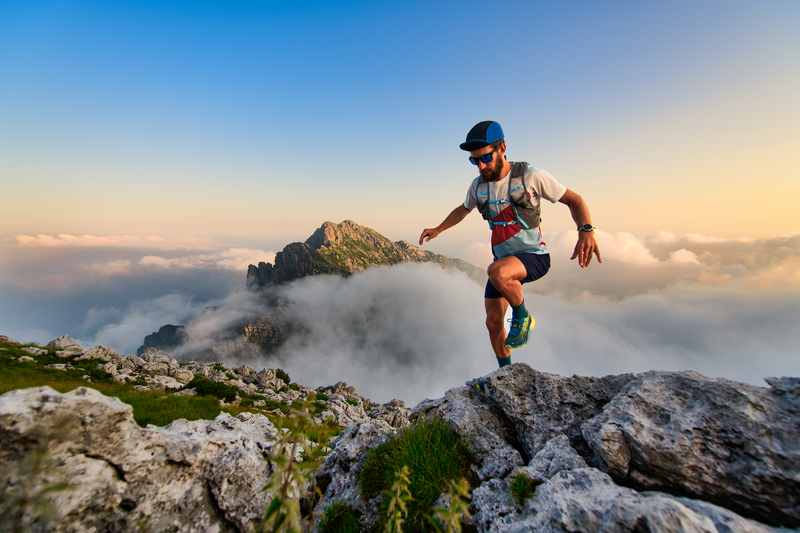
94% of researchers rate our articles as excellent or good
Learn more about the work of our research integrity team to safeguard the quality of each article we publish.
Find out more
ORIGINAL RESEARCH article
Front. Bioeng. Biotechnol. , 21 December 2018
Sec. Bioprocess Engineering
Volume 6 - 2018 | https://doi.org/10.3389/fbioe.2018.00196
The economically efficient utilization of NAD(P)H-dependent enzymes requires the regeneration of consumed reduction equivalents. Classically, this is done by substrate supplementation, and if necessary by addition of one or more enzymes. The simplest method thereof is whole cell NADPH regeneration. In this context we now present an easy-to-apply whole cell cofactor regeneration approach, which can especially be used in screening applications. Simply by applying citrate to a buffer or directly using citrate/-phosphate buffer NADPH can be regenerated by native enzymes of the TCA cycle, practically present in all aerobic living organisms. Apart from viable-culturable cells, this regeneration approach can also be applied with lyophilized cells and even crude cell extracts. This is exemplarily shown for the synthesis of 1-phenylethanol from acetophenone with several oxidoreductases. The mechanism of NADPH regeneration by TCA cycle enzymes was further investigated by a transient isotopic labeling experiment feeding [1,5-13C]citrate. This revealed that the regeneration mechanism can further be optimized by genetic modification of two competing internal citrate metabolism pathways, the glyoxylate shunt, and the glutamate dehydrogenase.
β-Nicotinamide adenine dinucleotide phosphate (NADPH) is an obligatory frequent cofactor for oxidoreductases, an important enzyme class in a manifold of academically and industrially relevant reactions (Straathof et al., 2002; Burton, 2003; Xu, 2005; Goldberg et al., 2007; Zheng et al., 2017). Unfortunately, NADPH is consumed in stoichiometric ratios during these reactions. An external NADPH addition is economically unfeasible, because it is a metastable and expensive molecule (Faber, 2011; Wu et al., 2013). Hence, several biological in vitro methods for cofactor regeneration (including also NADH) have been developed by using: (I) whole cell biocatalysis, (II) enzyme-coupled, or (III) substrate-coupled cofactor regeneration (van der Donk and Zhao, 2003; Wu et al., 2013; Marpani et al., 2017).
In the substrate coupled cofactor regeneration the backward reaction of a single NAD(P)H-dependent reduction is utilized by oxidizing a second auxiliary substrate under NAD(P) consumption (Kara et al., 2014; Marpani et al., 2017). However, the utilization of the backwards reaction lowers the catalytic activity of the enzyme of interest for the target reaction. An enzyme-coupled method supplements an additional enzyme together with a suitable co-substrate to the reaction for NADPH regeneration (Liese and Villela Filho, 1999; Goldberg et al., 2007; Hall and Bommarius, 2011; Rauter et al., 2015). Advantageously, this method allows the enzyme of interest to operate at full catalytic activity. Yet, the supplementation of an additional enzyme also increases process costs and is less economically feasible (Tufvesson et al., 2013).
Whole cell NADPH regeneration utilizes intracellular enzymes of the host organism (Wichmann and Vasic-Racki, 2005). Thereby, whole cell regeneration intrinsically inherits the advantages of an enzyme coupled approach, which are here combined with an inexpensive enzyme production. While several NADPH regenerating reactions are available in Escherichia coli, commonly only those associated with the central carbon metabolism of E. coli are considered in in vitro whole cell NADPH regeneration approaches. These are in particular glucose-6-phosphate dehydrogenase, glucose dehydrogenase, and isocitrate dehydrogenase (IDH) (Wichmann and Vasic-Racki, 2005; Blank et al., 2010; Hummel and Gröger, 2014; Spaans et al., 2015). Though the first two enzymes are most often utilized, an IDH application would be highly interesting due to its central metabolic position and high affinity for NADPH (Schwaneberg et al., 2001).
As such IDH found already an application in in vitro screening methods of different NADPH-dependent cytochrome P450 variants (Schwaneberg et al., 2001). In short, the cultivated whole cells were placed in an in-vitro environment together with the particular substrate, isocitrate, NADP+, dimethoxy-sulfoxide (DMSO) and polymyxin B sulfate. Polymyxin B sulfate was added to permeabilize the cells, while DMSO is supplemented to increase substrate solubility. The intracellular isocitrate dehydrogenase (IDH) of the host E. coli was utilized to regenerate NADPH by adding the expensive specialty chemical isocitrate to the system (Schwaneberg et al., 2001). Isocitrate is converted in one physiological step to 2-oxoglutarate, reducing NADP+ to NADPH. Likewise, other screening approaches have been developed, but they all suffer either from cost intensive NADP+ supplementation or require further additives to avoid diffusion limitation over the cell membrane. The latter might also impair the targeted enzyme activity (Klibanov, 1997; Faber, 2011; Gerhards et al., 2012; Bornadel et al., 2016).
