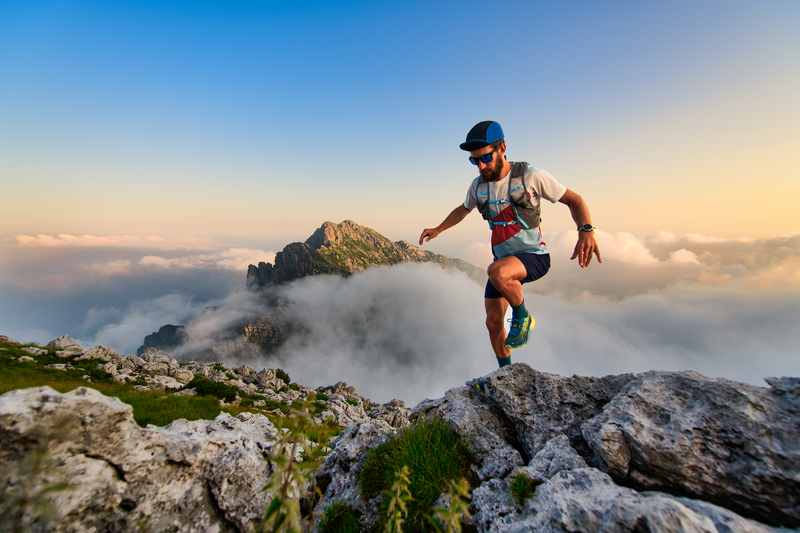
94% of researchers rate our articles as excellent or good
Learn more about the work of our research integrity team to safeguard the quality of each article we publish.
Find out more
PERSPECTIVE article
Front. Bioeng. Biotechnol. , 10 October 2019
Sec. Biomaterials
Volume 7 - 2019 | https://doi.org/10.3389/fbioe.2019.00260
This article is part of the Research Topic The Application of Biomaterials to Advance Induced Pluripotent Stem Cells in Tissue Engineering View all 8 articles
A primary goal in tissue engineering is to develop functional tissues by recapitulating salient features of complex biological systems that exhibit a diverse range of physical forces. Induced pluripotent stem cells (iPSCs) are promising autologous cell sources to execute these developmental programs and their functions; however, cells require an extracellular environment where they will sense and respond to mechanical forces. Thus, understanding the biophysical relationships between stem cells and their extracellular environments will improve the ability to design complex biological systems through tissue engineering. This article first describes how the mechanical properties of the environment are important determinants of developmental processes, and then further details how biomaterials can be designed to precisely control the mechanics of cell-matrix interactions in order to study and define their reprogramming, self-renewal, differentiation, and morphogenesis. Finally, a perspective is presented on how insights from the mechanics of cell-matrix interactions can be leveraged to control pluripotent stem cells for tissue engineering applications.
Complex biological structures arise from developmental processes, such as morphogenesis, which involves the precise transformation of materials with diverse physical properties. These materials consist of extracellular matrix (ECM), interstitial fluids (IFs) within the ECM, and cells (Forgacs and Newman, 2005). ECMs consist of fibrous components such as collagens and elastic fibers as well as glycoproteins and proteoglycans. The IF consists mainly of water and exists throughout the ECM, where it holds and transports biochemical factors critical for tissue homeostasis. The ECM and the IF jointly exhibit a diverse range of mechanical properties throughout biological systems, and the processes occurring within these systems are intrinsically tied to these properties. Embryonic development occurs within a matrix (Rozario and Desimone, 2010), and the embryo must sustain and express a diverse range of forces in order to develop into a precise and complex form. In this way, cell differentiation is fundamentally tied to force recognition and force generation (Davidson, 2017). Evidence exists for an epigenetic feedback system that allows switching of gene expression within cells depending on their morphogenic requirements (Table 1)—a system likely to be influenced by the physical environment (Smith S. J. et al., 2018). A specific example is observed in frog embryos, where mechanical properties of tissue and their relationship to the surrounding environment depend precisely on developmental stage and localization within the embryo (Shawky et al., 2018). Given these distinctions and the fact that cells exist within a diverse biophysical environment, it becomes clear that physical relationships between cell and environment are profoundly important in directing cell behaviors, including cell lineage specifications. In studies primarily involving adult stem cells, such considerations have been implemented (Shin and Mooney, 2016; Hiew et al., 2018). However, embryonic stem cells (ESCs) and morphogenesis remain to be understood with a deeper understanding of how cells generate forces and respond to mechanics of the ECM. Biomaterials can be designed to model the ECM by recapitulating biophysical properties—including intrinsic and extrinsic mechanical properties (Figure 1). Intrinsic properties such as stiffness, viscoelasticity, and degradability are based on the molecular properties of the ECM and are independent of scale (Reilly and Engler, 2010; Lee et al., 2016). Extrinsic properties such as dimensionality, patterning, and morphology are highly specific to scale and indeed very important when considering complex structures required in tissue engineering (Lee et al., 2016).
Table 1. Selected genes known to be involved in epigenetic feedback related to cell morphogenic requirements.
Figure 1. Engineered biomaterials can be used to model diverse mechanical properties of ECM. The native ECM is composed of fibrous materials (collagen, elastic fibers) as well as glycoproteins and proteoglycans, which confer adhesion between cells and the ECM. The ECM and the IF that exists within can possess many distinct mechanical properties, each of which can be recapitulated individually or in combination using biomaterial design. Intrinsic properties include stiffness, viscoelasticity, and degradability, and are generally independent of scale. Extrinsic properties include dimensionality, patterning, and morphology/geometry, and are determined by scale.
Biomaterial approaches to precisely control intrinsic and extrinsic biophysical properties of the environment will not only enable basic investigations into how matrix mechanics regulate ESCs and their morphogenesis but will also enable engineering applications leveraged to direct ESCs and induced pluripotent stem cells (iPSCs) toward various cell fates and tissue types. This article aims to describe the relationship between biomaterial mechanics and stem cell functions while summarizing existing strategies for biomaterials to direct stem cell self-renewal, reprogramming, and differentiation. Then, the article presents a perspective on the future of biomaterial systems to implement biomaterial mechanics in iPSC applications for tissue engineering.
Pluripotent stem cells (PSCs) are unique in their potential to undergo self-renewal and differentiation into many distinct types of cells. While ESCs were previously the major source of PSCs (Hou et al., 2013), acquisition of human ESCs from donors presents both logistic and ethical issues. Since the first demonstration of conversion from adult somatic cells into PSCs (induced PSCs, or iPSCs) (Takahashi et al., 2007), intense efforts have been made to leverage this readily accessible stem cell source to understand human development, model human disease, and regenerate human tissues. Numerous studies show that some soluble mediators, such as an inhibitors of transforming growth factor β (TGF-β) signaling, and animal-derived ECMs, such as Matrigel and collagen can be used to facilitate reprogramming of somatic cells into iPSCs (Ichida et al., 2009; Hou et al., 2013; Kim et al., 2013; Caiazzo et al., 2016), maintain self-renewal of ESCs (Watanabe et al., 2007), direct ESC differentiation into lineages (Buttery et al., 2001; Nava et al., 2012), and model organoid formation (Eiraku et al., 2011; Gjorevski et al., 2016). However, spatiotemporal presentation of soluble factors by controlled diffusion through tissues is often important to direct morphogenesis (Kinney and Mcdevitt, 2013; Lienemann et al., 2014; Zhang et al., 2018) and is typically not offered by traditional culture methods. Due to innate heterogeneities, animal-derived ECMs confound clinical translation and their properties are often difficult to control. We contend that designing biomaterials to physically control stem cell-matrix interactions would offer diverse platforms to direct pluripotent stem cells in terms of reprogramming, self-renewal, differentiation, and morphogenesis.
