi: 10.1016/S1567-1356(02)00114-9
Johnson, D. A., and Thomas, M. A. (2007). The monosaccharide transporter gene family in Arabidopsis and rice: a history of duplications, adaptive evolution, and functional divergence. Mol. Biol. Evol. 24, 2412–2423. doi: 10.1093/molbev/msm184
Karhumaa, K., Fromanger, R., Hahn-Hägerdal, B., and Gorwa-Grauslund, M.-F. (2006). High activity of xylose reductase and xylitol dehydrogenase improves xylose fermentation by recombinant Saccharomyces cerevisiae. Appl. Microbiol. Biotechnol. 73, 1039–1046. doi: 10.1007/s00253-006-0575-3
Karhumaa, K., Hahn-Hägerdal, B., and Gorwa-Grauslund, M.-F. (2005). Investigation of limiting metabolic steps in the utilization of xylose by recombinant Saccharomyces cerevisiae using metabolic engineering. Yeast 22, 359–368. doi: 10.1002/yea.1216
Kasahara, T., Maeda, M., Ishiguro, M., and Kasahara, M. (2007). Identification by comprehensive chimeric analysis of a key residue responsible for high affinity glucose transport by yeast HXT2. J. Biol. Chem. 282, 13146–13150. doi: 10.1074/jbc.C700041200
Katahira, S., Ito, M., Takema, H., Fujita, Y., Tanino, T., Tanaka, T., et al. (2008). Improvement of ethanol productivity during xylose and glucose co-fermentation by xylose-assimilating S. cerevisiae via expression of glucose transporter Sut1. Enzyme Microb. Technol. 43, 115–119. doi: 10.1016/j.enzmictec.2008.03.001
Kim, H., Lee, H.-S., Park, H., Lee, D.-H., Boles, E., Chung, D., et al. (2017). Enhanced production of xylitol from xylose by expression of Bacillus subtilis arabinose: H + symporter and Scheffersomyces stipitis xylose reductase in recombinant Saccharomyces cerevisiae. Enzyme Microb. Technol. 107, 7–14. doi: 10.1016/j.enzmictec.2017.07.014
Kim, J.-H., Brachet, V., Moriya, H., and Johnston, M. (2006). Integration of transcriptional and posttranslational regulation in a glucose signal transduction pathway in Saccharomyces cerevisiae. Eukaryot. Cell 5, 167–173. doi: 10.1128/EC.5.1.167-173.2006
Kim, J.-H., Polish, J., and Johnston, M. (2003). Specificity and regulation of DNA binding by the yeast glucose transporter gene repressor Rgt1. Mol. Cell. Biol. 23, 5208–5216. doi: 10.1128/MCB.23.15.5208-5216.2003
Knoshaug, E. P., Vidgren, V., Magalhães, F., Jarvis, E. E., Franden, M. A., Zhang, M., et al. (2015). Novel transporters from Kluyveromyces marxianus and Pichia guilliermondii expressed in Saccharomyces cerevisiae enable growth on l -arabinose and d -xylose. Yeast 32, 615–628. doi: 10.1002/yea.3084
Kotter, P., and Ciriacy, M. (1993). Xylose fermentation by Saccharomyces cerevisiae. Appl. Microbiol. Biotechnol. 38, 776–783. doi: 10.1007/BF00167144
Kou, S. C., Christensen, M. S., and Cirillo, V. P. (1970). Galactose transport in Saccharomyces cerevisiae. II. Characteristics of galactose uptake and exchange in galactokinaseless cells. J. Bacteriol. 103, 671–678.
