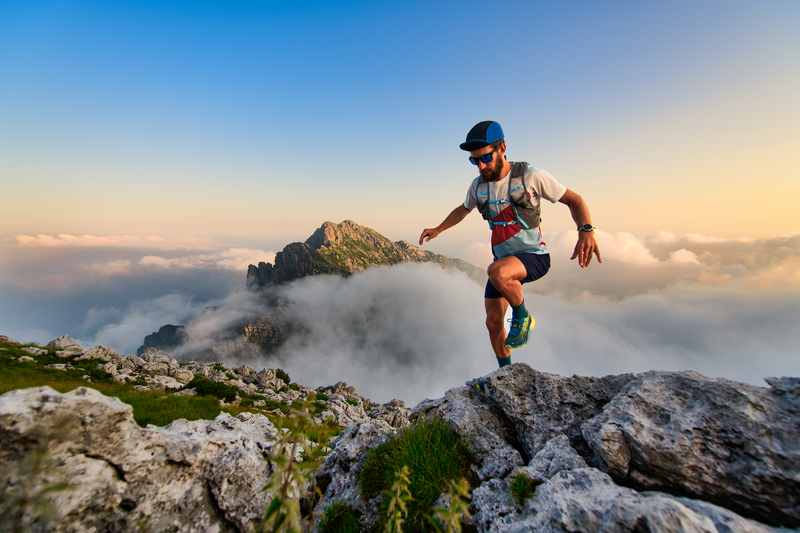
95% of researchers rate our articles as excellent or good
Learn more about the work of our research integrity team to safeguard the quality of each article we publish.
Find out more
REVIEW article
Front. Bioeng. Biotechnol. , 28 January 2020
Sec. Synthetic Biology
Volume 7 - 2019 | https://doi.org/10.3389/fbioe.2019.00459
This article is part of the Research Topic 2nd Synthetic Biology Forum: System and Synthetic Biology for Biofuels and Bioproducts View all 20 articles
Industrial biology plays a crucial role in the fields of medicine, health, food, energy, and so on. However, the lack of efficient genetic engineering tools has restricted the rapid development of industrial biology. Recently, the emergence of clustered regularly interspaced short palindromic repeats/CRISPR-associated protein 9 (CRISPR/Cas9) system brought a breakthrough in genome editing technologies due to its high orthogonality, versatility, and efficiency. In this review, we summarized the barriers of CRISPR/Cas9 and corresponding solutions for efficient genetic engineering in industrial microorganisms. In addition, the advances of industrial biology employing the CRISPR/Cas9 system were compared in terms of its application in bacteria, yeast, and filamentous fungi. Furthermore, the cooperation between CRISPR/Cas9 and synthetic biology was discussed to help build complex and programmable gene circuits, which can be used in industrial biotechnology.
Industrial biotechnology has advanced significantly in recent years due to the improvement of genomic engineering tools. Genetic engineering is a complex technology that manipulates genes at molecular level. The recombined exogenous genes can be replicated, transcribed, translated, and expressed in receptor cells to produce various valuable chemicals. During the long-time evolution of microorganisms, a unique adaptive immune system, named as clustered regularly interspaced short palindromic repeat sequences and CRISPR-associated protein 9 (CRISPR/Cas9), was employed by bacteria and archaea to defend against foreign-invading DNA (Figure 1; Horvath and Barrangou, 2010). This system is consisted of a Cas9 nuclease, a target-recognizing CRISPR RNA (crRNA), and auxiliary non-coding trans-activating crRNAs (tracrRNAs) (Jiang and Doudna, 2017). The precursor crRNA (pre-crRNA) is able to combine with several tracrRNAs, and can be recognized and processed by RNase III into mature crRNA::tracrRNAs (dual RNA hybrid). A single-guide RNA (sgRNA) can be constructed by fusing a crRNA containing the targeting guide sequence to a tracrRNA and then combines to Cas9 protein, generate DNA double-strand breaks (DSBs), and then alter the target gene by cellular DNA repair mechanisms (Cong et al., 2013). The DSBs can be repaired through two different ways: non-homologous end-joining (NHEJ) and homologous repair (HR) (Capecchi, 1989). NHEJ can introduce insertion and deletion at the target site. HR uses a foreign DNA donor template to recombine with the target site for introducing a specific point mutation or insertion of the desired sequence.
Figure 1. The CRISPR-Cas9 mediated adaptive immunity is divided into three phases: Adaptation, Expression, and Interference. In the adaptation phase, (A) the host is invaded by phage DNA during infection. (B) Subsequently, the invading DNA is processed by various Cas genes into small DNA fragments (protospacer). The selection of protospacer depends in part on the specific recognition of protospacer adjacent motif (PAM) present within the viral genome. (C) The small DNA fragments is then incorporated into the CRISPR locus of the bacterial genome as a new spacer, flanked by a repeat sequence. In the expression phase, (D) the CRISPR locus is transcribed into a long precursor CRISPR RNA (pre-crRNA). (E) Then, the tracrRNA combine to pre-crRNA to a dual RNA hybrid, this dual RNA hybrid will be recognized and cut by RNase III with the existence of Cas9 protein, resulting in mature crRNA. (F) The mature crRNA will combine to Cas9 protein and guide the DNA cleavage. In the Interference phase, when phage DNA invades again, the Cas9:crRNA complex identifies a sequence that is complementary to the spacer and adjacent to the PAM. (I) Finally, the invading DNA is cleaved by Cas9 protein to prevent infection.
Previous methods of manufacturing targeted DSBs relied on protein–DNA recognition systems, such as zinc-finger nucleases (ZFNs) and transcription activator-like effector nucleases (TALENs). However, these systems were limited due to their complex and expensive operation. On the contrary, the CRISPR/Cas9 system has been widely employed in various fields owing to its high efficiency, low cost, and convenience (Gaj et al., 2013; Hsu et al., 2014). Hence, in this review, the potential of CRISPR/Cas9 in industrial biotechnology was demonstrated by introducing its applications in bacteria, yeasts, and filamentous fungi. The future prospect of cooperation between CRISPR/Cas9 and synthetic biology to build complex and programmable gene circuits was also summarized.
Although the CRISPR/Cas9 system has been successfully used in bacteria, yeasts, and fungi, its gene editing efficiency is still unsatisfactory. How to improve the gene editing efficiency has been the focus in this filed. Several strategies employed to improve genome editing efficiency with CRISPR/Cas9 were accordingly summarized.
The mechanism of gene editing is repairing the DSBs generated by CRISPR/Cas9 through NHEJ or HR. Once DSBs occurs, most industrial microorganisms prefer the NHEJ pathway over HR even with exogenous donors, which retards the precise genome editing. In order to increase the frequency of HR, two main strategies were employed: (1) coupling the CRISPR/Cas9 system to lambda Red oligonucleotide recombineering and (2) deleting KU70 or KU80 heterodimer involved in NHEJ repair. For instance, Jiang et al. established a two-plasmid-based CRISPR/Cas9 system in Escherichia coli, in which Streptococcus pyogenes Cas9 and crRNA array were expressed in the low-copy plasmid (pCas) and high-copy plasmid (pCRISPR) series (Jiang et al., 2013; Mali et al., 2013). Although this novel genetic engineering tool had a better performance than did the traditional one, it still needed further modifications to obtain higher efficiency. Based on this system, a triple-plasmid strategy was designed with the third plasmid carrying the λ-Red genes expressed from ParaB. In contrast, this three-plasmid system increased the percentage of mutant cells from 19 to 65%. In another study, a CRISPR/Cas9 system, which had 94% efficiency toward single-gene non-sense mutations, was accordingly established in Komagataella pastoris. However, the integration efficiency was really low (2%), when a donor template with 1-kbp homologous arms was provided (Weninger et al., 2016). To improve the integration efficiency with markerless donor cassettes, the KU70 gene was accordingly knocked out and improved the knock-in efficiency up to nearly 100% (Weninger et al., 2018).