For successful reprogramming into iPSCs, transcription factors need to overcome the epigenetic barriers established by chromatin regulators in somatic cells (Liang and Zhang, 2013). While it is possible that incomplete or abnormal epigenetic reprogramming may not pose a problem for some applications of iPSCs (Maherali et al., 2007), iPSC-derived cells often present different phenotypes than ESC-derived cells. For example, iPSC-derived cardiomyocytes exhibit a slower beat frequency than ESC-derived cardiomyocytes (Zhang et al., 2009). Emerging studies show that the mechanics of culture environments will likely play important roles in epigenetic states of stem cells. Insights from stem cell mechanobiology already predict this observation, since the extent of nuclear lamin-A polymerization scales with matrix stiffness (Swift et al., 2013), and lamins are key determinants of how different chromatin domains interact with one another through epigenetic changes (Guelen et al., 2008; Zheng et al., 2018). A pioneering study shows that elongating fibroblasts on microgroove substrates increases iPSC reprogramming efficiency by activating chromatin regulators, such as histone H3 methyltransferase, which is necessary to overcome epigenetic barriers (Downing et al., 2013). A study with tumor-initiating stem-like cells shows that a soft fibrin-based matrix promotes histone H3 lysine 9 (H3K9) demethylation and Sex Determining Region Y-Box 2 (Sox2) expression (Tan et al., 2014), both of which are known determinants of iPSC reprogramming (Jaenisch and Young, 2008; Chen et al., 2013). Changes in epigenetic states induced by culture environments may be irreversible, as implied by a study showing that mesenchymal stromal cells (MSCs) cultured on rigid substrates for an extended period of time are no longer able to sense the difference between soft and stiff substrates (Yang et al., 2014). Thus, these studies suggest that biomaterial design can be used as an early intervention strategy to improve reprogramming by lifting epigenetic barriers.
Without self-renewal, the stem cell pool in a given system becomes depleted, which is exemplified clinically by conditions such as hematopoietic failure (Ficara et al., 2008; Wilson et al., 2009). Self-renewal of stem cells can be maintained at the level of both cell populations and single cells. At the population level, it is necessary to ensure that a fraction of stem cells is maintained, while the remaining fraction is used for differentiation or morphogenesis. In the absence of differentiation factors, ESCs proliferate rapidly in culture, as they have a significantly shorter G1 phase than other cell types (Becker et al., 2006; Fluckiger et al., 2006). However, the pool of ESCs often becomes depleted as they differentiate (Ruijtenberg and Van Den Heuvel, 2016). To overcome this issue endogenously, small fractions of stem cells are maintained in quiescence (G0 phase) in vivo (Cheung and Rando, 2013). Genetic studies have identified a number of factors from the bone marrow microenvironment that are required for a specific subpopulation of hematopoietic stem cells (HSCs) to undergo quiescence, such as angiopoietin-1 and stem cell factor and thrombopoietin (Arai et al., 2004; Yoshihara et al., 2007; Ding et al., 2012). Some of these factors have been conjugated with biomaterials to maintain stem cells in vitro (Mahadik et al., 2015). Indeed, some factors have been identified to maintain ESC self-renewal, such as basic fibroblast growth factor and leukemia inhibitory factor (Levenstein et al., 2006; Nicola and Babon, 2015). Thus, conjugating specific niche signals with biomaterials to control their spatiotemporal presentation will be useful to maintain self-renewal of a pluripotent stem cell subpopulation while simultaneously directing differentiation of other subpopulations. This strategy also presents opportunities to couple ligand presentation with biomaterial mechanics as demonstrated (Lee et al., 2011; Banks et al., 2014; Kowalczewski and Saul, 2018; Spicer et al., 2018). Alternatively, it is possible to load biochemical factors in materials that exhibit a controlled release property by designing hydrogels (Li and Mooney, 2016) to specifically couple with external stimuli such as temperature, light, affinity, or mechanical signals (Wang et al., 2017) that modulate the controlled release of biochemical factors. For example, heparin-binding-affinity-based delivery systems can be incorporated within hydrogels for simultaneously controlled delivery of several different growth factors to drive differentiation of ESCs into neural progenitors (Willerth et al., 2008). Heparin-affinity and similar systems can also be used to sequester growth factors secreted from cells (Hettiaratchi et al., 2016); for example, sequestration of growth factors secreted from co-cultured osteoblasts within heparin-containing hydrogels drives osteogenic differentiation of encapsulated MSCs (Seto et al., 2012).
At the single-cell level, self-renewal and differentiation can occur simultaneously in asymmetric cell division. During cell division, cues received through niche contact, mitotic spindle polarization, and asymmetric segregation of fate-determining molecules induce a different cell fate in a single daughter cell, while the second daughter cell remains in an undifferentiated state (Knoblich, 2008). Studies with HSCs show that asymmetric division of stem cells involves several different forces. Under external forces such as shear flow or adhesion to rigid matrices, biophysical forces become polarized toward one daughter cell, leading to asymmetric segregation of contractility molecules, such as myosin-IIB (Shin et al., 2014) and cell division cycle 42 (cdc42) (Florian et al., 2012); the daughter cell that retains these molecules remains undifferentiated. Force polarization has since been reported to control ESC self-renewal and fate specification (Maître et al., 2016) and has been used to form organized germ layers from ESCs using a soft fibrin-based matrix (Poh et al., 2014). Thus, biomaterials that control polarization of biophysical forces in dividing stem cells will be useful to maintain self-renewal while directing pluripotent stem cell differentiation.
Tissues exhibit a variety of physical properties. For example, bones and other tissues of mesodermal origin tend to be more rigid, while those of the neuroectoderm origin are soft. Advances in biomaterial design to precisely control material mechanics have revealed fundamental insights behind how stem cells generate forces and sense biophysical properties of the ECM during differentiation. MSCs have been used as a prototypical cell type to understand the mechanics of cell-material interactions, because they elaborate diverse cytoskeletal and nucleoskeletal machinery to sense and respond to the ECM (Discher et al., 2005). Pioneering studies leveraged engineered 2D substrates, such as polydimethylsiloxane (PDMS) and polyacrylamide-based systems, to show the importance of both cell spreading area (Mcbeath et al., 2004) and matrix stiffness (Engler et al., 2006) in directing MSC differentiation. More recent studies show that tuning substrate geometry (Kilian et al., 2010), substrate topography (Abagnale et al., 2015), and viscoelasticity (Cameron et al., 2011) of 2D substrates impacts MSC differentiation. The common conclusion of these studies is that when MSCs exhibit increased spreading and higher cortical tension on a given matrix, they undergo osteogenic differentiation. In contrast, when MSCs spread less and generate less cortical tension, they undergo adipogenic differentiation. Mechanistic studies show that increased cellular tension on stiff matrix increases polymerization of lamin-A, which leads to increased nuclear rigidity and retention of mechanosensitive transcription factors, such as Yes-associated protein (YAP), within the nucleus (Dupont et al., 2011). Nuclear retention of these transcription factors drives subsequent downstream gene expression related to osteogenesis (Swift et al., 2013). These studies suggest profound importance of 2D substrate mechanics in directing stem cell fate.