Krampe, S., Stamm, O., Hollenberg, C. P., and Boles, E. (1998). Catabolite inactivation of the high-affinity hexose transporters Hxt6 and Hxt7 of Saccharomyces cerevisiae occurs in the vacuole after internalization by endocytosis. FEBS Lett. 441, 343–347. doi: 10.1016/S0014-5793(98)01583-X
Kruckeberg, A. L. (1996). The hexose transporter family of Saccharomyces cerevisiae. Arch. Microbiol. 166, 283–292. doi: 10.1007/s002030050385
Kruckeberg, A. L., Ye, L., Berden, J. A., and van Dam, K. (1999). Functional expression, quantification and cellular localization of the Hxt2 hexose transporter of Saccharomyces cerevisiae tagged with the green fluorescent protein. Biochem. J. 339(Pt 2), 299–307. doi: 10.1042/bj3390299
Kuyper, M., Harhangi, H. R., Stave, A. K., Winkler, A. A., Jetten, M. S., de Laat, W. T., et al. (2003). High-level functional expression of a fungal xylose isomerase: the key to efficient ethanolic fermentation of xylose by Saccharomyces cerevisiae? FEMS Yeast Res. 4, 69–78. doi: 10.1016/S1567-1356(03)00141-7
Kuyper, M., Hartog, M. M., Toirkens, M. J., Almering, M. J., Winkler, A. A., van Dijken, J. P., et al. (2005a). Metabolic engineering of a xylose-isomerase-expressing Saccharomyces cerevisiae strain for rapid anaerobic xylose fermentation. FEMS Yeast Res. 5, 399–409. doi: 10.1016/j.femsyr.2004.09.010
Kuyper, M., Toirkens, M. J., Diderich, J. A., Winkler, A. A., van Dijken, J. P., and Pronk, J. T. (2005b). Evolutionary engineering of mixed-sugar utilization by a xylose-fermenting Saccharomyces cerevisiae strain. FEMS Yeast Res. 5, 925–934. doi: 10.1016/j.femsyr.2005.04.004
Kuyper, M., Winkler, A. A., van Dijken, J. P., and Pronk, J. T. (2004). Minimal metabolic engineering of Saccharomyces cerevisiae for efficient anaerobic xylose fermentation: a proof of principle. FEMS Yeast Res. 4, 655–664. doi: 10.1016/j.femsyr.2004.01.003
Lagunas, R. (1993). Sugar transport in Saccharomyces cerevisiae. FEMS Microbiol. Rev. 10, 229–242. doi: 10.1111/j.1574-6968.1993.tb05869.x
Lane, S., Xu, H., Oh, E. J., Kim, H., Lesmana, A., Jeong, D., et al. (2018). Glucose repression can be alleviated by reducing glucose phosphorylation rate in Saccharomyces cerevisiae. Sci Rep. 8:2613. doi: 10.1038/s41598-018-20804-4
Leandro, M. J., Goncalves, P., and Spencer-Martins, I. (2006). Two glucose/xylose transporter genes from the yeast Candida intermedia: first molecular characterization of a yeast xylose-H+ symporter. Biochem. J. 395, 543–549. doi: 10.1042/BJ20051465
Lee, J. (1997). Biological conversion of lignocellulosic biomass to ethanol. J. Biotechnol. 56, 1–24. doi: 10.1016/S0168-1656(97)00073-4
Lee, M., Rozeboom, H. J., de Waal, P. P., de Jong, R. M., Dudek, H. M., and Janssen, D. B. (2017). Metal dependence of the xylose isomerase from Piromyces sp. E2 explored by activity profiling and protein crystallography. Biochemistry 56, 5991–6005. doi: 10.1021/acs.biochem.7b00777
Li, H., Schmitz, O., and Alper, H. S. (2016). Enabling glucose/xylose co-transport in yeast through the directed evolution of a sugar transporter. Appl. Microbiol. Biotechnol. 100, 10215–10223. doi: 10.1007/s00253-016-7879-8
Li, J., Xu, J., Cai, P., Wang, B., Ma, Y., Benz, J. P., et al. (2015). Functional analysis of two l-arabinose transporters from filamentous fungi reveals promising characteristics for improved pentose utilization in Saccharomyces cerevisiae. Appl. Environ. Microbiol. 81, 4062–4070. doi: 10.1128/AEM.00165-15