In most studies, Cas9 protein and gRNA were separated into independent vectors. The Cas9 protein was commonly expressed in a low-copy plasmid with constitutive promoters, because high-level expression of Cas9 will lead to negative influence on microbial growth. In contrast, the expression of gRNA should choose high-copy plasmids with a strong promoter. The RNA polymerase III (pol III) promoters had been successfully employed in many cases; however, it was difficult to find suitable RNA pol III promoters. Thus, the sgRNA was flanked with two ribozyme sequences, 5′ end hammerhead (HH) and 3′ end hepatitis delta virus (HDV) to express under a strong RNA polymerase II promoter (Figure 2; Nødvig et al., 2015). In addition, synthesized hybrid promoters provide another feasible substitute for gRNA expression (Cai et al., 2019). For instance, the gene editing efficiency by harnessing the common RNA pol III promoter SNR52 to express sgRNA in oleaginous yeast Yarrowia lipolytica only reached 26%. In order to optimize the expression of sgRNA, Schwartz et al. constructed an RNA polymerase II (Pol II) TEF promoter for sgRNA with HH and HDV ribozymes in 5′ end and 3′ end, and fused the Pol III promoters RPR1, SCR1, and SNR52 with a glycine tRNA (tRNAGly) (Schwartz et al., 2015). Finally, the highest disruption efficiency of 92% reached with synthetic SCR1′-tRNAGly promoter. In addition, the disruption efficiency using the SNR52′-tRNAGly promoter was improved by 28% than the initial SNR52 promoter.
Figure 2. Schematic to illustrate how gRNA and Cas9 expressed in industrial microorganisms. The gRNA was mainly expressed by two ways. One is directly derived by RNA polymerase III promoters. Another one is flanking the gRNA with HH and HDV ribozymes and then derived by RNA polymerase II promoters. The Cas9 could be expressed by a strong or weak, constitutive, or inducible promoter and fused with nuclear localization sequence (NLS). The two expressing cassettes can be expressed either from the same plasmid, separate plasmids, or integrated into the genome, most time with a donor fragment. So, after the genetic engineering, the industrial microorganisms could be transformed to high-powered microbial cell factories to product valuable production, such as biofuels, chemicals, biocatalysts, food additives, drugs, and so on.
The importance of codon optimization of Cas9 is different in different strains. For example, the natural S. pyogenes Cas9 has shown high targeting efficiency in Saccharomyces cerevisiae (Bao et al., 2015). However, the targeting efficiency was unsatisfactory when using native S. pyogenes Cas9 in K. pastoris. By employing the human codon optimized Cas9 (HsCas9), the targeting efficiency improved from 32 to 73%, indicating that optimization of Cas9 protein codons plays a crucial role in improving the targeting efficiency. Hence, when the performance of the CRISPR/Cas9 system was unsatisfactory, optimization of Cas9 codons may be a good solution.
For industrial microorganisms, the sgRNA binding site is crucial in terms of the targeting efficiency. In order to improve the efficiency, different sgRNAs targeting sites should be tested. For instance, Peng et al. found that the targeting efficiency was distributed between 13 and 100% when using six different sgRNAs targeting six different sites of mepA gene, and the sgRNAs with the GC content under 60% had better performance. This result showed that different sgRNAs had great influence on editing efficiency and adoption of suitable sgRNA is important for a high success rate. A series of website tools had been set for sgRNA design and summarized in previous literature (Chuai et al., 2017).
The targeted chromosomal region could escape sgRNA/Cas9 endonuclease activity in some cases. The effective solution is to prolong the incubation under the appropriate selection pressure to generate iterative DSBs until mutagenic repair occurs. To confirm the effect of prolonged incubation on the mutation efficiency, So et al. introduced two plasmids that contained same sgRNA sequence and different length of homologous arms (30 and 500 bp) to the targeted gene into Bacillus subtilis, respectively (So et al., 2017). The mutation efficiencies were improved from 85% to almost 100% after incubation for 9 h. Results demonstrated that the mutation efficiency can be maximized by prolonging incubation.
Industrial microorganisms can produce various value-added chemicals from low-cost feedstock including renewable biomass and organic wastes (Yao et al., 2018). To enhance the performances of industrial microorganisms, genetic modifications were usually implemented to construct desired microbial cell factories. Although various methods are available for genetic modifications, it is time-consuming and labor-intensive. Fortunately, as a surprising “gift,” the CRISPR/Cas9 system greatly improved the efficiency of genetic engineering. Here, we reviewed the applications of the CRISPR/Cas9 system in bacteria, yeasts, and filamentous fungi with special emphasis on E. coli and S. cerevisiae, which were the most commonly used cell factory.
Escherichia coli is often used to produce a variety of valuable chemicals, drugs, and biofuels in industrial biotechnology. A traditional method of gene knockout in E. coli was to adopt the Red homologous recombination system to mediate the homologous recombination of DNA. However, it is inefficient and especially not suitable for recombination of multiple sites (Murphy and Campellone, 2003). To improve the genetic engineering efficiency, Jiang et al. construct a triple-plasmid system as mentioned above. This novel genetic engineering tool significantly improved the efficiency of genetic modification and thus accelerated the development of industrial biology. In previous studies, Cas9 and gRNA were expressed in two plasmids, respectively, as the simultaneous expression would burden the organism metabolism and cause cell death. Hence, Cas9 or gRNA should be repressed before a genome editing event. Cas9 and gRNA can be assembled into one plasmid containing a pBAD promoter, which is repressed by glucose and induced by arabinose and a temperature-sensitive replicon repA101ts, so that transformed E. coli could grow on glucose-amended plates and be edited under the induction with 2 g/L of arabinose. This fast and easy procedure for genome editing could perform continuously, as multiple loci only required one plasmid construction and one step of transformation. To further improve the simultaneous editing efficiency of multiple loci, a CRISPR/Cas9-assisted multiplex genome editing technique was developed. The CRISPR/Cas9-assisted multiplex genome editing technique contained three plasmids: pRedCas9 containing both λ-Red recombineering and Cas9 system under the control of pBAD promoter, pMgRNAs containing gRNAs, and pDonorDNAs carrying multiple donor DNA cassettes (Feng et al., 2018). In another versatile study, Li et al. firstly coexpressed a plasmid containing a gRNA targeting the bla gene and Cas9 with the λ-Red recombineering system into E. coli (Li et al., 2015). Then, the genetic editing started with cotransformation of donor DNA and gRNA plasmid into preceding cells. Comparing to the previously established system, this optimized system has a higher gene editing efficiency and less operating time, almost 100% for codon replacements and knockout genes within 2 days. It was noteworthy that using a double-strand DNA as a donor template has a better performance than a single-strand DNA in gene deletions. Subsequently, this optimized system was employed to strengthen the MEP pathway by substituting the promoters and ribosome binding sites, inserting a heterologous β-carotene biosynthetic pathway and optimizing the central carbon metabolism (Li et al., 2015). Finally, the best producer yielded 2.0 g/L β-carotene in fed-batch fermentation. This extensive work can hardly be completed without employing CRISPR-based tools, revealing their great potential for efficient and diverse manipulation of genomic DNA. The Cas9-recombineering method was further exploited with the development of the CRISPR-enabled trackable genome engineering (CREATE) tool (Garst et al., 2017). Application of this tool in E. coli allowed for simultaneous transformation of multiple libraries of plasmid-borne recombined templates (Garst et al., 2017). The CREATE strategy was employed to construct genome libraries of isopropanol pathway by introducing multiple ribosome binding site variations in E. coli, leading to the construction and testing of ~1,000 strains in a few days. The best performer reached a titer of 7.1 g/L isopropanol within 24 h (Liang et al., 2017).