In contrast to 2D substrates, cells are often mechanically confined in 3D matrix. Therefore, the ability of stem cells to remodel and navigate through confined environments becomes important in understanding and subsequently directing stem cell functions using 3D materials. An earlier study shows that MSCs encapsulated in 3D nanoporous alginate gels that present an integrin ligand (e.g., Arg-Gly-Asp, “RGD”) undergo osteogenesis at an optimal stiffness (Huebsch et al., 2010). While this study suggests the importance of 3D matrix stiffness in directing MSC differentiation, subsequent studies show that the effect is further enhanced when MSCs are allowed to spread and generate more tension, by engineering either a metalloproteinase enzyme-degradable matrix (Khetan et al., 2013) or a matrix with faster relaxation under stress (Chaudhuri et al., 2016). Mechanotransduction pathways remain to be further defined for 3D matrix mechanics, but a recent study highlights the importance of mechanosensitive ion channels in mediating MSC differentiation in stress-relaxing materials (Lee et al., 2019). Thus, mechanical confinement and intrinsic properties of 3D materials can be tuned to control stem cell fate.
Embryonic cells secrete ECM at the earliest stages of development (Rozario and Desimone, 2010), and hence will likely be sensitive to matrix mechanics. The perinuclear region surrounding the nucleus of stem cells exhibits mechanical properties linked to nuclear structure and geometry, which is conserved across multipotent and pluripotent cells and is influenced by biochemical and biophysical inputs (Lozoya et al., 2016). Nucleus size and geometry are linked to expression of nuclear lamins A/C and emerin, which regulate gene expression during differentiation, suggesting that external mechanics are used to guide differentiation of the early embryo (Smith et al., 2017). Along these lines, PSCs exhibit high nuclear deformability that is associated with cells in specific stages of embryonic development (Grespan et al., 2018). For example, cells differentiating toward early ectoderm lineages maintain a more rigid nuclear shape while cells differentiating toward an early endoderm lineage have more deformable nuclei. Recent studies have begun to leverage biomaterials to reveal fundamental insights behind how intrinsic and extrinsic material properties direct differentiation of pluripotent stem cells. ESCs generate a higher traction force on a more rigid PDMS-based micropost array (Sun et al., 2012). While matrix stiffness does not impact self-renewal of ESCs, soft matrices facilitate mesoderm induction by stabilizing cell-cell adhesion through epithelial cadherin (E-cadherin) (Przybyla et al., 2016). A recent study used micropatterning with varied substrate geometry and size to show that iPSCs exhibit higher tension and undergo more mesodermal differentiation into vascular endothelial cadherin (VE-cadherin)+ endothelial cells when they are present in higher local densities (Smith Q. et al., 2018). Another study shows that there exists an optimal stiffness of a polyethylene glycol (PEG)-based hydrogel where sprouting of iPSC-derived endothelial cells becomes the maximum (Zanotelli et al., 2016).
These studies are consistent with the notion that tension generated during early gastrulation events could be an important determinant of mesoderm layer formation (Hammerschmidt and Wedlich, 2008). Thus, biomaterials can be designed to provide pluripotent stem cells with essential mechanical cues to direct their differentiation.
Formation of highly organized tissues depends not only on spatiotemporal presentation of morphogens, substances such as TGF-β and bone morphogenic protein (BMP) that guide the patterned development of tissues, but also on precise control of physical forces. Remarkably, studies in the past decade show that some stem cells can spontaneously develop into organoids when they are cultured in animal-derived matrices such as Matrigel and collagen while in the presence of a specific combination of growth factors (Clevers, 2016). In particular, culturing ESC aggregates in Matrigel leads to an optic cup-like structure where a regional variation in tissue rigidity drives invagination (Eiraku et al., 2011), and external application of a local mechanical strain facilitates this process (Okuda et al., 2018). While these studies suggest that some cells may have the autonomous ability to coordinate physical forces during morphogenesis, cells will likely need to remodel and respond to the ECM to achieve this goal. For instance, both collagen and contractile proteins are upregulated during heart development to achieve tissue stiffness for optimal beating (Majkut et al., 2013). In addition, the ECM in the basement membrane exhibits a stiffness gradient to drive tissue elongation of the follicle during oogenesis (Crest et al., 2017). Indeed, a recent study used a PEG-based hydrogel crosslinked by a thiol-Michael addition to show that the hydrogel needs to be stiff to promote initial proliferation of intestinal stem cells, but needs to become degraded later to promote organoid formation by alleviating compressive forces (Gjorevski et al., 2016). Thus, tailoring biomaterial mechanics to a specific development process will likely determine the success of recapitulating morphogenesis from pluripotent stem cells.
Mechanical properties of the nucleus are closely linked to chromatin states (Wang P. et al., 2018; Zheng et al., 2018) as well as cellular functions such as cell trafficking (Rowat et al., 2013; Shin et al., 2013; Harada et al., 2014) and stem cell differentiation (Shin et al., 2013; Grigoryan et al., 2018). Thus, biomaterial strategies to control nuclear mechanics will significantly impact the success of iPSC reprogramming and downstream functions. The nucleus in pluripotent stem cells is generally more pliable than in differentiated cells (Pajerowski et al., 2007). When cells with more rigid nuclei are rendered deformable by knocking down lamin-A expression, they tend to be susceptible to nuclear rupture and DNA damage induced by mechanical stress, such as squeezing (Harada et al., 2014; Irianto et al., 2017), although cells appear to possess the natural machinery to repair the ruptured nuclei during this process (Denais et al., 2016; Raab et al., 2016). Nuclear deformability is associated with upregulation of H3K4 methylation by WD repeat domain 5 (WDR5) (Downing et al., 2013; Wang P. et al., 2018), which is involved in iPSC reprogramming (Ang et al., 2011). This suggests that biomaterials that enable gradual induction of nuclear deformability without sudden mechanical stress—such as soft, stress-relaxing, or degradable substrates—can potentially enhance reprogramming efficiency while maintaining cell viability.