Building on this research, we present a whole cell NADPH regeneration in a targeted oxidoreductase reaction with the mundane bulk chemical citrate, which is converted by endogenous tricarboxylic acid (TCA) cycle enzymes. For this purpose, the specific activity of a target oxidoreductase reaction toward acetophenone was investigated in presence of citrate, when the enzyme is formulated as lyophilized whole cells (LWC) and crude cell extract (CCE) (Figure 1). Citrate is hypothesized to be isomerized by aconitase to isocitrate. Isocitrate is then decarboxylated by IDH to 2-oxoglutarate and thereby NADP+ is reduced to NADPH (Blank et al., 2010; Spaans et al., 2015). In this context it was investigated if native TCA enzyme levels are sufficient for a NADPH regeneration from citrate. Additionally, a transient isotopic labeling experiment with [1,5-13C]citrate was recorded to evaluate if citrate truly is converted along the proposed pathway and if competing side reactions occur. Citrate was also investigated as buffer substance with NADPH-regenerating activity to emphasize a possible application in NADPH-dependent enzyme screenings, without the need of further additives. To verify the global transferability of this proposed system, we investigated it with three different alcohol dehydrogenases, which were all heterologously introduced into E. coli. These were ketoreductase 1 from Oogatea glycozyma (KRED1-Pglu), alcohol dehydrogenase from Ralstonia sp. (RADH), and alcohol dehydrogenase from Lactobacillus brevis (LbADH).
Figure 1. Citrate-dependent cofactor regeneration approach for screening NADPH-dependent oxidoreductases. Acetophenone is converted to 1-phenylethanol. In the reaction NADPH is consumed, which is proposed to be regenerated by the tricarboxylic acid (TCA) cycle enzymes present in whole cells or crude cell extract. Citrate is isomerized to isocitrate and then further converted by the enzyme isocitrate dehydrogenase (IDH). In this process IDH regenerates NADPH.
All applied chemicals were purchased from Sigma Aldrich (Germany), if specified in purities ≥95%. The cell disruption by sonification was performed on a UP 200s ultrasonic processor (Hielscher Ultrasonics GmbH, Teltow, Germany) with an S1 sonotrode (Hielscher Ultrasonics GmbH, Teltow, Germany). The reactions were incubated in an Eppendorf thermomixer comfort (Eppendorf, Hamburg, Germany) and samples were centrifuged in an Eppendorf centrifuge 5424 (Eppendorf, Hamburg, Germany). Sample analysis was performed on an Agilent technologies 1260 infinity high performance liquid chromatography system (Agilent Technologies, Santa Clara, CA, USA). Metabolic profile analysis was performed on an Agilent Technologies 6890N gas chromatography system (Agilent Technologies, Santa Clara, CA, USA) coupled to a GCT Premier mass spectrometer (Waters Corporation, Milford, MA, USA). Pipetting assistance was provided by Tecan Freedom Evo 1 (Tecan, Männedorf, Swiss).
Ogataea glucozyma CBS 5766 ketoreductase 1 (KRED1-Pglu; GenBank: AKP95857.1) on a pET-26b(+) vector, Ralstonia species alcohol dehydrogenase (RADH; PDB: 4BMS_A) on a pET-21a vector and Lactobacillus brevis alcohol dehydrogenase (LbADH; GenBank: CAD66648.1) on a pET-22b vector were heterologously expressed in E. coli BL21 (DE3) (see Supplementary S1). All were cultivated in 1 L auto induction medium at 37°C and 90 rpm (Studier, 2005). After 4 h of cultivation, temperature was reduced to 20°C. Cells were harvested after 72 h by centrifugation at 7,000 g for 45 min at 4°C. Harvested cells were further processed to CCE. To prepare a CCE, the pellets were resuspended in 50 mM KPi buffer pH 7.5, supplied with 0.1 mM MgCl2. Next, the cells were disrupted by sonication at an amplitude of 40 μm, and a cycle time of 0.5 s for 40 min at 4 to 8 °C. The solution was centrifuged at 8,000 g at 4°C for 45 min. The supernatant was transferred into a crystallization bowl and lyophilized at −54°C and 0.10 mbar. Lyophilized extract was mortared and stored at −20°C. The LWC catalyst was produced analogously, except cell lysis was not performed.
All experiments below were conducted in a reaction volume of 1 mL. The activity of employed lyophilized whole cell (LWC) KRED1-Pglu was 0.1 U mg−1 and for the corresponding CCE 0.4 U mg1 in presence of 5 mM acetophenone and 10 mM citrate. LbADH LWC had an activity of 1.61 U mg−1 and as CCE 3.54 U mg−1. In case of RADH the LWC had an activity of 0.81 U mg−1 and for CCE 1.78 U mg−1. 1 U is defined 1 μmol acetophenone that is converted per min by the indicated enzyme amount at defined reaction conditions of 5 mM acetophenonen and 10 mM citrate.
KRED1-Pglu reaction solutions contained 5 mM acetophenone, 0.1% (v/v) DMSO and 10 mM citrate suspended in 100 mM KPi buffer pH 8. Six different conditions were tested: (I) 20 mg mL−1 LWC, (II) 20 mg mL−1 CCE, (III) 20 mg mL−1 LWC with 0.5 mM NADPH as first positive control, (IV) 20 mg mL−1 CCE with 0.5 mM NADPH as second positive control, (V) 20 mg mL−1 LWC as negative control without citrate addition, and (VI) 5 mg mL−1 purified enzyme. The whole setup was analogously performed with RADH at pH 8 and LbADH at pH 6.5. Reactions were incubated at 30°C and 1,000 rpm. Samples were prepared and analyzed as described below. The activity toward acetophenone was analyzed via HPLC. Experiments were conducted in triplets.