Besides the versatile applications in E. coli, the CRISPR-based tools also had satisfactory performances in other bacteria (Table 1). For instance, genetic engineering technologies for solventogenic Clostridium were still immature due to low transformation efficiency, inadequate endogenous homologous recombination, and poorly understood physiology and metabolism (Papoutsakis, 2008; Pyne et al., 2014; Bruder et al., 2016). Recently, CRISPR/Cas9 for Clostridium saccharoperbutylacetonicum N1-4, a hyper-butanol-producing strain, was developed. The genome engineering efficiency was improved from 20 to 75% by selecting optimized promoter PJ23119 from E. coli for gRNA expression. After deleting two essential genes of phosphotransacetylase (PTA) and butyrate kinase (BUK) for acetate and butyrate production, the butanol production reached 19.0 g/L, which is one of the highest levels ever reported from batch fermentations (Wang et al., 2017). This Cas9-based editing tool could be easily adapted for use in closely related microorganisms, paving the way for elucidating the mechanism of solvent production and constructing robust strains with desirable butanol-producing features. In addition, the Cas9-based editing tools have also been successfully employed for the production of bulk chemicals, such as succinate in Synechococcus elongatus (Li et al., 2016), isopropanol-butanol-ethanol in Clostridium acetobutylicum (Wasels et al., 2017), and γ-amino-butyric acid (GABA) in Corynebacterium glutamicum (Cho et al., 2017). The wide application of the CRISPR/Cas9 system in a variety of bacteria genera demonstrates that it plays a critical role in the prosperous development of bioindustrial.
Yeasts play critical roles in industrial biology, as a wide range of products can be produced by yeasts, including biopharmaceuticals, biocatalysts, food additives, fine chemicals, and renewable biofuels (Raschmanova et al., 2018). Due to their robust physiology, yeasts could be cultivated in harsh growth conditions, such as low pH and elevated temperatures. Furthermore, yeasts can be introduced in complex eukaryotic post-translational modification systems, which are absent in bacterial hosts and often vital for biopharmaceuticals production (Thomas et al., 2013). Until now, a series of genetic engineering tools based on CRISPR/Cas9 had been exploited in yeast to improve the efficiency of genetic modification (Mitsui et al., 2019).
For decades, S. cerevisiae has been a well-known model organism in research and application areas (Jakočiunas et al., 2016). To verify the efficiency of the CRISPR/Cas9 system in S. cerevisiae, the endogenous genomic negative selectable marker CAN1 (encoding arginine permease) can be chosen as a target gene (Dicarlo et al., 2013). To further improve the gene knockout efficiency, 90-bp double-strand oligonucleotides (dsOligo) including homologous arms to the target site and an internal stop codon as the HR template were designed. The recombined frequency of mutations selected from the medium containing canavanine (a toxic arginine analog that can kill cells containing functional CAN1 gene) was almost 100%, providing foundations for simple and powerful genome engineering tools in yeasts. This system can be used to knock out LEU2, TRP1, URA3, and HIS3 in polyploid industrial yeast S. cerevisiae ATCC 4124 with efficiency of up to 60%, and a quadruple-deficient strain (Δura3, Δtrp1, Δleu2, Δhis3) was successfully constructed (Zhang et al., 2014). In order to further improve the genetic editing efficiency of the CRISPR/Cas9 system, the USER cloning technology was accordingly employed to assemble multiple sgRNAs in one plasmid for efficient gene disruption and promoter engineering of one to five target loci in one step (Jakočiunas et al., 2015a). This one-step maker-free genome editing approach achieved high efficiencies of 50–100% from single to quintuple edits. However, the low efficiency of cotransformation of gRNA plasmids and corresponding HR donors hindered large-scale genome engineering applications (Lian et al., 2018). To solve this problem, a homology-integrated CRISPR (HI-CRISPR) system by fusing a 100-bp HR template to the 5′ end of the crRNA sequences was constructed, leading to 87% efficiency of multiplex knockout of CAN1, ADE2, and LYP1 (Bao et al., 2015). This HI-CRISPR system further improved the efficiency of multiplex genome editing and genome-scale engineering.
In addition to gene knockout, the CRISPR/Cas9 system can also mediate gene insertion using the homologous arm of donor DNA to the target gene. To better control cellular levels of correctly folding sgRNA, HDV ribozyme was fused to the 5′ end of sgRNA to protect the 5′ end of the sgRNA from 5′ exonucleases. This HDV-gRNA expression strategy significantly increased the efficiency of multiplex genome editing in diploid yeast strains, in which the heterologous cellodextrin transporter (cdt-1) and endogenous β-glucosidase (gh1-1) were inserted. As a result, the efficiency of utilizing cellobiose was increased by 10 times through site-directed mutagenesis of cdt-1 and gh1-1 genes via the multiplexed CRISPR/Cas9 system (Ryan et al., 2014). Meanwhile, Ronda et al. cotransformed one episomal vector expressing three gRNAs with three donor DNAs containing β-carotene synthesis genes of BTS1, crtYB, and crtI into S. cerevisiae, enabling it to synthesize β-carotene (Ronda et al., 2015). Similarly, three exogenous genes (XYL1, XYL2, and XYL3) encoding for xylose reductase, xylitol dehydrogenase, and xylulokinase from Scheffersomyces stipites were integrated into the loci of PHO13 and ALD6 in S. cerevisiae by employing the CRISPR/Cas9 system (Tsai et al., 2015). The refactored strains achieved the ability of utilizing xylose and could be readily used for large-scale fermentations, as no antibiotic-resistant markers were adopted. In addition, a more versatile genome engineering tool, Cas9-facilitated multiloci integration of in vivo assembled DNA parts (CasEMBLR), was constructed by combining DNA assembly, HR of DSBs using donor DNAs, and multiplex gRNA expression cassettes (Jakočiunas et al., 2015b). As a proof of concept, this CasEMBLR was employed to assemble and integrate the gene expression cassettes (upstream homology arm, promoter, structural gene, terminator, and downstream homology arm) of CrtYB, CrtI, and CrtE into the loci of ADE2, HIS3, and URA3, with a marker-free engineering efficiency of 31%.