Since optimal mechanical properties of biomaterials will depend on different cell types and how cells are eventually organized in different tissues, solutions beyond simple bulk cell-matrix interactions will likely be required to leverage biomaterial mechanics for tissue engineering with iPSCs. From a manufacturing perspective, developing methods to precisely control biomaterial mechanics in a spatiotemporal manner using a consistent base material will lead to an economical and elegant solution to fabricate autologous tissues consisting of multiple cell types from a single iPSC source. One general strategy to achieve this goal is to combine biomaterial design with instrumentation, such as by using printers. An important advantage of this strategy is that extrinsic and intrinsic material properties can be independently controlled. In particular, light-crosslinkable materials, such as hydrogels with a methacrylate group, have been used to spatially control hydrogel crosslinking at the microscale and pattern different ligands or cells within the same substrate (Chan et al., 2010; Deforest and Tirrell, 2015; Zhang et al., 2015; Ouyang et al., 2017; Rizwan et al., 2017; Wang Z. et al., 2018). A recent example uses a printing method to spatially tune mechanical properties to simultaneously achieve adipogenic and osteogenic differentiation of MSCs in different regions of the same alginate-based substrate (Freeman and Kelly, 2017). Alternatively, biomaterials can be formed by means of temperature, pH, or even magnetic fields (Kim et al., 2016). This ability adds another dimension of possibility for precise control of patterning biomaterial mechanics (Buwalda et al., 2014), by allowing the design of biomaterials with multiscale mechanical properties, such as spatiotemporal patterning of porosity or unique topographies independent of base material properties. While precise spatial control of biomaterial mechanics remains to be leveraged for tissue engineering applications using stem cells in general, temporal control of biomaterial mechanics to control stem cell differentiation has already been demonstrated by a number of studies using light-degradable hydrogels (Kloxin et al., 2009) to dynamically control stem cell fate (Yang et al., 2014; Rosales et al., 2017). Photodegradable hydrogels have also been recently implemented in the context of 3D printing (Arakawa et al., 2017), although 3D printed photodegradable hydrogels remain to be used to mechanically direct stem cell differentiation. Biomaterials with properties sensitive to temporal application of temperature, pH, and chemical or biological stimuli have been used in other contexts such as controlled release or sequestration of molecules (Buwalda et al., 2014). Enzymatic reactions have been used to degrade biomaterials over time, leading to a temporal control of matrix properties. Actuation of biomaterials by means of temperature, pH, electricity or magnetism is possible in principle and has been demonstrated to achieve low levels of temporal mechanical control, though this concept is yet to be applied in a system to direct iPSC differentiation (Leijten et al., 2017). Nevertheless, a recent study suggests that mechanical conditioning of early-stage iPSC-derived cardiomyocytes is critical to formation of mature human cardiac tissues (Ronaldson-Bouchard et al., 2018), indicating that temporal control of mechanical cues plays an important role in directing iPSCs to form functional tissues. Thus, spatiotemporal control of biomaterial mechanics presents a promising future direction to tailor iPSCs for engineering of tissues with multiple cell types and degrees of maturity.
Stem cell functions are strongly influenced by mechanical cues in the environment. Thus far, biomaterials have served a crucial role in tissue engineering by offering control of the environment and its mechanics to direct stem cell functions. The future of biomaterial strategies for improving applications of iPSCs in tissue engineering remains optimistic with a high ceiling for advancement. Due to recent advances in fields such as biomaterial design and stereolithography, research efforts are establishing biomaterial systems that allow extremely precise spatiotemporal control and presentation of mechanical cues. These systems can be designed to facilitate iPSC reprogramming, self-renewal, differentiation, and morphogenesis in a stepwise manner. Ideally, such platforms will be developed to function with minimal inputs from the end user once the primary cell-material interactions are initiated. Therefore, future challenges will involve mediating developmental transitions in a growingly diverse population of cells. Advances in material science and engineering combined with an understanding of developmental biophysics and epigenetic regulation will be critical to facilitate this modern engineering effort.
SL, DD, and J-WS wrote and contributed to the work.
This work was supported by National Institutes of Health Grant R01-HL141255 (J-WS), R00-HL125884 (J-WS), T32-HL07829 (SL), and American Heart Association Grant 19PRE34380087 (SL).
The authors declare that the research was conducted in the absence of any commercial or financial relationships that could be construed as a potential conflict of interest.
Abagnale, G., Steger, M., Nguyen, V. H., Hersch, N., Sechi, A., Joussen, S., et al. (2015). Surface topography enhances differentiation of mesenchymal stem cells towards osteogenic and adipogenic lineages. Biomaterials 61, 316–326. doi: 10.1016/j.biomaterials.2015.05.030
Ang, Y. S., Tsai, S. Y., Lee, D. F., Monk, J., Su, J., Ratnakumar, K., et al. (2011). Wdr5 mediates self-renewal and reprogramming via the embryonic stem cell core transcriptional network. Cell 145, 183–197. doi: 10.1016/j.cell.2011.03.003
Arai, F., Hirao, A., Ohmura, M., Sato, H., Matsuoka, S., Takubo, K., et al. (2004). Tie2/angiopoietin-1 signaling regulates hematopoietic stem cell quiescence in the bone marrow niche. Cell 118, 149–161. doi: 10.1016/j.cell.2004.07.004
Arakawa, C. K., Badeau, B. A., Zheng, Y., and Deforest, C. A. (2017). Multicellular vascularized engineered tissues through user-programmable biomaterial photodegradation. Adv. Mater. 29:1703156. doi: 10.1002/adma.201703156
Banks, J. M., Mozdzen, L. C., Harley, B. A., and Bailey, R.C. (2014). The combined effects of matrix stiffness and growth factor immobilization on the bioactivity and differentiation capabilities of adipose-derived stem cells. Biomaterials 35, 8951–8959. doi: 10.1016/j.biomaterials.2014.07.012