A basic reaction solution was prepared containing 20 mg mL−1 KRED1-Pglu CCE in 100 mM KPi buffer pH 8 with 5 mM acetophenone, 0.1% (v/v) DMSO, and 10 mM citrate. This basic solution was tested (i) alone, (ii) with 1 U mL−1 isocitrate dehydrogenase (IDH), (iii) with 1 U mL−1 aconitase, (iv) with both enzymes supplemented to the reaction solution, and (v) with 0.5 mM NADP+ supplemented. (vi) As negative control, the reaction solution was prepared without citrate and enzyme addition. The reaction was incubated at 30°C and 1,000 rpm. The screening was analogously performed for 20 mg mL−1 RADH under the same reaction conditions and 20 mg mL−1 LbADH with the KPi buffer set to pH 6.5. All experiments in this section were produced in triplicates and pipetted with the aid of a robotic station. Samples were prepared and analyzed as described below. The activity toward acetophenone was analyzed via HPLC. Experiments were carried out in triplets.
20 mg mL−1 KRED1-Pglu CCE were added to 40 mM KPi buffer pH 8 with 10 mM citrate acid-1,5-13C, 5 mM acetophenone, and 0.1% (v/v) DMSO. The reaction solution was incubated at 30°C and 1,000 rpm. Samples were taken after 0, 1, 2, 3, 4, and 5 h. A second reaction solution was prepared with 20 mg mL−1 LbADH CCE in 20 mM citrate acid-1,5-13C phosphate buffer pH 6.5 and 5 mM acetophenone (McIlvaine, 1921). Samples were taken after 0, 10, 20, and 30 min. A negative control of the LbADH reaction was prepared, applying the same conditions, without citrate addition. Samples were taken after 0 and 30 min. All samples were centrifuged at 20,000 g and 130 μL of the supernatant were transferred into a 1.5 mL reaction vessel, frozen in liquid nitrogen and then stored at −20°C. The samples were further processed for GC-MS metabolic profile analysis as described below. Measurements were performed in singlets.
Citrate phosphate buffer was prepared by combining defined amounts of 200 mM K2HPO4 with 100 mM citrate solution. The pH of CPi is adjusted by the ratio of the two solutions to each other (McIlvaine, 1921). 20 mg mL−1 KRED1-Pglu CCE was added to buffered solutions that all contained 5 mM acetophenone and 0.1% (v/v) DMSO, but differed in source and concentration as follows: (i) 20 mM citrate phosphate buffer pH 8, (ii) 100 mM citrate phosphate buffer pH 8, and (iii) 100 mM KPi buffer pH 8 containing 10 mM citrate. The reaction solutions were incubated at 30°C and 1,000 rpm. The experimental setup was repeated analogously with RADH at pH 8 and LbADH at pH 6.5. The activity toward acetophenone was analyzed via HPLC. Measurements were performed in triplets.
Quantitative analysis was performed to determine initial activity rates in the range of maximum 10% of substrate conversion toward acetophenone on an HPLC. In case of LbADH, samples were taken after 0, 5, 10, 20, 30, and 40 min. In case of RADH and KRED1-Pglu, samples were taken after 0, 30, 60, 120, 150, and 180 min. Samples were diluted 1:25 in acetonitrile containing 100 μL L−1 toluene as internal standard and subsequently analyzed with a ChiralPak ID column (5 μm, 4.6 × 250 mm) at a flow of 1.0 mL min−1 under isocratic conditions of 50% acetonitrile and 50% water (purified by reverse osmosis, MilliQ) at 20°C. Retention times: 1-phenylethanol: 3.3 min (215 nm); acetophenone: 5.4 min (250 nm); toluene: 6.3 min (215 nm).
Qualitative untargeted GC-MS metabolic profile analysis was carried out under the same conditions as described by Paczia et al. (2012).
In aerobic organisms citrate is oxidized in the TCA cycle, while regenerating redox-equivalents. In this study, we investigated if this pathway can also be accessed in vitro in LWC to provide NADPH for a targeted oxidoreductase reaction. Since citrate uptake in E. coli is administered by a membrane transporter CitT, also crude cell extract (CCE) was investigated to rule out a substrate transport limitation (Pos et al., 1998). To ensure an independency of obtained results from a studied alcohol dehydrogenase, the experimental setup was investigated with KRED1-Pglu, LbADH, and RADH individually. As model reaction for all three oxidoreductases served the reduction of acetophenone to 1-phenylethanol under NADPH consumption (herein called target reaction; Figure 1; Leuchs and Greiner, 2011; Kulig et al., 2013; Contente et al., 2015, 2016). If citrate is converted by endogenous TCA cycle enzymes NADPH would be generated and thus a 1-phenylethanol formation would be observable. The possibility of endogenous NADPH levels to suffice for catalytic activity or other endogenous sources to regenerate NADPH was investigated by a LWC setup with acetophenone, but without citrate addition. The possibility that citrate could also be converted by the applied alcohol dehydrogenases was ruled out by performing the model reaction with purified KRED1-Pglu, LbADH, and RADH individually. Also NADPH was supplemented instead of citrate to rule out a limitation of available NADPH.
In the results all three targeted oxidoreductases were active in LWC and CCE formulation when citrate was present and reduced acetophenone (Figure 2). In absence of citrate LWCs did not convert any acetophenone, which proves that citrate is neither substrate of the selected oxidoreductases nor does citrate directly reduce NADP+. Moreover, the reaction with purified enzyme also did not display any activity, which demonstrates that citrate is not converted by the target oxidoreductase to gain NADPH. Together this indicates strongly, that citrate is converted by endogenous TCA cycle enzymes of both the CCE and LWC. More importantly, it verifies that citrate is applicable in vitro as inexpensive cosubstrate to achieve good product conversion by whole cell NADPH regeneration.
Figure 2. Citrate operates as redox-equivalent regenerating agent in product synthesis. The capability of citrate to regenerate NADPH in a targeted oxidoreductase reaction was investigated with Ogataea glucozyma CBS 5766 ketoreductase1 (KRED1-Pglu), Lactobacillus brevis alcohol dehydrogenase (LbADH), and Ralstonia sp. alcohol dehydrogenase (RADH) as lyophilized whole cell (LWC) and crude cell extract (CCE) catalysts. Therefore, citrate was provided to regenerate through endogenous tricarboxylic acid cycle enzyme NADPH, which is consumed by the targeted oxidoreductases in the reduction of acetophenone. Only the “negative control purified enzyme” contained the oxidoreductases in purified form instead of LWC/CCE. The “negative control LWC”: contained all compounds except citrate. The reaction setups with “0.5 mM NADPH” contained NADPH instead of citrate. n = 3.