Londesborough, J., Richard, P., Valkonen, M., and Viljanen, K. (2014). Effect of C-terminal protein tags on pentitol and L-arabinose transport by Ambrosiozyma monospora Lat1 and Lat2 transporters in Saccharomyces cerevisiae. Appl. Environ. Microbiol. 80, 2737–2745. doi: 10.1128/AEM.04067-13
Lynd, L., Zyl, W., McBride, J., and Laser, M. (2005). Consolidated bioprocessing of cellulosic biomass: an update. Curr. Opin. Biotechnol. 16, 577–583. doi: 10.1016/j.copbio.2005.08.009
Lynd, L. R. (1996). Overview and evaluation of fuel ethanol from cellulosic biomass: technology, economics, the environment, and policy. Annu. Rev. Energy Environ. 21, 403–465. doi: 10.1146/annurev.energy.21.1.403
Maiden, M. C. J., Davis, E. O., Baldwin, S. A., Moore, D. C. M., and Henderson, P. J. F. (1987). Mammalian and bacterial sugar transport proteins are homologous. Nature 325, 641–643. doi: 10.1038/325641a0
Matsushika, A., Watanabe, S., Kodaki, T., Makino, K., Inoue, H., Murakami, K., et al. (2008). Expression of protein engineered NADP+-dependent xylitol dehydrogenase increases ethanol production from xylose in recombinant Saccharomyces cerevisiae. Appl. Microbiol. Biotechnol. 81, 243–255. doi: 10.1007/s00253-008-1649-1
Moyses, D., Reis, V., Almeida, J., Moraes, L., and Torres, F. (2016). Xylose Fermentation by Saccharomyces cerevisiae: challenges and prospects. Int. J. Mol. Sci. 17:207. doi: 10.3390/ijms17030207
Nehlin, J. O., and Ronne, H. (1990). Yeast MIG1 repressor is related to the mammalian early growth response and Wilms' tumour finger proteins. EMBO J. 9, 2891–2898. doi: 10.1002/j.1460-2075.1990.tb07479.x
Nijland, J. G., Shin, H. Y., Boender, L. G. M., de Waal, P. P., Klaassen, P., and Driessen, A. J. M. (2017). Improved xylose metabolism by a CYC8 mutant of Saccharomyces cerevisiae. Appl. Environ. Microbiol. 83, e00095–e00017. doi: 10.1128/AEM.00095-17
Nijland, J. G., Shin, H. Y., de Jong, R. M., de Waal, P. P., Klaassen, P., and Driessen, A. J. (2014). Engineering of an endogenous hexose transporter into a specific D-xylose transporter facilitates glucose-xylose co-consumption in Saccharomyces cerevisiae. Biotechnol. Biofuels 7:168. doi: 10.1186/s13068-014-0168-9
Nijland, J. G., Shin, H. Y., de Waal, P. P., Klaassen, P., and Driessen, A. J. M. (2018). Increased xylose affinity of Hxt2 through gene shuffling of hexose transporters in Saccharomyces cerevisiae. J. Appl. Microbiol. 124, 503–510. doi: 10.1111/jam.13670
Nijland, J. G., Vos, E., Shin, H. Y., de Waal, P. P., Klaassen, P., and Driessen, A. J. M. (2016). Improving pentose fermentation by preventing ubiquitination of hexose transporters in Saccharomyces cerevisiae. Biotechnol. Biofuels 9:158. doi: 10.1186/s13068-016-0573-3
Nikko, E., and Pelham, H. R. B. (2009). Arrestin-mediated endocytosis of yeast plasma membrane transporters. Traffic 10, 1856–1867. doi: 10.1111/j.1600-0854.2009.00990.x
Olofsson, K., Runquist, D., Hahn-Hägerdal, B., and Lidén, G. (2011). A mutated xylose reductase increases bioethanol production more than a glucose/xylose facilitator in simultaneous fermentation and co-fermentation of wheat straw. AMB Express 1:4. doi: 10.1186/2191-0855-1-4
Ozcan, S., and Johnston, M. (1995). Three different regulatory mechanisms enable yeast hexose transporter (HXT) genes to be induced by different levels of glucose. Mol. Cell. Biol. 15, 1564–1572. doi: 10.1128/MCB.15.3.1564
Ozcan, S., and Johnston, M. (1999). Function and regulation of yeast hexose transporters. Microbiol. Mol. Biol. Rev. 63, 554–569.
Pao, S. S., Paulsen, I. T., and Saier, M. H. (1998). Major facilitator superfamily. Microbiol. Mol. Biol. Rev. 62, 1–34.