CRISPR/Cas9 was also implemented in many other industrial yeasts (Table 2). For example, to construct more suitable promoters for sgRNA in Schizosaccharomyces pombe, Jacobs et al. constructed an expression cassette by adding the leader RNA from rrk1 gene between the promoter and sgRNA sequence, and fused the HH ribozymes with 3′ end of the mature sgRNA to achieve correct 3′ sgRNA processing. This system achieved a high efficiency of 98% when a donor template was cotransformed. To further simplify the operation of the CRISPR/Cas9 system in S. pombe, Zhang et al. developed a cloning-free procedure including a gapped Cas9-encoding plasmid and a PCR-amplified sgRNA insert. Ura4 and rrk1 promoter-leader was just between the gap of Cas9-encoding plasmid. The PCR-amplified sgRNA insert was consisted of sgRNA target sequence and scaffold, and flanked with the homologous arms of ura4 and promoter-leader. Accordingly, a circular plasmid including Cas9 and sgRNA could generate two gap-repairing fragments. This cloning-free procedure could change the sgRNA target sequence only using an 83-bp sgRNA premier instead of cloning the whole sgRNA plasmid.
Due to the considerable economic value of their metabolites, filamentous fungi have been applied to produce antibiotics, organic acids, pigments, polyunsaturated fatty acids, and so on (Dufossé et al., 2014; Ji et al., 2014; Xu X. et al., 2015). However, challenges of delivery through fungal cell wall and lack of available promoters and plasmids hindered the development of genetic engineering tools in filamentous fungi. In addition, the low editing efficiency and consequent large amount of labor time impeded the further application of filamentous fungi in the industry. The emerged CRISPR/Cas9 has brought a breakthrough for genetic manipulation in filamentous fungi.
Conventional genetic engineering strategies to improve the efficiency of HR were to delete KU70 or KU80 heterodimer (Weld et al., 2006). However, if KU70 or KU80 is interrupted, the filamentous fungi will become more sensitive to growth environments with specific requirements for some chemicals, such as phleomycin, bleomycin, and methyl/ethyl methanesulfonate (Liu et al., 2015). To overcome this obstacle, the CRISPR/Cas9 system in the filamentous fungus of Trichoderma reesei using specific optimized codon and in vitro RNA transcription was established (Hao and Su, 2019). The highest frequency of single HR in T. reesei using a pair of ≥600-bp homology arms was almost 100%. Subsequently, a microhomology-mediated end-joining system based on CRISPR/Cas9 in Aspergillus fumigatus was also established by flanking the sgRNA with HH and HDV. This system achieved accurate target gene editing with a high efficiency of 95–100% via very short (~35-bp) homology arms, indicating that it can function as a powerful and versatile genome editing tool in A. fumigatus (Zhang et al., 2016). In addition to the successful application in different species of Aspergillus, this evolved system has also been employed in several different filamentous fungal species, broadening the application of the CRISPR/Cas9 system (Hao and Xia, 2015; Weber et al., 2016; Weyda et al., 2017).
Taken together, the CRISPR/Cas9-mediated gene editing technology not only efficiently edited individual target gene but also showed satisfactory performances in multigene editing, which greatly promoted the development of genetic manipulations in filamentous fungi.
In addition to the overexpression or deletion of genes, which occur in desired metabolite pathways, regulation of gene expression at the transcription levels is also very important to obtain high yields of metabolites (Mougiakos et al., 2018). Conventional methods to regulate gene expression were to use different promoters with desired strength or RNA interference (Crook et al., 2014). However, with the increased numbers of target genes, the task of testing promoters with suitable strength was time-consuming and labor-intensive. In addition, in order to achieve transcriptional control in terms of level and timing, a complex and sophisticated genetic circuit including activating and repressive transcription factors is indispensable. According to the unique features of the CRISPR/Cas9 system, Jinek et al. constructed a catalytically deactivated Cas9 (dCas9) by introducing inactivating mutations into two nuclease domains of the Cas9 endonuclease, one in the HNH nuclease domain (H840A) and the other in the RuvC-like (D10A) domain (Jinek et al., 2012). This dCas9 protein lost the activity of DNA cleavage but retained the ability to specifically bind to target DNA sequences complementary to the sgRNA. Soon after, this CRISPR/dCas9 system was employed to synthesize a transcriptional repressor. Qi et al. showed that RNA guiding of dCas9 to target genes or promoters would block RNA polymerase binding and genetic transcription, leading to the repression of gene expression (Qi et al., 2013). This CRISPR interference (CRISPRi) system subsequently was used to create efficient, programmable, and genome-wide scale transcriptional regulators (Gilbert et al., 2013, 2014; Silvana et al., 2015). Similarly, CRISPR activation (CRISPRa) was constructed through the fusion of dCas9 to transcriptional activators or activation domains, allowing for transcriptional upregulation of select genes (David et al., 2013). To investigate whether the system could activate the transcription of reporter gene, dCas9 with an omega (ω) protein was fused, which could improve RNA polymerase activity and activate the transcription in E. coli. As a result, the transcription level of the reporter gene was increased by 2.8 times (David et al., 2013). In order to broaden the application of CRISPRa, several transcription activator domains have been excavated, such as VP64 and p65AD (Farzadfard et al., 2013; Silvana et al., 2015). Therefore, CRISPRi/a was allowed for facile transcriptional modification of gene networks and satisfied as an important tool to control enzyme expression levels in endogenous or synthetic pathways (Donohoue et al., 2017).
The core concept of synthetic biology is to transform existing natural systems and construct gene circuits by building and integrating standardized components and modules (Dai et al., 2018). However, an ultimate challenge in the construction of gene circuits was lack of effective, programmable, secure, and sequence-specific gene editing tools. The CRISPRi/a system was poised to solve this problem owning to its programmable targeting, efficacy as activator or repressor, high specificity, and rapid and tight binding kinetics (Sternberg et al., 2014; Xu and Qi, 2019). The CRISPR/Cas9 transcriptional regulator (CRISPR-TF) has been developed to become an important component of scalable device libraries, which were essential in creating complex genetic circuits. Nissim et al. constructed a multi-RNA-mediated gene network regulatory toolkit including RNA-triple-helix structures, introns, microRNAs, ribozymes, Cas9-based CRISPR-TFs, and Cas6/Csy4-based RNA processing to perform tunable synthetic circuits (Nissim et al., 2014). Nielsen et al. also constructed multiple transcriptional logic gates by employing CRISPR/dCas9 and linked them to perform logical computations in living cells (Nielsen and Voigt, 2014). In specific applications, researchers constructed a set of NOT gates by designing five synthetic σ70 promoters in E. coli. These promoters were inhibited by the corresponding sgRNAs, and interrelationships between various components did not exhibit crosstalk. Furthermore, they used these NOT gates to build larger lines, including the Boolean-complete NOR gate and three gates, which were consisted of four-layered sgRNAs. The previously designed gene synthetic lines were ligated into the native regulatory network using an export sgRNA capable of targeting the transcriptional regulator (MalT) in E. coli. By using these methods, the output of synthetic lines can be converted into switches of various cell phenotypes, such as sugar utilization, chemotaxis, and phage resistance.