A more detailed look at relative initial activities under the investigated conditions demonstrates CCEs to be more active than LWCs (Figure 3). This indicates indeed mass transport of citrate as limiting to some extent. A prior cell lysis to synthesis application may thus circumvent such a limitation and make an application of CCE recommendable.
Figure 3. Relative initial activities gained by whole cell regeneration from citrate. The capability of citrate to regenerate NADPH in a targeted oxidoreductase reaction was investigated with (A) Ogataea glucozyma CBS 5766 ketoreductase1 (KRED1-Pglu), (B) Lactobacillus brevis alcohol dehydrogenase (LbADH), and (C) Ralstonia sp. alcohol dehydrogenase (RADH) as lyophilized whole cell (LWC) and crude cell extract catalysts. The “negative control purified enzyme” contained the oxidoreductases in purified form instead of LWC/CCE. The “negative control LWC”: contained all compounds except citrate. The reaction setups with “0.5 mM NADPH” contained NADPH instead of citrate. 0.10 U mg−1 KRED1-Pglu LWC, 1.61 U mg−1 LbADH LWC, and 0.81 U mg−1 RADH LWC were normalized to 100% specific activity. n = 3.
An additional observation shows higher initial activities by direct NADPH supplementation than with CCE (Figure 3). This indicates that under citrate addition the reaction is limited in KM for NADPH. For this matter three factors are conceivable: (i) endogenous NADP+ amounts are too low, (ii) the present TCA cycle enzymes do not suffice to regenerate NADPH fast enough, and (iii) untargeted side-reactions in the CCE and LWC setup may compete with the targeted oxidoreductase reaction for NADPH. In all three cases an excess of NADPH saturates the demand of all NADPH-dependent enzymes in the reaction, allowing the targeted oxidoreductases to operate at Vmax.
In conclusion these first findings demonstrate citrate as a well applicable, inexpensive cosubstrate for NADPH regeneration during product synthesis. A detailed analysis of initial activities shows that a mass transport limitation for citrate reduces activities in LWCs. Hence, an application of this NADPH regeneration method would be preferred in CCE. During initial activity analysis it was also observed that all investigated enzymes do not operate at Vmax, which was revealed by the addition of 0.5 mM NADPH. Subsequently, the reason for this limitation is investigated on a cellular basis. Here, three different circumstances were proposed as limiting an operation at Vmax: (i) too low endogenous NADP+ levels, (ii) too low TCA cycle enzyme expression, or (iii) untargeted side-reactions that deplete NADPH.
First the potential of an inherent limitation caused by either low basal levels of the involved TCA cycle enzymes aconitase and IDH (Figure 1) or insufficient endogenous NADP+ levels was investigated. To investigate the first case, both enzymes were supplemented in CCE experiments individually as well as in combination (Figure 4). For the second case 0.5 mM NADP+ were supplemented to a CCE setup. The negative control neither contained citrate nor any supplemented aconitase, IDH, or NADP+. As positive control the CCE of each of the three oxidoreductases KRED1-Pglu, LbADH, and RADH, was added to a buffered reaction solution which contained acetophenone and citrate, but no additional enzymes.
Figure 4. Limitation analysis based on endogenous NADP+ amounts and endogenous TCA cycle enzymes. In an applied CCE setup the reduction of acetophenone by (A) Ogataea glucozyma CBS 5766 ketoreductase 1 (KRED1-Pglu), (B) Lactobacillus brevis alcohol dehydrogenase (LbADH), and (C) Ralstonia sp. alcohol dehydrogenase (RADH) was investigated in presence of citrate. To identify a possible limitation by endogenous TCA cycle enzymes 1 U mL−1 aconitase and 1 U mL−1 isocitrate dehydrogenase (IDH) were added individually and together. Further also the possibility of limiting NADP+ amounts was investigated by supplementation thereof. The negative control (neg. control) contained neither additional enzymes for cofactor regeneration nor citrate; 0.34 U mg−1 KRED1-Pglu CCE, 3.56 U mg−1 LbADH CCE, and 2.4 U mg−1 RADH CCE were normalized to 100% specific activity. n = 3.
The recorded initial activities of the positive control and supplemented aconitase and IDH both individually and combined were approximately equally fast (Figure 4). This indicates endogenous enzyme levels as indeed sufficient for citrate metabolism. Literature supports this claim as IDH is naturally well overexpressed in E. coli (Vasquez and Reeves, 1979; Schwaneberg et al., 2001).
The supplementation of NADP+ also did not affect any activity increase (Figure 4). This indicates NADP+ also as not limiting in the setup. The finding is reinforced by an estimation of endogenous NADP+ amounts based on literature values published by Bennett et al. (2009) and Chemler et al. (2010). Based on their values NADP+/NADPH molecules were estimated to be in excess by a magnitude of 107 to the targeted oxidoreduteases (estimation see Supplementary S3.1).
Together these findings rule out a possibility of a limitation caused by insufficient TCA enzymes to regenerate NADPH. Further, intrinsically provided NADP+ levels are abundant enough as to not limit catalytic rates of the targeted oxidoreductase reactions. Thus, the potential of undesired side reaction as cause for the observed limitation has to be investigated.
To identify if citrate is funneled into side reactions a transient isotopic labeling experiment with 13C-labeled citrate was performed. Acetophenone and [1,5-13C]citrate were added to a buffered reaction mixture with LbADH CCE catalyst (for the same experiment performed with KRED1-Pglu see Supplementary S2.3). A GC-ToF-MS analysis recorded the consumption of citrate, the formation of 1-phenylethanol and, most importantly, for the specific labeling enrichment in several TCA cycle intermediates downstream of citrate. Any further implications from citrate conversion, which did not directly affect NADPH regeneration, are beyond the scope of this study and were thus not considered.
In principle, three proximate competing routes to the conversion of citrate along the TCA cycle are conceivable (Figure 5): (i) 2-oxoglutarate is partly reduced to glutamate by glutamate dehydrogenase (GDH); (ii) isocitrate is partly split into succinate and glyoxylate, which both are further converted to malate and (iii) glyoxylate is partly reduced to glycolate by glyoxylate reductase (GR; Ornston and Ornston, 1969; Csonka and Fraenkel, 1976; Spaans et al., 2015).