Becker, K. A., Ghule, P. N., Therrien, J. A., Lian, J. B., Stein, J. L., Van Wijnen, A. J., et al. (2006). Self-renewal of human embryonic stem cells is supported by a shortened G1 cell cycle phase. J. Cell. Physiol. 209, 883–893. doi: 10.1002/jcp.20776
Bhatlekar, S., Fields, J. Z., and Boman, B. M. (2018). Role of HOX genes in stem cell differentiation and cancer. Stem Cells Int. 2018:3569493. doi: 10.1155/2018/3569493
Buttery, L. D. K., Bourne, S., Xynos, J. D., Wood, H., Hughes, F. J., Hughes, S. P. F., et al. (2001). Differentiation of osteoblasts and in vitro bone formation from murine embryonic stem cells. Tissue Eng. 7, 89–99. doi: 10.1089/107632700300003323
Buwalda, S. J., Boere, K. W. M., Dijkstra, P. J., Feijen, J., Vermonden, T., and Hennink, W. E. (2014). Hydrogels in a historical perspective: from simple networks to smart materials. J. Control. Release 190, 254–273. doi: 10.1016/j.jconrel.2014.03.052
Caiazzo, M., Okawa, Y., Ranga, A., Piersigilli, A., Tabata, Y., and Lutolf, M. P. (2016). Defined three-dimensional microenvironments boost induction of pluripotency. Nat. Mater. 15:344. doi: 10.1038/nmat4536
Cameron, A. R., Frith, J. E., and Cooper-White, J. J. (2011). The influence of substrate creep on mesenchymal stem cell behaviour and phenotype. Biomaterials 32, 5979–5993. doi: 10.1016/j.biomaterials.2011.04.003
Castelli-Gair Hombría, J., and Lovegrove, B. (2003). Beyond homeosis - HOX function in morphogenesis and organogenesis. Differentiation 71, 461–476. doi: 10.1046/j.1432-0436.2003.7108004.x
Chan, V., Zorlutuna, P., Jeong, J. H., Kong, H., and Bashir, R. (2010). Three-dimensional photopatterning of hydrogels using stereolithography for long-term cell encapsulation. Lab Chip 10, 2062–2070. doi: 10.1039/c004285d
Chaudhuri, O., Gu, L., Klumpers, D., Darnell, M., Bencherif, S. A., Weaver, J. C., et al. (2016). Hydrogels with tunable stress relaxation regulate stem cell fate and activity. Nat. Mater. 15, 326–334. doi: 10.1038/nmat4489
Chen, J., Liu, H., Liu, J., Qi, J., Wei, B., Yang, J., et al. (2013). H3K9 methylation is a barrier during somatic cell reprogramming into iPSCs. Nat. Genet. 45, 34–42. doi: 10.1038/ng.2491
Cheung, T. H., and Rando, T. A. (2013). Molecular regulation of stem cell quiescence. Nat. Rev. Mol. Cell Biol. 14, 329–340. doi: 10.1038/nrm3591
Clevers, H. (2016). Modeling development and disease with organoids. Cell 165, 1586–1597. doi: 10.1016/j.cell.2016.05.082
Crest, J., Diz-Munoz, A., Chen, D. Y., Fletcher, D. A., and Bilder, D. (2017). Organ sculpting by patterned extracellular matrix stiffness. Elife 6:e24958. doi: 10.7554/eLife.24958
Dale, J. K., Malapert, P., Chal, J., Vilhais-Neto, G., Maroto, M., Johnson, T., et al. (2006). Oscillations of the snail genes in the presomitic mesoderm coordinate segmental patterning and morphogenesis in vertebrate somitogenesis. Dev. Cell 10, 355–366. doi: 10.1016/j.devcel.2006.02.011
Davidson, L. A. (2017). Mechanical design in embryos: mechanical signalling, robustness and developmental defects. Philos. Trans. R. Soc. Lond. B Biol. Sci. 372:20150516. doi: 10.1098/rstb.2015.0516
Deforest, C. A., and Tirrell, D. A. (2015). A photoreversible protein-patterning approach for guiding stem cell fate in three-dimensional gels. Nat. Mater. 14, 523–531. doi: 10.1038/nmat4219
Denais, C. M., Gilbert, R. M., Isermann, P., Mcgregor, A. L., Te Lindert, M., Weigelin, B., et al. (2016). Nuclear envelope rupture and repair during cancer cell migration. Science 352, 353–358. doi: 10.1126/science.aad7297
Deschamps, J., and van Nes, J. (2005). Developmental regulation of the Hox genes during axial morphogenesis in the mouse. Development 132, 2931–2942. doi: 10.1242/dev.01897
Ding, L., Saunders, T. L., Enikolopov, G., and Morrison, S. J. (2012). Endothelial and perivascular cells maintain haematopoietic stem cells. Nature 481, 457–462. doi: 10.1038/nature10783
Discher, D. E., Janmey, P., and Wang, Y. L. (2005). Tissue cells feel and respond to the stiffness of their substrate. Science 310, 1139–1143. doi: 10.1126/science.1116995
Downing, T. L., Soto, J., Morez, C., Houssin, T., Fritz, A., Yuan, F., et al. (2013). Biophysical regulation of epigenetic state and cell reprogramming. Nat. Mater. 12, 1154–1162. doi: 10.1038/nmat3777
Dupont, S., Morsut, L., Aragona, M., Enzo, E., Giulitti, S., Cordenonsi, M., et al. (2011). Role of YAP/TAZ in mechanotransduction. Nature 474, 179–183. doi: 10.1038/nature10137
Eiraku, M., Takata, N., Ishibashi, H., Kawada, M., Sakakura, E., Okuda, S., et al. (2011). Self-organizing optic-cup morphogenesis in three-dimensional culture. Nature 472, 51–56. doi: 10.1038/nature09941
Engler, A. J., Sen, S., Sweeney, H. L., and Discher, D. E. (2006). Matrix elasticity directs stem cell lineage specification. Cell 126, 677–689. doi: 10.1016/j.cell.2006.06.044
Ficara, F., Murphy, M. J., Lin, M., and Cleary, M. L. (2008). Pbx1 regulates self-renewal of long-term hematopoietic stem cells by maintaining their quiescence. Cell Stem Cell 2, 484–496. doi: 10.1016/j.stem.2008.03.004
Florian, M. C., Dorr, K., Niebel, A., Daria, D., Schrezenmeier, H., Rojewski, M., et al. (2012). Cdc42 activity regulates hematopoietic stem cell aging and rejuvenation. Cell Stem Cell 10, 520–530. doi: 10.1016/j.stem.2012.04.007
Fluckiger, A. C., Marcy, G., Marchand, M., Negre, D., Cosset, F. L., Mitalipov, S., et al. (2006). Cell cycle features of primate embryonic stem cells. Stem Cells 24, 547–556. doi: 10.1634/stemcells.2005-0194
Forgacs, G., and Newman, S. A. (2005). Biological Physics of the Developing Embryo. Cambridge: Cambridge University Press. doi: 10.1017/CBO9780511755576
Freeman, F. E., and Kelly, D. J. (2017). Tuning alginate bioink stiffness and composition for controlled growth factor delivery and to spatially direct MSC fate within bioprinted tissues. Sci. Rep. 7:17042. doi: 10.1038/s41598-017-17286-1
Freeman, M. R. (2010). Specification and morphogenesis of astrocytes. Science 330, 774–778. doi: 10.1126/science.1190928
Gjorevski, N., Sachs, N., Manfrin, A., Giger, S., Bragina, M. E., Ordonez-Moran, P., et al. (2016). Designer matrices for intestinal stem cell and organoid culture. Nature 539, 560–564. doi: 10.1038/nature20168
Gori, F., Friedman, L. G., and Demay, M. B. (2006). Wdr5, a WD-40 protein, regulates osteoblast differentiation during embryonic bone development. Dev. Biol. 15, 498–506. doi: 10.1016/j.ydbio.2006.02.031
Grespan, E., Giobbe, G. G., Badique, F., Anselme, K., Rühe, J., and Elvassore, N. (2018). Effect of geometrical constraints on human pluripotent stem cell nuclei in pluripotency and differentiation. Integr. Biol. 10, 278–289. doi: 10.1039/C7IB00194K