Comparing with traditional methods of regulating expression, these complex gene circuits based on CRISPRi/a could carry out more precise regulation in terms of levels and timing. In addition, a cascade of responses in these circuits could be triggered by only one signal, which is more advanced than complex promoter engineering. Implementation of CRISPRi/a to design precise gene circuits in biosynthesis pathways will facilitate an unprecedented level of control of metabolic flux and promote the rapid development of the bioindustry.
In the past, non-model microbes have been greatly limited in product diversity and yield due to the lack of efficient genetic engineering tools. These problems could be solved under the wide application of the CRISPR/Cas9 system, which will bring a bright future in the industrial biotechnology. Comparing to the conventional marker-based genome editing tools, the CRISPR/Cas9 system enabled fast strain engineering of prototrophic wild and industrial strains, allowing for multiple genome editing simultaneously with marker cassette integration (Stovicek et al., 2017). The high-throughput screening of industrial strains was another bottleneck in industrial biology (Donohoue et al., 2017). Recently, droplet-based microfluidics technology attracted great attention in terms of strain isolation and characterization due to its high-throughput screening efficiency. The combination of the CRISPR/Cas9 system and droplet-based microfluidics technologies maybe bring new breakthroughs in industrial biology in the future.
Although CRISPR/Cas9-meditated genome editing techniques were increasingly used in biotechnology, some disadvantages still existed (Zhang et al., 2019). First, the off-target effect is still a main issue needed to be solved. The designed sgRNAs may form mismatches with non-target DNA sequences, resulting in unexpected gene mutations. These unexpected gene mutations may cause genomic instability and disrupt the function of other normal genes. Although a variety of methods have optimized off-target effects, further improvement is still needed. Hence, conformational changes associated with sgRNA and DNA recognition need to be further explored to obtain higher genome editing accuracy (Mir et al., 2017). In addition, time-dependent control of Cas9 protein activity and the ratio of Cas9 to sgRNA also require further investigation. High expression of Cas9 protein, whether in gene knockout or gene insertion, will affect the growth and recovery rate of host bacteria after transformation, and cause fatal damage to cells in most cases. The existing methods to increase the HR frequency are not suitable to many non-conventional industrial microorganisms. Hence, exploitation of novel approaches to improve homologous recombination efficiency in these strains is critical for precise and efficient CRISPR/Cas9-mediated genetic engineering. Besides, the intellectual property and scientific ethics issues also hindered the wide application of CRISPR/Cas9. The patent dispute has come to an end in the United States, but the war is continuing in Europe and other countries, delaying its adaptation for industrial biotechnology and pharmaceutical applications. Just like the first test-tube baby that appeared in 1978, employing CRISPR/Cas9 for gene therapy also caused some controversies. Therefore, if the laws in the application of the CRISPR/Cas system were completed, it would promote rapid development in the fields of medicine, genomics, agriculture, and so on.
SZ conceived, designed, and drafted the paper. SZ and FG wrote the part of the application of CRISPR-Cas9 in bacteria. WY and ZD wrote the part of the application of CRISPR-Cas9 system in Yeast. JZ and WD wrote the part of the applications of CRISPR-Cas9 system in filamentous fungi. MJ, WZ, and FX wrote the part of Cas9-mediated transcriptional regulation and critically revised the manuscript. All authors read and approved the final manuscript.
This work was supported by National Key R&D Program of China (2018YFA0902200), National Natural Science Foundation of China (Nos. 21978130, 31961133017, 21706125, 21978129), Jiangsu Province Natural Science Foundation for Youths (BK20170993, BK20170997), the Jiangsu Synergetic Innovation Center for Advanced Bio-Manufacture, Jiangsu Key Lab of Biomass-based Green Fuels and Chemicals Foundation (JSBEM201908), and Project of State Key Laboratory of Materials-Oriented Chemical Engineering (ZK201601).
The authors declare that the research was conducted in the absence of any commercial or financial relationships that could be construed as a potential conflict of interest.
Bao, Z., Xiao, H., Liang, J., Zhang, L., Xiong, X., Sun, N., et al. (2015). Homology-integrated CRISPR-Cas (HI-CRISPR) system for one-step multigene disruption in Saccharomyces cerevisiae. ACS Synth. Biol. 4, 585–594. doi: 10.1021/sb500255k
Bruder, M. R., Pyne, M. E., Moo-Young, M., Chung, D. A., and Chou, C. P. (2016). Extending CRISPR-Cas9 technology from genome editing to transcriptional engineering in Clostridium. Appl. Environ. Microbiol. 82, 6109–6119. doi: 10.1128/AEM.02128-16
Cai, P., Gao, J. Q., and Zhou, Y. J. (2019). CRISPR-mediated genome editing in non-conventional yeasts for biotechnological applications. Microb. Cell Fact. 18:63. doi: 10.1186/s12934-019-1112-2
Capecchi, M. R. (1989). Altering the genome by homologous recombination. Science 244, 1288–1292. doi: 10.1126/science.2660260
Cho, J. S., Choi, K. R., Prabowo, C. P. S., Shin, J. H., Yang, D., Jang, J., et al. (2017). CRISPR/Cas9-coupled recombineering for metabolic engineering of Corynebacterium glutamicum. Metab. Eng. 42, 157–167. doi: 10.1016/j.ymben.2017.06.010
Chuai, G. H., Wang, Q. L., and Liu, Q. (2017). In silico meets in vivo: towards computational CRISPR-based sgRNA design. Trends Biotechnol. 35, 12–21. doi: 10.1016/j.tibtech.2016.06.008
Cong, L., Ran, F. A., Cox, D., Lin, S., Barretto, R., Habib, N., et al. (2013). Multiplex genome engineering using CRISPR/Cas systems. Science 339, 819–823. doi: 10.1126/science.1231143