Figure 5. Potential routes of citrate metabolism in Escherichia coli BL21 crude cell extract. The 13C-labeling introduced via citrate is distributed among the depicted intermediates, depending on which enzymes are active under CCE conditions. All four routes contribute differently to NADPH regeneration. The first step of the TCA cycle, the condensation of oxaloacetate and acetyl-CoA to citrate is presumably not active in CCE.
Ideally, only the TCA cycle is involved in citrate metabolization, because only by this mode NADPH is regenerated in the targeted manner by IDH. Accordingly, 13C-labeling would be introduced into the TCA cycle intermediates and malate would accumulate. In the first competing case, the GDH reaction would partly consume the NADPH formed by IDH, resulting in an overall lowered NADPH regeneration capacity and an accumulation of glutamate. In the second case, NADPH regeneration by IDH would be partly skipped and, again, accumulation of malate is expected. Finally, the third case would be the worst one, since the GR reaction would directly compete for the available NADPH pool without adding any reduction equivalents to it (Figure 5).
From the total ion chromatograms (TIC) it can be seen that citrate is completely converted within the first 20 min of the reaction (Figure 6). In the same pattern the product concentration of the target reaction, 1-phenylethanol, increases. The TCA cycle intermediate succinate was already present at the start of the reaction and no significant change was observed. By contrast, malate and glutamate strongly accumulated along the CCE experiment. For glycolate, only a minor elevation was recorded over time (Figure 6). The corresponding negative control, which contained all reactants like the reaction solution except for citrate, did not exhibit any product formation and no significant elevation of TCA cycle intermediates (Supplementary S2.2).
Figure 6. Total ion chromatograms (TIC) of selected TCA cycle intermediates. Relative concentration changes during the isotopic labeling experiment with [1,5-13C]citrate in Lactobacillus brevis alcohol dehydrogenase (LbADH) crude cell extract are shown (for corresponding mass spectrometic chromatogram of all detected metabolites see Supplementary S2.1 and for corresponding results of KRED1-Pglu see Supplementary S2.3).
The obtained TIC findings suggest all presented pathways for citrate metabolism (Figure 5) to be indeed active under CCE conditions. Foremost, the observation of 1-phenylethanol in the reaction solution (Figure 6) and its absence in the negative control (Supplementary S2.2) substantiates the theory of an active TCA cycle, because required NADPH can only be gained by a conversion through IDH. The accumulation of glutamate and glycolate also indicates a presence of side-reactions caused by GR and GDH, which lower NADPH amounts for the target oxidoreductase reaction. To validate an occurrence of these side reactions the transition of the 13C labeled carbon atom in the metabolite profile was investigated.
In extracted ion chromatograms (XIC) the distinct mass fragments for the unlabeled and 13C labeled metabolites were tracked. Firstly, unlabeled succinate dissipates over the reaction time, while 13C-labeling from citrate is introduced via the TCA cycle and glyoxylate shunt (Figure 7). This indicates a continuous turnover of the succinate pool, leading to a stable metabolite level during the CCE experiment (Figure 5). For malate and glutamate, the fractions of 13C-labeled and unlabeled species elevated to an equal extent, indicating that both metabolites represent dead-end pools for (i) citrate metabolism along the TCA cycle, (ii) the GDH by-pass, and (iii) the glyoxylate shunt. Thus, the selected XIC verifies all three citrate pathway as active. Yet, it did not grant quantitative information on the distribution ratio of citrate metabolism between the three pathways. An estimation of the extent of the GDH by-pass and the glyoxylate shunt is nonetheless possible with literature values. In the gloxylate shunt acetyl-CoA is required to obtain malate (Figure 5). Based on literature values the acetyl-CoA concentration in the setup can be estimated to range of 0.04 to 0.03 mM (estimation Supplementary S3.2; Bennett et al., 2009). Accordingly, only 0.4% of citrate would dissipate in this pathway. This estimation adjoined with the insignificant findings of GDH product in the TIC chromatogram indicates the glyoxylate shunt as dissipation source for citrate as negligible. In case of glutamate such an estimation is not possible and any ratio on the loss would be speculative. Yet, E. coli is a strong glutamate producer, which is indicated by the fact that the most abundant intracellular E. coli metabolite is glutamate (Bennett et al., 2009). A loss in this side reaction could be substantial in dependence of available NH3, which is unfortunately unknown. The ratio of this side reactions could be clarified in the future with a [2,4-13C]citrate tracer, which gives a distinct 13C-labeling pattern for the origin of each metabolite (see Supplementary S4).
Figure 7. Extracted ion chromatogram (XIC) of selected TCA cycle intermediates. The accumulation and dissipation of 12C and 13C labeled species during the isotopic labeling experiment with [1,5-13C]citrate in Lactobacillus brevis alcohol dehydrogenase (LbADH) crude cell extract are displayed (corresponding results of KRED1-Pglu see Supplementary S2.4).
In summary, the transient 13C isotope labeling verified the theory of NADPH regeneration through TCA cycle enzymes. However, the citrate metabolism into the glyoxylate shunt, glycolate synthesis, and glutamate synthesis were identified as competing side reactions (Figure 5). The glyoxylate shunt and glycolate synthesis was determined of minor to insignificant importance in the citrate depletion, because required intracellular acetyl-CoA levels are estimated to be too low to substantially promote a conversion of citrate through this pathway. The extent of NADPH loss by glutamate could unfortunately not be quantified, as the concentration of ammonia in the setup was unknown. Yet, all herein obtained findings indicate glutamate synthesis as limitation cause for NADPH regeneration efficiency. Interestingly, by the sheer quantity of present targeted oxidoreductase to all other host enzymes it could be assume to be more likely that the majority of NADPH is consumed by the target oxidoreductase. Acetophenone, however, is an artificial substrate for the targeted oxidoreductases (KRED1-Pglu, LbADH, RADH), while citrate and the metabolites of the TCA cycle are natural substrates of the primary metabolism. Thus, it can be speculated that the host enzymes and in this particular case the GDH exhibit a higher NADPH turnover rate than the targeted oxidoreductase. In future applications this issue could be solved by genetically engineering the efficiency of the glutamate synthesis or introducing an ammonium limitation that would slow GDH synthesis and thereby the depletion of NADPH. Also the inactivation of the glyoxylate shunt in E. coli by cultivating it on glucose as sole carbon and energy source could enhance the NADPH regeneration efficiency from citrate (Noronha et al., 2000; Phue and Shiloach, 2004; Phue et al., 2005).