Grigoryan, A., Guidi, N., Senger, K., Liehr, T., Soller, K., Marka, G., et al. (2018). LaminA/C regulates epigenetic and chromatin architecture changes upon aging of hematopoietic stem cells. Genome Biol. 19:189. doi: 10.1186/s13059-018-1557-3
Guelen, L., Pagie, L., Brasset, E., Meuleman, W., Faza, M. B., Talhout, W., et al. (2008). Domain organization of human chromosomes revealed by mapping of nuclear lamina interactions. Nature 453, 948–951. doi: 10.1038/nature06947
Hammerschmidt, M., and Wedlich, D. (2008). Regulated adhesion as a driving force of gastrulation movements. Development 135, 3625–3641. doi: 10.1242/dev.015701
Harada, T., Swift, J., Irianto, J., Shin, J. W., Spinler, K. R., Athirasala, A., et al. (2014). Nuclear lamin stiffness is a barrier to 3D migration, but softness can limit survival. J. Cell. Biol. 204, 669–682. doi: 10.1083/jcb.201308029
Hettiaratchi, M. H., Guldberg, R. E., and McDevitt, T. C. (2016). Biomaterial strategies for controlling stem cell fate: via morphogen sequestration. J. Mater. Chem. B 4, 3464–3481. doi: 10.1039/C5TB02575C
Hiew, V. V., Simat, S. F. B., and Teoh, P. L. (2018). The advancement of biomaterials in regulating stem cell fate. Stem Cell Rev. 14, 43–57. doi: 10.1007/s12015-017-9764-y
Hou, P., Li, Y., Zhang, X., Liu, C., Guan, J., Li, H., et al. (2013). Pluripotent stem cells induced from mouse somatic cells by small-molecule compounds. Science 341, 651–654. doi: 10.1126/science.1239278
Huebsch, N., Arany, P. R., Mao, A. S., Shvartsman, D., Ali, O. A., Bencherif, S. A., et al. (2010). Harnessing traction-mediated manipulation of the cell/matrix interface to control stem-cell fate. Nat. Mater. 9, 518–526. doi: 10.1038/nmat2732
Ichida, J. K., Blanchard, J., Lam, K., Son, E. Y., Chung, J. E., Egli, D., et al. (2009). A small-molecule inhibitor of Tgf-β signaling replaces Sox2 in reprogramming by inducing nanog. Cell Stem Cell 5, 491–503. doi: 10.1016/j.stem.2009.09.012
Irianto, J., Xia, Y., Pfeifer, C. R., Athirasala, A., Ji, J., Alvey, C., et al. (2017). DNA damage follows repair factor depletion and portends genome variation in cancer cells after pore migration. Curr. Biol. 27, 210–223. doi: 10.1016/j.cub.2016.11.049
Jaenisch, R., and Young, R. (2008). Stem cells, the molecular circuitry of pluripotency and nuclear reprogramming. Cell 132, 567–582. doi: 10.1016/j.cell.2008.01.015
Jing, H., and Lin, H. (2015). Sirtuins in epigenetic regulation. Chem. Rev. 115, 2350–2375. doi: 10.1021/cr500457h
Khetan, S., Guvendiren, M., Legant, W. R., Cohen, D. M., Chen, C. S., and Burdick, J. A. (2013). Degradation-mediated cellular traction directs stem cell fate in covalently crosslinked three-dimensional hydrogels. Nat. Mater. 12, 458–465. doi: 10.1038/nmat3586
Kilian, K. A., Bugarija, B., Lahn, B. T., and Mrksich, M. (2010). Geometric cues for directing the differentiation of mesenchymal stem cells. Proc. Natl. Acad. Sci. U.S.A. 107, 4872–4877. doi: 10.1073/pnas.0903269107
Kim, H.-T., Lee, K.-I., Kim, D.-W., and Hwang, D.-Y. (2013). An ECM-based culture system for the generation and maintenance of xeno-free human iPS cells. Biomaterials 34, 1041–1050. doi: 10.1016/j.biomaterials.2012.10.064
Kim, J., Staunton, J. R., and Tanner, K. (2016). Independent control of topography for 3D patterning of the ECM microenvironment. Adv. Mater. 28, 132–137. doi: 10.1002/adma.201503950
Kinney, M. A., and Mcdevitt, T. C. (2013). Emerging strategies for spatiotemporal control of stem cell fate and morphogenesis. Trends Biotechnol. 31, 78–84. doi: 10.1016/j.tibtech.2012.11.001
Kloxin, A. M., Kasko, A. M., Salinas, C. N., and Anseth, K. S. (2009). Photodegradable hydrogels for dynamic tuning of physical and chemical properties. Science 324, 59–63. doi: 10.1126/science.1169494
Knoblich, J. A. (2008). Mechanisms of asymmetric stem cell division. Cell 132, 583–597. doi: 10.1016/j.cell.2008.02.007
Kowalczewski, C. J., and Saul, J. M. (2018). Biomaterials for the delivery of growth factors and other therapeutic agents in tissue engineering approaches to bone regeneration. Front. Pharmacol. 9:513. doi: 10.3389/fphar.2018.00513
Kulkarni, S., and Khokha, M. (2018). WDR5 regulates left-right patterning via chromatin-dependent and -independent functions. Development 145:159889. doi: 10.1242/dev.159889
Lee, H. P., Stowers, R., and Chaudhuri, O. (2019). Volume expansion and TRPV4 activation regulate stem cell fate in three-dimensional microenvironments. Nat. Commun. 10:529. doi: 10.1038/s41467-019-08465-x
Lee, J.-H., Park, H.-K., and Kim, K. S. (2016). Intrinsic and extrinsic mechanical properties related to the differentiation of mesenchymal stem cells. Biochem. Biophys. Res. Commun. 473, 752–757. doi: 10.1016/j.bbrc.2015.09.081
Lee, K., Silva, E. A., and Mooney, D. J. (2011). Growth factor delivery-based tissue engineering: general approaches and a review of recent developments. J. R. Soc. Interface 8, 153–170. doi: 10.1098/rsif.2010.0223
Leijten, J., Seo, J., Yue, K., Trujillo-de Santiago, G., Tamayol, A., Ruiz-Esparza, G. U., et al. (2017). Spatially and temporally controlled hydrogels for tissue engineering. Mater. Sci. Eng. R Rep. 119, 1–35. doi: 10.1016/j.mser.2017.07.001
Levenstein, M. E., Ludwig, T. E., Xu, R. H., Llanas, R. A., Vandenheuvel-Kramer, K., Manning, D., et al. (2006). Basic fibroblast growth factor support of human embryonic stem cell self-renewal. Stem Cells 24, 568–574. doi: 10.1634/stemcells.2005-0247
Li, J., and Mooney, D. J. (2016). Designing hydrogels for controlled drug delivery. Nat. Rev. Mater. 1:16071. doi: 10.1038/natrevmats.2016.71
Liang, G., and Zhang, Y. (2013). Embryonic stem cell and induced pluripotent stem cell: an epigenetic perspective. Cell Res. 23, 49–69. doi: 10.1038/cr.2012.175
Liberti, D. C., Zepp, J. A., Bartoni, C. A., Liberti, K. H., Zhou, S., Lu, M., et al. (2019). Dnmt1 is required for proximal-distal patterning of the lung endoderm and for restraining alveolar type 2 cell fate. Dev. Biol. 454, 108–117. doi: 10.1016/j.ydbio.2019.06.019
Lienemann, P. S., Devaud, Y. R., Reuten, R., Simona, B. R., Karlsson, M., Weber, W., et al. (2014). Locally controlling mesenchymal stem cell morphogenesis by 3D PDGF-BB gradients towards the establishment of an in vitro perivascular niche. Integr. Biol. 7, 101–111. doi: 10.1039/C4IB00152D
Lozoya, O. A., Gilchrist, C. L., and Guilak, F. (2016). Universally conserved relationships between nuclear shape and cytoplasmic mechanical properties in human stem cells. Sci. Rep. 6:23047. doi: 10.1038/srep23047
Maître, J.-L., Turlier, H., Illukkumbura, R., Eismann, B., Niwayama, R., Nédélec, F., et al. (2016). Asymmetric division of contractile domains couples cell positioning and fate specification. Nature 536:344. doi: 10.1038/nature18958