Crook, N. C., Schmitz, A. C., and Alper, H. S. (2014). Optimization of a yeast RNA interference system for controlling gene expression and enabling rapid metabolic engineering. ACS Synth. Biol. 3, 307–313. doi: 10.1021/sb4001432
Dai, Z., Zhang, S., Yang, Q., Zhang, W., Qian, X., Dong, W., et al. (2018). Genetic tool development and systemic regulation in biosynthetic technology. Biotechnol. Biofuels 11:152. doi: 10.1186/s13068-018-1153-5
David, B., Wenyan, J., Poulami, S., Ann, H., Feng, Z., and Marraffini, L. A. (2013). Programmable repression and activation of bacterial gene expression using an engineered CRISPR-Cas system. Nucleic Acids Res. 41, 7429–7437. doi: 10.1093/nar/gkt520
Dicarlo, J. E., Norville, J. E., Prashant, M., Xavier, R., John, A., and Church, G. M. (2013). Genome engineering in Saccharomyces cerevisiae using CRISPR-Cas systems. Nucleic Acids Res. 41, 4336–4343. doi: 10.1093/nar/gkt135
Donohoue, P. D., Barrangou, R., and May, A. P. (2017). Advances in industrial biotechnology using CRISPR-Cas systems. Trends Biotechnol. 36, 134–146. doi: 10.1016/j.tibtech.2017.07.007
Dufossé, L., Fouillaud, M., Caro, Y., Mapari, S. A., and Sutthiwong, N. (2014). Filamentous fungi are large-scale producers of pigments and colorants for the food industry. Curr. Opin. Biotech. 26, 56–61. doi: 10.1016/j.copbio.2013.09.007
Farzadfard, F., Perli, S. D., and Lu, T. K. (2013). Tunable and multifunctional eukaryotic transcription factors based on CRISPR/Cas. ACS Synth. Biol. 2, 604–613. doi: 10.1021/sb400081r
Feng, X., Zhao, D. D., Zhang, X. L., Ding, X., and Bi, C. H. (2018). CRISPR/Cas9 assisted multiplex genome editing technique in Escherichia coli. Biotechnol. J. 13:e1700604. doi: 10.1002/biot.201700604
Gaj, T., Gersbach, C. A., and Iii, C. F. B. (2013). ZFN, TALEN, and CRISPR/Cas-based methods for genome engineering. Trends Biotechnol. 31, 397–405. doi: 10.1016/j.tibtech.2013.04.004
Gao, S., Tong, Y., Wen, Z., Zhu, L., Ge, M., Chen, D., et al. (2016). Multiplex gene editing of the Yarrowia lipolytica genome using the CRISPR-Cas9 system. J. Ind. Microbiol. Biotechnol. 43, 1085–1093. doi: 10.1007/s10295-016-1789-8
Garst, A. D., Bassalo, M. C., Pines, G., Lynch, S. A., Halwegedwards, A. L., Liu, R., et al. (2017). Genome-wide mapping of mutations at single-nucleotide resolution for protein, metabolic and genome engineering. Nat. Biotechnol. 35, 48–55. doi: 10.1038/nbt.3718
Gilbert, L., Horlbeck, M., Adamson, B., Villalta, J., Chen, Y., Whitehead, E., et al. (2014). Genome-scale CRISPR-mediated control of gene repression and activation. Cell 159, 647–661. doi: 10.1016/j.cell.2014.09.029
Gilbert, L. A., Larson, M. H., Leonardo, M., Zairan, L., Brar, G. A., Torres, S. E., et al. (2013). CRISPR-mediated modular RNA-guided regulation of transcription in eukaryotes. Cell 154, 442–451. doi: 10.1016/j.cell.2013.06.044
Hao, F., and Xia, L. (2015). Cellulase production by recombinant Trichoderma reesei and its application in enzymatic hydrolysis of agricultural residues. Fuel 143, 211–216. doi: 10.1016/j.fuel.2014.11.056
Hao, Z., and Su, X. (2019). Fast gene disruption in Trichoderma reesei using in vitro assembled Cas9/gRNA complex. BMC Biotechnol. 19:2. doi: 10.1186/s12896-018-0498-y
Horvath, P., and Barrangou, R. (2010). CRISPR/Cas, the immune system of bacteria and archaea. Science 327, 167–170. doi: 10.1126/science.1179555
Horwitz, A., Walter, J., Schubert, M., Kung, S., Hawkins, K., Platt, D., et al. (2015). Efficient multiplexed integration of synergistic alleles and metabolic pathways in yeasts via CRISPR-Cas. Cell Syst. 1, 88–96. doi: 10.1016/j.cels.2015.02.001
Hsu, P., Lander, E., and Zhang, F. (2014). Development and applications of CRISPR-Cas9 for genome engineering. Cell 157, 1262–1278. doi: 10.1016/j.cell.2014.05.010
Jacobs, J. Z., Ciccaglione, K. M., Tournier, V., and Zaratiegui, M. (2014). Implementation of the CRISPR-Cas9 system in fission yeast. Nat. Commun. 5:5344. doi: 10.1038/ncomms6344
Jakočiunas, T., Bonde, I., Herrgård, M., Harrison, S. J., Kristensen, M., Pedersen, L. E., et al. (2015a). Multiplex metabolic pathway engineering using CRISPR/Cas9 in Saccharomyces cerevisiae. Metab. Eng. 28, 213–222. doi: 10.1016/j.ymben.2015.01.008
Jakočiunas, T., Jensen, M. K., and Keasling, J. D. (2016). CRISPR/Cas9 advances engineering of microbial cell factories. Metab. Eng. 34, 44–59. doi: 10.1016/j.ymben.2015.12.003
Jakočiunas, T., Rajkumar, A. S., Zhang, J., Arsovska, D., Rodriguez, A., Jendresen, C. B., et al. (2015b). CasEMBLR: Cas9-facilitated multiloci genomic integration of in vivo assembled DNA parts in Saccharomyces cerevisiae. ACS Synth. Biol. 4, 1226–1234. doi: 10.1021/acssynbio.5b00007
Jee-Hwan, O., and Jan-Peter, V. P. (2014). CRISPR-Cas9-assisted recombineering in Lactobacillus reuteri. Nucleic Acids Res. 42:e131. doi: 10.1093/nar/gku623
Ji, X. J., Ren, L. J., Nie, Z. K., Huang, H., and Ouyang, P. K. (2014). Fungal arachidonic acid-rich oil: research, development and industrialization. Crit. Rew. Biotechnol. 34, 197–214. doi: 10.3109/07388551.2013.778229
Jiang, F., and Doudna, J. A. (2017). CRISPR–Cas9 structures and mechanisms. Annu. Rev. Biophys. 46, 505–529. doi: 10.1146/annurev-biophys-062215-010822
Jiang, W., Bikard, D., Cox, D., Zhang, F., and Marraffini, L. A. (2013). RNA-guided editing of bacterial genomes using CRISPR-Cas systems. Nat. Biotechnol. 31, 233–239. doi: 10.1038/nbt.2508
Jinek, M., Chylinski, K., Fonfara, I., Hauer, M., Doudna, J. A., and Charpentier, E. (2012). A programmable dual-RNA–guided DNA endonuclease in adaptive bacterial immunity. Science 337, 816–821. doi: 10.1126/science.1225829
Li, H., Shen, C. R., Huang, C. H., Sung, L. Y., Wu, M. Y., and Hu, Y. C. (2016). CRISPR-Cas9 for the genome engineering of Cyanobacteria and succinate production. Metab. Eng. 38, 293–302. doi: 10.1016/j.ymben.2016.09.006
Li, Y., Lin, Z., Huang, C., Zhang, Y., Wang, Z., Tang, Y. J., et al. (2015). Metabolic engineering of Escherichia coli using CRISPR–Cas9 meditated genome editing. Metab. Eng. 31, 13–21. doi: 10.1016/j.ymben.2015.06.006