In summary these gained findings illustrate very well that citrate is capable to provide NADPH to target product conversions. An application in investigation of initial activity rates is presently limited by the reported side reactions. However, a relative initial activity comparison may already be applicable, and implementation of necessary genetic alterations could unfold the full potential of this method.
The potential for initial activity comparison by this method could be increased by applying citrate as in vitro reaction environment in form of citrate-phosphate (CPi) buffer. This buffer is a universal buffer, which covers a pH range from 2 to 8. Interestingly, this it would allow a relative activity comparison of oxidoreductases in screenings without the need to switch buffer types. Especially, because buffer types are reported to also affect oxidoreductase activity (Zhao, 2005; Kulig et al., 2013). All three targeted oxidoreductases were applied as CCE in 100 mM CPi buffer and 20 mM CPi buffer and compared against 100 mM KPi buffer with 10 mM citrate supplementation.
KRED1-Pglu and RADH CCE exhibited only reduced specific activity in 100 mM CPi and accordingly with 20 mM CPi, when compared to the standard reaction conditions (Figures 8A,C). For LbADH almost no activity loss was observed, when 100 mM CPi was compared against the standard conditions (Figure 8B). Even at 20 mM CPi only a minor decline in activity was determined.
Figure 8. Comparison of citrate-phosphate buffer against citrate addition in terms of specific initial activities of different oxidoreductases. The required cofactor for the NADPH-dependent oxidoreductases is regenerated by either 10 mM citrate supplementation in an environment using 100 mM KPi buffer or directly applying citrate as 20 or 100 mM citrate-phosphate buffer. (A) was performed with KRED1-Pglu at pH 8, (B) was performed with LbADH at pH 6.5, and (C) was performed with RADH at pH 8. 0.34 U mg−1 KRED1-Pglu CCE, 3.56 U mg−1 LbADH CCE, and 2.4 U mg−1 RADH CCE were normalized to 100 % specific activity. n = 3.
This observation could be caused by the pH-dependent buffer composition of the reactions. Both KRED1-Pglu and RADH have their pH optimum for reduction at 8, while LbADH performs best at pH 6.5 (Leuchs and Greiner, 2011; Kulig et al., 2013; Contente et al., 2015). CPi buffer consists of a distinct mixture of a K2HPO4 and a citrate stock solution. The acidic citrate part represent only a minor fraction at a higher pH (McIlvaine, 1921). Hence, 100 mM CPi pH 8 contains 1.2 mM citrate and 20 mM CPi only 0.24 mM. At pH 6 citrate is present in 13 mM concentrations in 100 mM CPi buffer and in the 20 mM setup 2.6 mM concentrations. The results for LbADH indicate this concentration of 2.6 mM in the 20 mM CPi setup as sufficient for a catalytic comparison. This concentration is calculated to also be present in 100 mM CPi buffer pH 7.7 (McIlvaine, 1921). Hence, 100 mM CPi buffer would allow a pH screening under consistent buffer components in a range of pH 2 to 7.7. The clear advantage for screenings is, that effects of changing buffer salts can be neglected (Zhao, 2005; Kulig et al., 2013).
Conclusively, CPi offers the possibility of a universal buffer environment for oxidoredcutase applications in a buffer range of pH 2 to 7.7. This could for instance presently be employed for relative activity comparisons. However, it should be treated cautiously as the applied enzymes in this study exhibit different activities toward the used artificial substrate acetophenone. Thus, the regeneration capacity for NADPH in the present stage may be insufficient for reactions with high Vmax.
In summary, the cheap bulk chemical citrate was proven to regenerate NADPH by conversion through the endogenous TCA cycle in a CCE and LWC setup. CCE setups yielded higher specific activities than LWCs, most likely due to the absence of a diffusion barrier. An analysis of initial activities revealed a limitation of the recombinant oxidoreductases, which was thought to be due to too low NADPH levels to achieve Vmax. Here, endogenous TCA enzyme levels and NADP+ amounts could be excluded as limitation origin as experiments found them to be present in abundance. A transient isotopic labeling experiment feeding [1,5-13C]citrate verified citrate dissipation in the glyoxylate shunt to a minor extent and a supposedly significant loss of NADPH in glutamate synthesis. In the future, the glyoxylate shunt could be inactivated through cultivation of E. coli on glucose as sole carbon source. Glutamate synthesis could be lowered by feeding only defined amounts of ammonia. Apart from the characterization of the citrate regeneration, citrate was also successfully applied as CPi reaction buffer for targeted oxidoreductase reactions. Hence, citrate is proposed as applicable in comparative activity screenings of oxidoreductases in a range of pH 6.5 to 7.7. Advantageously, this allows an inexpensive NADPH regeneration in a simple reaction setup, without the need of extensive additives. This may become even more appealing once necessary genetic optimizations in E. coli have been made. Nonetheless, the described method is already in the present form well applicable to produce satisfying conversions in product synthesis. Notably, this study also uncovered that further steps of the TCA cycle are active, in which also NADH is regenerated. Though this was not further investigated in this study, the application of citrate may even be broadened to NADH-dependent enzymes.
RO and DöR designed the study and prepared the manuscript. The enzyme KRED1-Pglu from Ogataea glucozyma CBD 5766 was contributed by DiR. TN carried out the experimental setup and procedure. RO, TN, and JG carried out the analytics and evaluation of results. RO and DöR supervised the experimental work. RO, JG, DiR, SN, and DöR helped to finalize the manuscript. All authors approved the final manuscript.
This project was funded by the Helmholtz-Association in frame of the young investigators group synthetic enzyme cascades.
The authors declare that the research was conducted in the absence of any commercial or financial relationships that could be construed as a potential conflict of interest.