Papapetridis, I., Goudriaan, M., Vázquez Vitali, M., de Keijzer, N. A., van den Broek, M., van Maris, A. J. A., et al. (2018). Optimizing anaerobic growth rate and fermentation kinetics in Saccharomyces cerevisiae strains expressing Calvin-cycle enzymes for improved ethanol yield. Biotechnol. Biofuels 11:17. doi: 10.1186/s13068-017-1001-z
Reider Apel, A., Ouellet, M., Szmidt-Middleton, H., Keasling, J. D., and Mukhopadhyay, A. (2016). Evolved hexose transporter enhances xylose uptake and glucose/xylose co-utilization in Saccharomyces cerevisiae. Sci. Rep. 6:19512. doi: 10.1038/srep19512
Reifenberger, E., Boles, E., and Ciriacy, M. (1997). Kinetic characterization of individual hexose transporters of Saccharomyces cerevisiae and their relation to the triggering mechanisms of glucose repression. Eur. J. Biochem. 245, 324–333. doi: 10.1111/j.1432-1033.1997.00324.x
Reifenberger, E., Freidel, K., and Ciriacy, M. (1995). Identification of novel HXT genes in Saccharomyces cerevisiae reveals the impact of individual hexose transporters on glycolytic flux. Mol. Microbiol. 16, 157–167. doi: 10.1111/j.1365-2958.1995.tb02400.x
Reznicek, O., Facey, S. J., de Waal, P. P., Teunissen, A. W. R. H., de Bont, J. A. M., Nijland, J. G., et al. (2015). Improved xylose uptake in Saccharomyces cerevisiae due to directed evolution of galactose permease Gal2 for sugar co-consumption. J. Appl. Microbiol. 119, 99–111. doi: 10.1111/jam.12825
Richard, P., Verho, R., Putkonen, M., Londesborough, J., and Penttilä, M. (2003). Production of ethanol from L-arabinose by Saccharomyces cerevisiae containing a fungal L-arabinose pathway. FEMS Yeast Res. 3, 185–189. doi: 10.1016/S1567-1356(02)00184-8
Roy, A., Kim, Y.-B., Cho, K. H., and Kim, J.-H. (2014). Glucose starvation-induced turnover of the yeast glucose transporter Hxt1. Biochim. Biophys. Acta 1840, 2878–2885. doi: 10.1016/j.bbagen.2014.05.004
Roy, A., Shin, Y. J., Cho, K. H., and Kim, J.-H. (2013). Mth1 regulates the interaction between the Rgt1 repressor and the Ssn6-Tup1 corepressor complex by modulating PKA-dependent phosphorylation of Rgt1. Mol. Biol. Cell 24, 1493–1503. doi: 10.1091/mbc.e13-01-0047
Runquist, D., Fonseca, C., Rådström, P., Spencer-Martins, I., and Hahn-Hägerdal, B. (2009). Expression of the Gxf1 transporter from Candida intermedia improves fermentation performance in recombinant xylose-utilizing Saccharomyces cerevisiae. Appl. Microbiol. Biotechnol. 82, 123–130. doi: 10.1007/s00253-008-1773-y
Runquist, D., Hahn-Hagerdal, B., and Radstrom, P. (2010). Comparison of heterologous xylose transporters in recombinant Saccharomyces cerevisiae. Biotechnol. Biofuels 3:5. doi: 10.1186/1754-6834-3-5
Saloheimo, A., Rauta, J., Stasyk, O. V., Sibirny, A. A., Penttila, M., and Ruohonen, L. (2007). Xylose transport studies with xylose-utilizing Saccharomyces cerevisiae strains expressing heterologous and homologous permeases. Appl. Microbiol. Biotechnol. 74, 1041–1052. doi: 10.1007/s00253-006-0747-1
Sedlak, M., and Ho, N. W. (2001). Expression of E. coli araBAD operon encoding enzymes for metabolizing L-arabinose in Saccharomyces cerevisiae. Enzyme Microb. Technol. 28, 16–24. doi: 10.1016/S0141-0229(00)00282-9