Lian, J., Hamedirad, M., and Zhao, H. (2018). Advancing metabolic engineering of Saccharomyces cerevisiae using the CRISPR/Cas system. Biotechnol. J. 13:e1700601. doi: 10.1002/biot.201700601
Liang, L., Liu, R., Garst, A. D., Lee, T., Nogué, V. S. I., Beckham, G. T., et al. (2017). CRISPR enabled trackable genome engineering for isopropanol production in Escherichia coli. Metab. Eng. 41, 1–10. doi: 10.1016/j.ymben.2017.02.009
Liu, J., Wang, Y., Lu, Y., Zheng, P., Sun, J., and Ma, Y. (2017). Development of a CRISPR/Cas9 genome editing toolbox for Corynebacterium glutamicum. Microb. Cell Fact. 16:205. doi: 10.1186/s12934-017-0815-5
Liu, R., Chen, L., Jiang, Y., Zhou, Z., and Zou, G. (2015). Efficient genome editing in filamentous fungus Trichoderma reesei using the CRISPR/Cas9 system. Cell Discov. 1:15007. doi: 10.1038/celldisc.2015.7
Löbs, A. K., Engel, R., Schwartz, C., Flores, A., and Wheeldon, I. (2017). CRISPR–Cas9-enabled genetic disruptions for understanding ethanol and ethyl acetate biosynthesis in Kluyveromyces marxianus. Biotechnol. Biofuels 10:164. doi: 10.1186/s13068-017-0854-5
Mali, P., Yang, L., Esvelt, K. M., Aach, J., Guell, M., DiCarlo, J. E., et al. (2013). RNA-guided human genome engineering via Cas9. Science 339, 823–826. doi: 10.1126/science.1232033
Mir, A., Edraki, A., Lee, J., and Sontheimer, E. J. (2017). Type II-C CRISPR-Cas9 biology, mechanism, and application. ACS Chem. Biol. 13, 357–365. doi: 10.1021/acschembio.7b00855
Mitsui, R., Yamada, R., and Ogino, H. (2019). CRISPR system in the yeast Saccharomyces cerevisiae and its application in the bioproduction of useful chemicals. World J. Microb. Biotechnol. 35:111. doi: 10.1007/s11274-019-2688-8
Mougiakos, I., Bosma, E. F., Ganguly, J., Van, D. O. J., and Van, K. R. (2018). Hijacking CRISPR-Cas for high-throughput bacterial metabolic engineering: advances and prospects. Curr. Opin. Biotech. 50, 146–157. doi: 10.1016/j.copbio.2018.01.002
Murphy, K. C., and Campellone, K. G. (2003). Lambda Red-mediated recombinogenic engineering of enterohemorrhagic and enteropathogenic E. coli. BMC Mol. Biol. 4:11. doi: 10.1186/1471-2199-4-11
Nagaraju, S., Davies, N. K., Walker, D. J. F., Köpke, M., and Simpson, S. D. (2016). Genome editing of Clostridium autoethanogenum using CRISPR/Cas9. Biotechnol. Biofuels 9:219. doi: 10.1186/s13068-016-0638-3
Nielsen, A. A., and Voigt, C. A. (2014). Multi-input CRISPR/Cas genetic circuits that interface host regulatory networks. Mol. Syst. Biol. 10, 763–763. doi: 10.15252/msb.20145735
Nissim, L., Perli, S., Fridkin, A., Perez-Pinera, P., and Lu, T. (2014). Multiplexed and programmable regulation of gene networks with an integrated RNA and CRISPR/Cas toolkit in human cells. Mol. Cell 54, 698–710. doi: 10.1016/j.molcel.2014.04.022
Nødvig, C. S., Nielsen, J. B., Kogle, M. E., and Mortensen, U. H. (2015). A CRISPR-Cas9 system for genetic engineering of filamentous fungi. PLoS ONE 10:e0133085. doi: 10.1371/journal.pone.0133085
Numamoto, M., Maekawa, H., and Kaneko, Y. (2017). Efficient genome editing by CRISPR/Cas9 with a tRNA-sgRNA fusion in the methylotrophic yeast Ogataea polymorpha. J. Biosci. Bioeng. 124, 487–492. doi: 10.1016/j.jbiosc.2017.06.001
Papoutsakis, E. T. (2008). Engineering solventogenic clostridia. Curr. Opin. Biotechnol. 19, 420–429. doi: 10.1016/j.copbio.2008.08.003
Park, J., Yu, B. J., Choi, J. I., and Woo, H. M. (2019). Heterologous production of squalene from glucose in engineered Corynebacterium glutamicum using multiplex CRISPR interference and high-throughput fermentation. J Agric Food Chem 67, 308–319. doi: 10.1021/acs.jafc.8b05818
Pyne, M. E., Bruder, M., Moo-Young, M., Chung, D. A., and Chou, C. P. (2014). Technical guide for genetic advancement of underdeveloped and intractable Clostridium. Biotechnol. Adv. 32, 623–641. doi: 10.1016/j.biotechadv.2014.04.003
Pyne, M. E., Bruder, M. R., Mooyoung, M., Chung, D. A., and Chou, C. P. (2016). Harnessing heterologous and endogenous CRISPR-Cas machineries for efficient markerless genome editing in Clostridium. Sci. Rep. 6:25666. doi: 10.1038/srep25666
Qi, L. S., Larson, M. H., Gilbert, L. A., Doudna, J. A., Weissman, J. S., Arkin, A. P., et al. (2013). Repurposing CRISPR as an RNA-guided platform for sequence-specific control of gene expression. Cell 152, 1173–1183. doi: 10.1016/j.cell.2013.02.022
Raschmanova, H., Weninger, A., Glieder, A., Kovar, K., and Vogl, T. (2018). Implementing CRISPR-Cas technologies in conventional and non-conventional yeasts: current state and future prospects. Biotechnol. Adv. 36, 641–665. doi: 10.1016/j.biotechadv.2018.01.006
Ronda, C., Maury, J., JakočiuNas, T., Jacobsen, S. A. B., Germann, S. M., Harrison, S. J., et al. (2015). CrEdit: CRISPR mediated multi-loci gene integration in Saccharomyces cerevisiae. Microb. Cell Fact. 14:97. doi: 10.1186/s12934-015-0288-3
Ryan, O. W., Skerker, J. M., Maurer, M. J., Li, X., Tsai, J. C., Poddar, S., et al. (2014). Selection of chromosomal DNA libraries using a multiplex CRISPR system. Elife Sci. 3:e03703. doi: 10.7554/eLife.03703
Schwartz, C. M., Hussain, M. S., Blenner, M., and Wheeldon, I. (2015). Synthetic RNA polymerase III promoters facilitate high-efficiency CRISPR–Cas9-mediated genome editing in Yarrowia lipolytica. ACS Synth. Biol. 5, 356–9. doi: 10.1021/acssynbio.5b00162
Silvana, K., Brigham, M. D., Trevino, A. E., Julia, J., Abudayyeh, O. O., Clea, B., et al. (2015). Genome-scale transcriptional activation by an engineered CRISPR-Cas9 complex. Nature 517, 583–588. doi: 10.1038/nature14136
So, Y., Park, S. Y., Park, E. H., Park, S. H., Kim, E. J., Pan, J. G., et al. (2017). A highly efficient CRISPR-Cas9-mediated large genomic deletion in Bacillus subtilis. Front. Microbiol. 8:1167. doi: 10.3389/fmicb.2017.01167
Sternberg, S. H., Sy, R., Martin, J., Greene, E. C., and Doudna, J. A. (2014). DNA interrogation by the CRISPR RNA-guided endonuclease Cas9. Nature 507, 62–67. doi: 10.1038/nature13011
Stovicek, V., Holkenbrink, C., and Borodina, I. (2017). CRISPR/Cas system for yeast genome engineering: advances and applications. FEMS Yeast Res. 17:fox030. doi: 10.1093/femsyr/fox030