Many thanks go to Jannick Kappelmann providing us with the isocitrate dehydrogenase form Bacillus subtilis. Further we thank Jennifer Goldmanns for her experimental work on the subject.
The Supplementary Material for this article can be found online at: https://www.frontiersin.org/articles/10.3389/fbioe.2018.00196/full#supplementary-material
CCE, crude cell extract; DMSO, dimethyl-sulfoxide; GDH, glutamate dehydrogenase; GR, glyoxylate reductase; LbADH, Lactobacillus brevis alcohol dehydrogenase; LWC, lyophilized whole cells; IDH, isocitrate dehydrogenase; NADH, β-1, 2-nicotinamide adenine dinucleotide; NADPH, β-1, 2-nicotinamide adenine dinucleotide phosphate; KRED1-Pglu, Ogataea glucozyma CBS 5766 ketoreductase 1; RADH, Ralstonia species alcohol dehydrogenase; TCA, tricarboxylic acid; TIC, total ion chromatogram; XIC, extracted ion chromatogram.
Bennett, B. D., Kimball, E. H., Gao, M., Osterhout, R., Van Dien, S. J., and Rabinowitz, J. D. (2009). Absolute metabolite concentrations and implied enzyme active site occupancy in Escherichia coli. Nat. Chem. Biol. 5, 593–599. doi: 10.1038/nchembio.186
Blank, L. M., Ebert, B. E., Buehler, K., and Bühler, B. (2010). Redox biocatalysis and metabolism: molecular mechanisms and metabolic network analysis. Antioxid. Redox Signal. 13, 349–394. doi: 10.1089/ars.2009.2931
Bornadel, A., Hatti-Kaul, R., Hollmann, F., and Kara, S. (2016). Enhancing the productivity of the bi-enzymatic convergent cascade for ε-caprolactone synthesis through design of experiments and a biphasic system. Tetrahedron 72, 7222–7228. doi: 10.1016/j.tet.2015.11.054
Burton, S. G. (2003). Oxidizing enzymes as biocatalysts. Trends Biotechnol. 21, 543–549. doi: 10.1016/j.tibtech.2003.10.006
Chemler, J. A., Fowler, Z. L., McHugh, K. P., and Koffas, M. A. G. (2010). Improving NADPH availability for natural product biosynthesis in Escherichia coli by metabolic engineering. Metab. Eng. 12, 96–104. doi: 10.1016/j.ymben.2009.07.003
Contente, M. L., Serra, I., Brambilla, M., Eberini, I., Gianazza, E., De Vitis, V., et al. (2015). Stereoselective reduction of aromatic ketones by a new ketoreductase from Pichia glucozyma. Appl. Microbiol. Biotechnol. 100, 193–201. doi: 10.1007/s00253-015-6961-y
Contente, M. L., Serra, I., Palazzolo, L., Parravicini, C., Gianazza, E., Eberini, I., et al. (2016). Enzymatic reduction of acetophenone derivatives with a benzil reductase from Pichia glucozyma (KRED1-Pglu): electronic and steric effects on activity and enantioselectivity. Org. Biomol. Chem. 14, 3404–3408. doi: 10.1039/C6OB00047A
Csonka, L. N., and Fraenkel, D. G. (1976). Pathways of NADPH formation in Escherichia coli. J. Biol. Chem. 252, 3382–3391.
Faber, K. (ed.). (2011). “Introduction and Background Information,” in Biotransformations in Organic Chemistry (Berlin, Heidelberg: Springer), 1–30.
Gerhards, T., Mackfeld, U., Bocola, M., von Lieres, E., Wiechert, W., Pohl, M., et al. (2012). Influence of organic solvents on enzymatic asymmetric carboligations. Adv. Synth. Catal. 354, 2805–2820. doi: 10.1002/adsc.201200284
Goldberg, K., Schroer, K., Lütz, S., and Liese, A. (2007). Biocatalytic ketone reduction—a powerful tool for the production of chiral alcohols—part II: whole-cell reductions. Appl. Microbiol. Biotechnol. 76, 249–255. doi: 10.1007/s00253-007-1005-x
Hall, M., and Bommarius, A. S. (2011). Enantioenriched compounds via enzyme-catalyzed redox reactions. Chem. Rev. 111, 4088–4110. doi: 10.1021/cr200013n
Hummel, W., and Gröger, H. (2014). Strategies for regeneration of nicotinamide coenzymes emphasizing self-sufficient closed-loop recycling systems. J. Biotechnol. 191, 22–31. doi: 10.1016/j.jbiotec.2014.07.449
Kara, S., Schrittwieser, J. H., Hollmann, F., and Ansorge-Schumacher, M. B. (2014). Recent trends and novel concepts in cofactor-dependent biotransformations. Appl. Microbiol. Biotechnol. 98, 1517–1529. doi: 10.1007/s00253-013-5441-5
Klibanov, A. (1997). Why are enzymes less active in organic solvents than in water? Trends Biotechnol. 15, 97–101. doi: 10.1016/S0167-7799(97)01013-5
Kulig, J., Frese, A., Kroutil, W., Pohl, M., and Rother, D. (2013). Biochemical characterization of an alcohol dehydrogenase from Ralstonia sp. Biotechnol. Bioeng. 110, 1838–1848. doi: 10.1002/bit.24857
Leuchs, S., and Greiner, L. (2011). Alcohol dehydrogenase from Lactobacillus brevis: a versatile robust catalyst for enantioselective transformations. Chem. Biochem. Eng. Q. 25, 267–281. Available online at: https://hrcak.srce.hr/69863
Liese, A., and Villela Filho, M. (1999). Production of fine chemicals using biocatalysis. Curr. Opin. Biotechnol. 10, 595–603. doi: 10.1016/S0958-1669(99)00040-3
Marpani, F., Pinelo, M., and Meyer, A. S. (2017). Enzymatic conversion of CO2 to CH3 OH via reverse dehydrogenase cascade biocatalysis: quantitative comparison of efficiencies of immobilized enzyme systems. Biochem. Eng. J. 127, 217–228. doi: 10.1016/j.bej.2017.08.011
Noronha, S. B., Yeh, H. J. C., Spande, T. F., and Shiloach, J. (2000). Investigation of the TCA cycle and the glyoxylate shunt in Escherichia coli BL21 and JM109 using 13C-NMR/MS. Biotechnol. Bioeng. 68, 316–327. doi: 10.1002/(SICI)1097-0290(20000505)68:3<316::AID-BIT10>3.0.CO;2-2
Ornston, L. N., and Ornston, M. K. (1969). Regulation of glyoxylate metabolism in Escherichia coli K-12. J. Bacteriol. 98, 1098–1108.