Sen, A., Acosta-Sampson, L., Alvaro, C. G., Ahn, J. S., Cate, J. H. D., and Thorner, J. (2016). Internalization of heterologous sugar transporters by endogenous α-arrestins in the yeast Saccharomyces cerevisiae. Appl. Environ. Microbiol. 82, 7074–7085. doi: 10.1128/AEM.02148-16
Shin, H. Y., Nijland, J. G., de Waal, P. P., de Jong, R. M., Klaassen, P., and Driessen, A. J. M. (2015). An engineered cryptic Hxt11 sugar transporter facilitates glucose–xylose co-consumption in Saccharomyces cerevisiae. Biotechnol. Biofuels 8:176. doi: 10.1186/s13068-015-0360-6
Shin, H. Y., Nijland, J. G., de Waal, P. P., and Driessen, A. J. M. (2017). The amino-terminal tail of Hxt11 confers membrane stability to the Hxt2 sugar transporter and improves Xylose fermentation in the presence of acetic acid. Biotechnol. Bioeng. 114, 1937–1945. doi: 10.1002/bit.26322
Snowdon, C., and van der Merwe, G. (2012). Regulation of Hxt3 and Hxt7 turnover converges on the Vid30 complex and requires inactivation of the Ras/cAMP/PKA pathway in Saccharomyces cerevisiae. PLoS ONE 7:e50458. doi: 10.1371/journal.pone.0050458
Solomon, B. D. (2010). Biofuels and sustainability. Ann. N. Y. Acad. Sci. 1185, 119–134. doi: 10.1111/j.1749-6632.2009.05279.x
Steffensen, L., and Pedersen, P. A. (2006). Heterologous expression of membrane and soluble proteins derepresses GCN4 mRNA translation in the yeast Saccharomyces cerevisiae. Eukaryot. Cell 5, 248–261. doi: 10.1128/EC.5.2.248-261.2006
Subtil, T., and Boles, E. (2011). Improving L-arabinose utilization of pentose fermenting Saccharomyces cerevisiae cells by heterologous expression of L-arabinose transporting sugar transporters. Biotechnol. Biofuels 4:38. doi: 10.1186/1754-6834-4-38
Subtil, T., and Boles, E. (2012). Competition between pentoses and glucose during uptake and catabolism in recombinant Saccharomyces cerevisiae. Biotechnol. Biofuels 5:14. doi: 10.1186/1754-6834-5-14
Sun, L., Zeng, X., Yan, C., Sun, X., Gong, X., Rao, Y., et al. (2012). Crystal structure of a bacterial homologue of glucose transporters GLUT1-4. Nature 490, 361–366. doi: 10.1038/nature11524
Tanino, T., Ito, T., Ogino, C., Ohmura, N., Ohshima, T., and Kondo, A. (2012). Sugar consumption and ethanol fermentation by transporter-overexpressed xylose-metabolizing Saccharomyces cerevisiae harboring a xyloseisomerase pathway. J. Biosci. Bioeng. 114, 209–211. doi: 10.1016/j.jbiosc.2012.03.004
Tantirungkij, M., Seki, T., and Yoshida, T. (1994). Genetic improvement of Saccharomyces cerevisiae for ethanol production from xylose. Ann. N. Y. Acad. Sci. 721, 138–147. doi: 10.1111/j.1749-6632.1994.tb47386.x
Traff, K. L., Otero Cordero, R. R., van Zyl, W. H., and Hahn-Hagerdal, B. (2001). Deletion of the GRE3 aldose reductase gene and its influence on xylose metabolism in recombinant strains of Saccharomyces cerevisiae expressing the xylA and XKS1 genes. Appl. Environ. Microbiol. 67, 5668–5674. doi: 10.1128/AEM.67.12.5668-5674.2001
Truernit, E., Stadler, R., Baier, K., and Sauer, N. (1999). A male gametophyte-specific monosaccharide transporter inArabidopsis. Plant J. 17, 191–201. doi: 10.1046/j.1365-313X.1999.00372.x
van Zyl, W. H., Eliasson, A., Hobley, T., and Hahn-Hägerdal, B. (1999). Xylose utilisation by recombinant strains of Saccharomyces cerevisiae on different carbon sources. Appl. Microbiol. Biotechnol. 52, 829–833. doi: 10.1007/s002530051599