Mahadik, B. P., Pedron Haba, S., Skertich, L. J., and Harley, B. A. (2015). The use of covalently immobilized stem cell factor to selectively affect hematopoietic stem cell activity within a gelatin hydrogel. Biomaterials 67, 297–307. doi: 10.1016/j.biomaterials.2015.07.042
Maherali, N., Sridharan, R., Xie, W., Utikal, J., Eminli, S., Arnold, K., et al. (2007). Directly reprogrammed fibroblasts show global epigenetic remodeling and widespread tissue contribution. Cell Stem Cell 1, 55–70. doi: 10.1016/j.stem.2007.05.014
Majkut, S., Idema, T., Swift, J., Krieger, C., Liu, A., and Discher, D. E. (2013). Heart-specific stiffening in early embryos parallels matrix and myosin expression to optimize beating. Curr. Biol. 23, 2434–2439. doi: 10.1016/j.cub.2013.10.057
Mcbeath, R., Pirone, D. M., Nelson, C. M., Bhadriraju, K., and Chen, C. S. (2004). Cell shape, cytoskeletal tension, and RhoA regulate stem cell lineage commitment. Dev. Cell 6, 483–495. doi: 10.1016/S1534-5807(04)00075-9
Mulas, C., Chia, G., Jones, K. A., Hodgson, A. C., Stirparo, G. G., and Nichols, J. (2018). Oct4 regulates the embryonic axis and coordinates exit from pluripotency and germ layer specification in the mouse embryo. Development 145:159103. doi: 10.1242/dev.159103
Nava, M. M., Raimondi, M. T., and Pietrabissa, R. (2012). Controlling self-renewal and differentiation of stem cells via mechanical cues. J. Biomed. Biotechnol. 2012:797410. doi: 10.1155/2012/797410
Nicola, N. A., and Babon, J. J. (2015). Leukemia inhibitory factor (LIF). Cytokine Growth Factor Rev. 26, 533–544. doi: 10.1016/j.cytogfr.2015.07.001
Nieto, M. A. (2002). The snail superfamily of zinc-finger transcription factors. Nat. Rev. Mol. Cell Biol. 3, 155–166. doi: 10.1038/nrm757
Okuda, S., Takata, N., Hasegawa, Y., Kawada, M., Inoue, Y., Adachi, T., et al. (2018). Strain-triggered mechanical feedback in self-organizing optic-cup morphogenesis. Sci. Adv. 4:eaau1354. doi: 10.1126/sciadv.aau1354
Ouyang, L., Highley, C. B., Sun, W., and Burdick, J. A. (2017). A generalizable strategy for the 3D bioprinting of hydrogels from nonviscous photo-crosslinkable inks. Adv. Mater. 29:1604983. doi: 10.1002/adma.201604983
Pajerowski, J. D., Dahl, K. N., Zhong, F. L., Sammak, P. J., and Discher, D. E. (2007). Physical plasticity of the nucleus in stem cell differentiation. Proc. Natl. Acad. Sci. U.S.A. 104, 15619–15624. doi: 10.1073/pnas.0702576104
Poh, Y.-C., Chen, J., Hong, Y., Yi, H., Zhang, S., Chen, J., et al. (2014). Generation of organized germ layers from a single mouse embryonic stem cell. Nat. Commun. 5:4000. doi: 10.1038/ncomms5000
Przybyla, L., Lakins, J. N., and Weaver, V. M. (2016). Tissue mechanics orchestrate wnt-dependent human embryonic stem cell differentiation. Cell Stem Cell 19, 462–475. doi: 10.1016/j.stem.2016.06.018
Raab, M., Gentili, M., De Belly, H., Thiam, H. R., Vargas, P., Jimenez, A. J., et al. (2016). ESCRT III repairs nuclear envelope ruptures during cell migration to limit DNA damage and cell death. Science 352, 359–362. doi: 10.1126/science.aad7611
Reilly, G. C., and Engler, A. J. (2010). Intrinsic extracellular matrix properties regulate stem cell differentiation. J. Biomech. 43, 55–62. doi: 10.1016/j.jbiomech.2009.09.009
Rizwan, M., Peh, G. S. L., Ang, H. P., Lwin, N. C., Adnan, K., Mehta, J. S., et al. (2017). Sequentially-crosslinked bioactive hydrogels as nano-patterned substrates with customizable stiffness and degradation for corneal tissue engineering applications. Biomaterials 120, 139–154. doi: 10.1016/j.biomaterials.2016.12.026
Ronaldson-Bouchard, K., Ma, S. P., Yeager, K., Chen, T., Song, L., Sirabella, D., et al. (2018). Advanced maturation of human cardiac tissue grown from pluripotent stem cells. Nature 556, 239–243. doi: 10.1038/s41586-018-0016-3
Rosales, A. M., Vega, S. L., Delrio, F. W., Burdick, J. A., and Anseth, K. S. (2017). Hydrogels with reversible mechanics to probe dynamic cell microenvironments. Angew. Chem. Int. Ed. Engl. 56, 12132–12136. doi: 10.1002/anie.201705684
Rossant, J., and Tam, P. P. L. (2017). New insights into early human development: lessons for stem cell derivation and differentiation. Cell Stem Cell 1, 18–28. doi: 10.1016/j.stem.2016.12.004
Rowat, A. C., Jaalouk, D. E., Zwerger, M., Ung, W. L., Eydelnant, I. A., Olins, D. E., et al. (2013). Nuclear envelope composition determines the ability of neutrophil-type cells to passage through micron-scale constrictions. J. Biol. Chem. 288, 8610–8618. doi: 10.1074/jbc.M112.441535
Rozario, T., and Desimone, D. W. (2010). The extracellular matrix in development and morphogenesis: a dynamic view. Dev. Biol. 341, 126–140. doi: 10.1016/j.ydbio.2009.10.026
Ruijtenberg, S., and Van Den Heuvel, S. (2016). Coordinating cell proliferation and differentiation: antagonism between cell cycle regulators and cell type-specific gene expression. Cell Cycle 15, 196–212. doi: 10.1080/15384101.2015.1120925
Seto, S. P., Casas, M. E., and Temenoff, J. S. (2012). Differentiation of mesenchymal stem cells in heparin-containing hydrogels via coculture with osteoblasts. Cell Tissue Res. 347, 589–601. doi: 10.1007/s00441-011-1265-8
Shawky, J. H., Balakrishnan, U. L., Stuckenholz, C., and Davidson, L. A. (2018). Multiscale analysis of architecture, cell size and the cell cortex reveals cortical F-actin density and composition are major contributors to mechanical properties during convergent extension. Development 145:dev161281. doi: 10.1242/dev.161281
Shin, J. W., Buxboim, A., Spinler, K. R., Swift, J., Christian, D. A., Hunter, C. A., et al. (2014). Contractile forces sustain and polarize hematopoiesis from stem and progenitor cells. Cell Stem Cell 14, 81–93. doi: 10.1016/j.stem.2013.10.009
Shin, J. W., and Mooney, D. J. (2016). Improving stem cell therapeutics with mechanobiology. Cell Stem Cell 18, 16–19. doi: 10.1016/j.stem.2015.12.007
Shin, J. W., Spinler, K. R., Swift, J., Chasis, J. A., Mohandas, N., and Discher, D. E. (2013). Lamins regulate cell trafficking and lineage maturation of adult human hematopoietic cells. Proc. Natl. Acad. Sci. U.S.A. 110, 18892–18897. doi: 10.1073/pnas.1304996110
Smith, E. R., Meng, Y., Moore, R., Tse, J. D., Xu, A. G., and Xu, X. X. (2017). Nuclear envelope structural proteins facilitate nuclear shape changes accompanying embryonic differentiation and fidelity of gene expression. BMC Cell Biol. 18:8. doi: 10.1186/s12860-017-0125-0
Smith, Q., Rochman, N., Carmo, A. M., Vig, D., Chan, X. Y., Sun, S., et al. (2018). Cytoskeletal tension regulates mesodermal spatial organization and subsequent vascular fate. Proc. Natl. Acad. Sci. U.S.A. 115, 8167–8172. doi: 10.1073/pnas.1808021115