Thomas, V., Hartner, F. S., and Anton, G. (2013). New opportunities by synthetic biology for biopharmaceutical production in Pichia pastoris. Curr. Opin. Biotech. 24, 1094–1101. doi: 10.1016/j.copbio.2013.02.024
Tsai, C. S., Kong, I. I., Lesmana, A., Million, G., Zhang, G. C., Kim, S. R., et al. (2015). Rapid and marker-free Cas9/CRISPR refactoring yields equivalent xylose-utilization performance in yeast. Biotechnol. Bioeng. 112, 2406–2411. doi: 10.1002/bit.25632
Walter, J. M., Chandran, S. S., and Horwitz, A. A. (2016). CRISPR-Cas-Assisted Multiplexing (CAM): simple same-day multi-locus engineering in yeast. J. Cell. Physiol. 231, 2563–2569. doi: 10.1002/jcp.25375
Wang, L., Deng, A., Zhang, Y., Liu, S., Liang, Y., Bai, H., et al. (2018). Efficient CRISPR–Cas9 mediated multiplex genome editing in yeasts. Biotechnol. Biofuels 11:277. doi: 10.1186/s13068-018-1271-0
Wang, S., Dong, S., Wang, P., Tao, Y., and Wang, Y. (2017). Genome Editing in Clostridium saccharoperbutylacetonicum N1-4 with the CRISPR-Cas9 system. Appl. Environ. Microbiol. 83:e00233-17. doi: 10.1128/AEM.00233-17
Wang, Y., Cobb, R. E., and Zhao, H. (2016). High-efficiency genome editing of Streptomyces species by an engineered CRISPR/Cas system. Method Enzymol. 575, 271–284. doi: 10.1016/bs.mie.2016.03.014
Wasels, F., Jean-Marie, J., Collas, F., López-Contreras, A. M., and Ferreira, N. L. (2017). A two-plasmid inducible CRISPR/Cas9 genome editing tool for Clostridium acetobutylicum. J. Microbiol. Methods 140, 5–11. doi: 10.1016/j.mimet.2017.06.010
Weber, J., Valiante, V., Nødvig, C. S., Mattern, D. J., Slotkowski, R. A., Mortensen, U. H., et al. (2016). Functional reconstitution of a fungal natural product gene cluster by advanced genome editing. ACS Synth. Biol. 6, 62–68. doi: 10.1021/acssynbio.6b00203
Weld, R. J., Plummer, K. M., Carpenter, M. A., and Ridgway, H. J. (2006). Approaches to functional genomics in filamentous fungi. Cell Res. 16, 31–44. doi: 10.1038/sj.cr.7310006
Weninger, A., Fischer, J., Raschmanová, H., Kniely, C., Vogl, T., and Glieder, A. (2018). Expanding the CRISPR/Cas9 toolkit for Pichia pastoris with efficient donor integration and alternative resistance markers. J. Cell. Biochem. 119, 3183–3198. doi: 10.1002/jcb.26474
Weninger, A., Hatzl, A. M., Schmid, C., Vogl, T., and Glieder, A. (2016). Combinatorial optimization of CRISPR/Cas9 expression enables precision genome engineering in the methylotrophic yeast Pichia pastoris. J. Biotechnol. 235, 139–149. doi: 10.1016/j.jbiotec.2016.03.027
Westbrook, A. W., Moo-Young, M., and Chou, C. P. (2016). Development of a CRISPR-Cas9 tool kit for comprehensive engineering of Bacillus subtilis. Appl. Environ. Microbiol. 82, 4876–4895. doi: 10.1128/AEM.01159-16
Weyda, I., Yang, L., Vang, J., Ahring, B. K., Lübeck, M., and Lübeck, P. S. (2017). A comparison of Agrobacterium-mediated transformation and protoplast-mediated transformation with CRISPR-Cas9 and bipartite gene targeting substrates, as effective gene targeting tools for Aspergillus carbonarius. J. Microbiol. Methods 135, 26–34. doi: 10.1016/j.mimet.2017.01.015
Xu, T., Li, Y., Shi, Z., Hemme, C. L., Li, Y., Zhu, Y., et al. (2015). Efficient genome editing in Clostridium cellulolyticum via CRISPR-Cas9 nickase. Appl. Environ. Microb. 81, 4423–4431. doi: 10.1128/AEM.00873-15
Xu, X., and Qi, L. S. (2019). A CRISPR-dCas toolbox for genetic engineering and synthetic biology. J. Mol. Biol. 431, 34–47. doi: 10.1016/j.jmb.2018.06.037
Xu, X., Zhang, X., Wu, Z., and Wang, Z. (2015). Accumulation of yellow Monascus pigments by extractive fermentation in nonionic surfactant micelle aqueous solution. Appl. Microbiol. Biotechnol. 99, 1173–1180. doi: 10.1007/s00253-014-6227-0
Yao, R., Liu, D., Jia, X., Zheng, Y., Liu, W., and Xiao, Y. (2018). CRISPR-Cas9/Cas12a biotechnology and application in bacteria. Synth. Syst. Biotechnol. 3, 135–149. doi: 10.1016/j.synbio.2018.09.004
Zhang, C., Meng, X., Wei, X., and Lu, L. (2016). Highly efficient CRISPR mutagenesis by microhomology-mediated end joining in Aspergillus fumigatus. Fungal Genet. Biol. 86, 47–57. doi: 10.1016/j.fgb.2015.12.007
Zhang, G. C., Kong, I. I., Kim, H., Liu, J. J., Cate, J. H., and Jin, Y. S. (2014). Construction of a quadruple auxotrophic mutant of an industrial polyploid Saccharomyces cerevisiae strain by using RNA-guided Cas9 nuclease. Appl. Environ. Microbiol. 80, 7694–7701. doi: 10.1128/AEM.02310-14
Keywords: industrial biology, CRISPR/Cas9, synthetic biology, gene circuits, genetic engineering
Citation: Zhang S, Guo F, Yan W, Dai Z, Dong W, Zhou J, Zhang W, Xin F and Jiang M (2020) Recent Advances of CRISPR/Cas9-Based Genetic Engineering and Transcriptional Regulation in Industrial Biology. Front. Bioeng. Biotechnol. 7:459. doi: 10.3389/fbioe.2019.00459
Received: 02 September 2019; Accepted: 19 December 2019;
Published: 28 January 2020.
Edited by:
Pablo Ivan Nikel, Novo Nordisk Foundation Center for Biosustainability (DTU Biosustain), DenmarkReviewed by:
Hodaka Fujii, Hirosaki University, JapanCopyright © 2020 Zhang, Guo, Yan, Dai, Dong, Zhou, Zhang, Xin and Jiang. This is an open-access article distributed under the terms of the Creative Commons Attribution License (CC BY). The use, distribution or reproduction in other forums is permitted, provided the original author(s) and the copyright owner(s) are credited and that the original publication in this journal is cited, in accordance with accepted academic practice. No use, distribution or reproduction is permitted which does not comply with these terms.
*Correspondence: Wenming Zhang, emhhbmd3bUBuanRlY2guZWR1LmNu; Fengxue Xin, eGluZmVuZ3h1ZUBuanRlY2guZWR1LmNu; Min Jiang, amlhbmdtaW5Abmp0ZWNoLmVkdS5jbg==
Disclaimer: All claims expressed in this article are solely those of the authors and do not necessarily represent those of their affiliated organizations, or those of the publisher, the editors and the reviewers. Any product that may be evaluated in this article or claim that may be made by its manufacturer is not guaranteed or endorsed by the publisher.
Research integrity at Frontiers
Learn more about the work of our research integrity team to safeguard the quality of each article we publish.