Vardy, E., Arkin, I. T., Gottschalk, K. E., Kaback, H. R., and Schuldiner, S. (2004). Structural conservation in the major facilitator superfamily as revealed by comparative modeling. Protein Sci. 13, 1832–1840. doi: 10.1110/ps.04657704
Verho, R., Londesborough, J., Penttilä, M., and Richard, P. (2003). Engineering redox cofactor regeneration for improved pentose fermentation in Saccharomyces cerevisiae. Appl. Environ. Microbiol. 69, 5892–5897. doi: 10.1128/AEM.69.10.5892-5897.2003
Verho, R., Penttilä, M., and Richard, P. (2011). Cloning of Two Genes (LAT1,2) Encoding specific l-arabinose transporters of the l-arabinose fermenting yeast Ambrosiozyma monospora. Appl. Biochem. Biotechnol. 164, 604–611. doi: 10.1007/s12010-011-9161-y
Verhoeven, M. D., Bracher, J. M., Nijland, J. G., Bouwknegt, J., Daran, J.-M. G., Driessen, A. J. M., et al. (2018). Laboratory evolution of a glucose-phosphorylation-deficient, arabinose-fermenting S. cerevisiae strain reveals mutations in GAL2 that enable glucose-insensitive l-arabinose uptake. FEMS Yeast Res. 18. doi: 10.1093/femsyr/foy062
Verhoeven, M. D., Lee, M., Kamoen, L., van den Broek, M., Janssen, D. B., Daran, J.-M. G., et al. (2017). Mutations in PMR1 stimulate xylose isomerase activity and anaerobic growth on xylose of engineered Saccharomyces cerevisiae by influencing manganese homeostasis. Sci. Rep. 7, 46155. doi: 10.1038/srep46155
Verwaal, R., Paalman, J. W. G., Hogenkamp, A., Verkleij, A. J., Verrips, C. T., and Boonstra, J. (2002). HXT5 expression is determined by growth rates in Saccharomyces cerevisiae. Yeast 19, 1029–1038. doi: 10.1002/yea.895
Wang, C., Bao, X., Li, Y., Jiao, C., Hou, J., Zhang, Q., et al. (2015). Cloning and characterization of heterologous transporters in Saccharomyces cerevisiae and identification of important amino acids for xylose utilization. Metab. Eng. 30, 79–88. doi: 10.1016/j.ymben.2015.04.007
Wang, C., Li, Y., Qiu, C., Wang, S., Ma, J., Shen, Y., et al. (2017). Identification of important amino acids in Gal2p for improving the L-arabinose transport and metabolism in Saccharomyces cerevisiae. Front. Microbiol. 8:1391. doi: 10.3389/fmicb.2017.01391
Wang, C., Shen, Y., Zhang, Y., Suo, F., Hou, J., and Bao, X. (2013). Improvement of L-arabinose fermentation by modifying the metabolic pathway and transport in Saccharomyces cerevisiae. Biomed Res. Int. 2013:461204. doi: 10.1155/2013/461204
Watanabe, S., Abu Saleh, A., Pack, S. P., Annaluru, N., Kodaki, T., and Makino, K. (2007a). Ethanol production from xylose by recombinant Saccharomyces cerevisiae expressing protein-engineered NADH-preferring xylose reductase from Pichia stipitis. Microbiology 153, 3044–3054. doi: 10.1099/mic.0.2007/007856-0
Watanabe, S., Saleh, A. A., Pack, S. P., Annaluru, N., Kodaki, T., and Makino, K. (2007b). Ethanol production from xylose by recombinant Saccharomyces cerevisiae expressing protein engineered NADP+-dependent xylitol dehydrogenase. J. Biotechnol. 130, 316–319. doi: 10.1016/j.jbiotec.2007.04.019
Weierstall, T., Hollenberg, C. P., and Boles, E. (1999). Cloning and characterization of three genes (SUT1-3) encoding glucose transporters of the yeast Pichia stipitis. Mol. Microbiol. 31, 871–883. doi: 10.1046/j.1365-2958.1999.01224.x