Smith, S. J., Rebeiz, M., and Davidson, L. (2018). From pattern to process: studies at the interface of gene regulatory networks, morphogenesis, and evolution. Curr. Opin. Genet. Dev. 51, 103–110. doi: 10.1016/j.gde.2018.08.004
Smith, Z. D., and Meissner, A. (2013). DNA methylation: roles in mammalian development. Nat. Rev. Genet. 14, 204–220. doi: 10.1038/nrg3354
Soo, K., O'Rourke, M. P., Khoo, P. L., Steiner, K. A., Wong, N., Behringer, R. R., et al. (2002). Twist function is required for the morphogenesis of the cephalic neural tube and the differentiation of the cranial neural crest cells in the mouse embryo. Dev. Biol. 247, 251–270. doi: 10.1006/dbio.2002.0699
Spicer, C. D., Pashuck, E. T., and Stevens, M. M. (2018). Achieving controlled biomolecule-biomaterial conjugation. Chem. Rev. 118, 7702–7743. doi: 10.1021/acs.chemrev.8b00253
Sun, Y., Villa-Diaz, L. G., Lam, R. H., Chen, W., Krebsbach, P. H., and Fu, J. (2012). Mechanics regulates fate decisions of human embryonic stem cells. PLoS ONE 7:e37178. doi: 10.1371/journal.pone.0037178
Swift, J., Ivanovska, I. L., Buxboim, A., Harada, T., Dingal, P. C., Pinter, J., et al. (2013). Nuclear lamin-A scales with tissue stiffness and enhances matrix-directed differentiation. Science 341:1240104. doi: 10.1126/science.1240104
Takahashi, K., Tanabe, K., Ohnuki, M., Narita, M., Ichisaka, T., Tomoda, K., et al. (2007). Induction of pluripotent stem cells from adult human fibroblasts by defined factors. Cell 131, 861–872. doi: 10.1016/j.cell.2007.11.019
Tan, Y., Tajik, A., Chen, J., Jia, Q., Chowdhury, F., Wang, L., et al. (2014). Matrix softness regulates plasticity of tumour-repopulating cells via H3K9 demethylation and Sox2 expression. Nat. Commun. 5:4619. doi: 10.1038/ncomms5619
Wang, J., Kaplan, J. A., Colson, Y. L., and Grinstaff, M. W. (2017). Mechanoresponsive materials for drug delivery: harnessing forces for controlled release. Adv. Drug Deliv. Rev. 108, 68–82. doi: 10.1016/j.addr.2016.11.001
Wang, P., Dreger, M., Madrazo, E., Williams, C. J., Samaniego, R., Hodson, N. W., et al. (2018). WDR5 modulates cell motility and morphology and controls nuclear changes induced by a 3D environment. Proc. Natl. Acad. Sci. U.S.A. 115, 8581–8586. doi: 10.1073/pnas.1719405115
Wang, Z., Kumar, H., Tian, Z., Jin, X., Holzman, J. F., Menard, F., et al. (2018). Visible light photoinitiation of cell-adhesive gelatin methacryloyl hydrogels for stereolithography 3D bioprinting. ACS Appl. Mater. Interfaces 10, 26859–26869. doi: 10.1021/acsami.8b06607
Watanabe, K., Ueno, M., Kamiya, D., Nishiyama, A., Matsumura, M., Wataya, T., et al. (2007). A ROCK inhibitor permits survival of dissociated human embryonic stem cells. Nat. Biotechnol. 25, 681–686. doi: 10.1038/nbt1310
Willerth, S. M., Rader, A., and Sakiyama-Elbert, S. E. (2008). The effect of controlled growth factor delivery on embryonic stem cell differentiation inside fibrin scaffolds. Stem Cell Res. 1, 205–218. doi: 10.1016/j.scr.2008.05.006
Wilson, A., Laurenti, E., and Trumpp, A. (2009). Balancing dormant and self-renewing hematopoietic stem cells. Curr. Opin. Genet. Dev. 19, 461–468. doi: 10.1016/j.gde.2009.08.005
Wysocka, J., Swigut, T., Milne, T. A., Dou, Y., Zhang, X., Burlingame, A. L., et al. (2005). WDR5 associates with histone H3 methylated at K4 and is essential for H3 K4 methylation and vertebrate development. Cell 121, 859–872. doi: 10.1016/j.cell.2005.03.036
Yang, C., Tibbitt, M. W., Basta, L., and Anseth, K. S. (2014). Mechanical memory and dosing influence stem cell fate. Nat. Mater. 13, 645–652. doi: 10.1038/nmat3889
Yoshihara, H., Arai, F., Hosokawa, K., Hagiwara, T., Takubo, K., Nakamura, Y., et al. (2007). Thrombopoietin/MPL signaling regulates hematopoietic stem cell quiescence and interaction with the osteoblastic niche. Cell Stem Cell 1, 685–697. doi: 10.1016/j.stem.2007.10.020
Zanotelli, M. R., Ardalani, H., Zhang, J., Hou, Z., Nguyen, E. H., Swanson, S., et al. (2016). Stable engineered vascular networks from human induced pluripotent stem cell-derived endothelial cells cultured in synthetic hydrogels. Acta Biomater. 35, 32–41. doi: 10.1016/j.actbio.2016.03.001
Zhang, J., Wilson, G. F., Soerens, A. G., Koonce, C. H., Yu, J., Palecek, S. P., et al. (2009). Functional cardiomyocytes derived from human induced pluripotent stem cells. Circ. Res. 104, e30–41. doi: 10.1161/CIRCRESAHA.108.192237
Zhang, X., Xu, B., Puperi, D. S., Yonezawa, A. L., Wu, Y., Tseng, H., et al. (2015). Integrating valve-inspired design features into poly(ethylene glycol) hydrogel scaffolds for heart valve tissue engineering. Acta Biomater. 14, 11–21. doi: 10.1016/j.actbio.2014.11.042
Zhang, Y., Sun, T., and Jiang, C. (2018). Biomacromolecules as carriers in drug delivery and tissue engineering. Acta Pharm. Sin. B 8, 34–50. doi: 10.1016/j.apsb.2017.11.005
Zheng, X., Hu, J., Yue, S., Kristiani, L., Kim, M., Sauria, M., et al. (2018). Lamins organize the global three-dimensional genome from the nuclear periphery. Mol. Cell 71, 802–815.e807. doi: 10.1016/j.molcel.2018.05.017
Keywords: biomaterial mechanics, induced pluripotent stem cells (iPS cells), mechanobiology of stem cells, mechanotransduction, extracellular matrix (ECM)
Citation: Lenzini S, Devine D and Shin J-W (2019) Leveraging Biomaterial Mechanics to Improve Pluripotent Stem Cell Applications for Tissue Engineering. Front. Bioeng. Biotechnol. 7:260. doi: 10.3389/fbioe.2019.00260
Received: 18 August 2019; Accepted: 26 September 2019;
Published: 10 October 2019.
Edited by:
Stephanie Michelle Willerth, University of Victoria, CanadaReviewed by:
Emilie Velot, Université de Lorraine, FranceCopyright © 2019 Lenzini, Devine and Shin. This is an open-access article distributed under the terms of the Creative Commons Attribution License (CC BY). The use, distribution or reproduction in other forums is permitted, provided the original author(s) and the copyright owner(s) are credited and that the original publication in this journal is cited, in accordance with accepted academic practice. No use, distribution or reproduction is permitted which does not comply with these terms.
*Correspondence: Jae-Won Shin, c2hpbmp3QHVpYy5lZHU=
Disclaimer: All claims expressed in this article are solely those of the authors and do not necessarily represent those of their affiliated organizations, or those of the publisher, the editors and the reviewers. Any product that may be evaluated in this article or claim that may be made by its manufacturer is not guaranteed or endorsed by the publisher.
Research integrity at Frontiers
Learn more about the work of our research integrity team to safeguard the quality of each article we publish.