Wieczorke, R., Krampe, S., Weierstall, T., Freidel, K., Hollenberg, C. P., and Boles, E. (1999). Concurrent knock-out of at least 20 transporter genes is required to block uptake of hexoses in Saccharomyces cerevisiae. FEBS Lett. 464, 123–128. doi: 10.1016/S0014-5793(99)01698-1
Wisselink, H. W., Toirkens, M. J., del Rosario Franco Berriel, M., Winkler, A. A., van Dijken, J. P., Pronk, J. T., et al. (2007). Engineering of Saccharomyces cerevisiae for Efficient Anaerobic Alcoholic Fermentation of L-Arabinose. Appl. Environ. Microbiol. 73, 4881–4891. doi: 10.1128/AEM.00177-07
Wisselink, H. W., Toirkens, M. J., Wu, Q., Pronk, J. T., and van Maris, A. J. (2009). Novel evolutionary engineering approach for accelerated utilization of glucose, xylose, and arabinose mixtures by engineered Saccharomyces cerevisiae strains. Appl. Environ. Microbiol. 75, 907–914. doi: 10.1128/AEM.02268-08
Wisselink, H. W., van Maris, A. J. A., Pronk, J. T., Klaasen, P., and De Jong, R. M. (2012). Polypeptides With Permease Activity. Patent WO/2012/049173. WIPO/PCT.
Young, E., Poucher, A., Comer, A., Bailey, A., and Alper, H. (2011). Functional survey for heterologous sugar transport proteins, using Saccharomyces cerevisiae as a host. Appl. Environ. Microbiol. 77, 3311–3319. doi: 10.1128/AEM.02651-10
Young, E. M., Comer, A. D., Huang, H., and Alper, H. S. (2012). A molecular transporter engineering approach to improving xylose catabolism in Saccharomyces cerevisiae. Metab. Eng. 14, 401–411. doi: 10.1016/j.ymben.2012.03.004
Young, E. M., Tong, A., Bui, H., Spofford, C., and Alper, H. S. (2014). Rewiring yeast sugar transporter preference through modifying a conserved protein motif. Proc. Natl. Acad. Sci. U.S.A. 111, 131–136. doi: 10.1073/pnas.1311970111
Zaldivar, J., Nielsen, J., Olsson, L., Arensdorf, J. J., Loomis, A. K., DiGrazia, P. M., et al. (2002). Fuel ethanol production from lignocellulose: a challenge for metabolic engineering and process integration. Appl. Environ. Microbiol. 68, 691–698. doi: 10.1007/s002530100624
Zhou, H., Cheng, J., sheng, Wang, B. L., Fink, G. R., and Stephanopoulos, G. (2012). Xylose isomerase overexpression along with engineering of the pentose phosphate pathway and evolutionary engineering enable rapid xylose utilization and ethanol production by Saccharomyces cerevisiae. Metab. Eng. 14, 611–622. doi: 10.1016/j.ymben.2012.07.011
Keywords: pentose transport, D-xylose, L-arabinose, yeast, bioethanol
Citation: Nijland JG and Driessen AJM (2020) Engineering of Pentose Transport in Saccharomyces cerevisiae for Biotechnological Applications. Front. Bioeng. Biotechnol. 7:464. doi: 10.3389/fbioe.2019.00464
Received: 14 November 2019; Accepted: 19 December 2019;
Published: 29 January 2020.
Edited by:
Ilja Kusters, Harvard Medical School, United StatesReviewed by:
Yu Shen, Shandong University, ChinaEckhard Boles, Goethe University Frankfurt, Germany
Copyright © 2020 Nijland and Driessen. This is an open-access article distributed under the terms of the Creative Commons Attribution License (CC BY). The use, distribution or reproduction in other forums is permitted, provided the original author(s) and the copyright owner(s) are credited and that the original publication in this journal is cited, in accordance with accepted academic practice. No use, distribution or reproduction is permitted which does not comply with these terms.
*Correspondence: Arnold J. M. Driessen, YS5qLm0uZHJpZXNzZW5AcnVnLm5s