Paczia, N., Nilgen, A., Lehmann, T., Gätgens, J., Wiechert, W., and Noack, S. (2012). Extensive exometabolome analysis reveals extended overflow metabolism in various microorganisms. Microb. Cell Fact. 11:122. doi: 10.1186/1475-2859-11-122
Phue, J.-N., Noronha, S. B., Hattacharyya, R., Wolfe, A. J., and Shiloach, J. (2005). Glucose metabolism at high density growth of E. coli B and E. coli K: differences in metabolic pathways are responsible for efficient glucose utilization in E. coli B as determined by microarrays and Northern blot analyses. Biotechnol. Bioeng. 90, 805–820. doi: 10.1002/bit.20478
Phue, J. N., and Shiloach, J. (2004). Transcription levels of key metabolic genes are the cause for different glucose utilization pathways in E. coli B (BL21) and E. coli K (JM109). J. Biotechnol. 109, 21–30. doi: 10.1016/j.jbiotec.2003.10.038
Pos, K. M., Dimroth, P., and Bott, M. (1998). The Escherichia coli citrate carrier CitT: a member of a novel eubacterial transporter family related to the 2-oxoglutarate/malate translocator from spinahc chloroplasts. J. Bacteriol. 180, 4160–4165.
Rauter, M., Prokoph, A., Kasprzak, J., Becker, K., Baronian, K., Bode, R., et al. (2015). Coexpression of Lactobacillus brevis ADH with GDH or G6PDH in Arxula adeninivorans for the synthesis of 1-(R)-phenylethanol. Appl. Microbiol. Biotechnol. 99, 4723–4733. doi: 10.1007/s00253-014-6297-z
Schwaneberg, U., Otey, C., Cirino, P. C., Farinas, E., and Arnold, F. H. (2001). Cost-efficient whole-cell assay for laboratory evolution of hydroxylases in Escherichia coli. J. Biomol. Screen. 6, 111–117. doi: 10.1177/108705710100600207
Spaans, S. K., Weusthuis, R. A., van der Oost, J., and Kengen, S. W. M. (2015). NADPH-generating systems in bacteria and archaea. Front. Microbiol. 6, 1–27. doi: 10.3389/fmicb.2015.00742
Straathof, A. J., Panke, S., and Schmid, A. (2002). The production of fine chemicals by biotransformations. Curr. Opin. Biotechnol. 13, 548–556. doi: 10.1016/S0958-1669(02)00360-9
Studier, F. W. (2005). Protein production by auto-induction in high-density shaking cultures. Protein Expr. Purif. 41, 207–234. doi: 10.1016/j.pep.2005.01.016
Tufvesson, P., Lima-Ramos, J., Al Haque, N., Gernaey, K. V., and Woodley, J. M. (2013). Advances in the process development of biocatalytic processes. Org. Process Res. Dev. 17, 1233–1238. doi: 10.1021/op4001675
van der Donk, W. A., and Zhao, H. (2003). Recent developments in pyridine nucleotide regeneration. Curr. Opin. Biotechnol. 14, 421–426. doi: 10.1016/S0958-1669(03)00094-6
Vasquez, B., and Reeves, H. C. (1979). NADP-specific isocitrate dehydrogenase of Escherichia coli. Biochim. Biophys. Acta - Protein Struct. 578, 31–40. doi: 10.1016/0005-2795(79)90109-0
Wichmann, R., and Vasic-Racki, D. (2005). Cofactor regeneration at the lab scale. Adv. Biochem. Eng. Biotechnol. 92, 225–260. doi: 10.1007/b98911
Wu, H., Tian, C., Song, X., Liu, C., Yang, D., and Jiang, Z. (2013). Methods for the regeneration of nicotinamide coenzymes. Green Chem. 15:1773. doi: 10.1039/c3gc37129h
Xu, F. (2005). Applications of oxidoreductases: recent progress. Ind. Biotechnol. 1, 38–50. doi: 10.1089/ind.2005.1.38
Zhao, H. (2005). Effect of ions and other compatible solutes on enzyme activity, and its implication for biocatalysis using ionic liquids. J. Mol. Catal. B Enzym. 37, 16–25. doi: 10.1016/j.molcatb.2005.08.007
Keywords: citrate oxidation, oxidoreductase screening, nicotinamide cofactor, reduction equivalent regeneration, NADPH regeneration, cofactor regeneration
Citation: Oeggl R, Neumann T, Gätgens J, Romano D, Noack S and Rother D (2018) Citrate as Cost-Efficient NADPH Regenerating Agent. Front. Bioeng. Biotechnol. 6:196. doi: 10.3389/fbioe.2018.00196
Received: 29 March 2018; Accepted: 28 November 2018;
Published: 21 December 2018.
Edited by:
Roland Wohlgemuth, Lodz University of Technology, PolandReviewed by:
Rajni Hatti Kaul, Lund University, SwedenCopyright © 2018 Oeggl, Neumann, Gätgens, Romano, Noack and Rother. This is an open-access article distributed under the terms of the Creative Commons Attribution License (CC BY). The use, distribution or reproduction in other forums is permitted, provided the original author(s) and the copyright owner(s) are credited and that the original publication in this journal is cited, in accordance with accepted academic practice. No use, distribution or reproduction is permitted which does not comply with these terms.
*Correspondence: Dörte Rother, ZG8ucm90aGVyQGZ6LWp1ZWxpY2guZGU=
Disclaimer: All claims expressed in this article are solely those of the authors and do not necessarily represent those of their affiliated organizations, or those of the publisher, the editors and the reviewers. Any product that may be evaluated in this article or claim that may be made by its manufacturer is not guaranteed or endorsed by the publisher.
Research integrity at Frontiers
Learn more about the work of our research integrity team to safeguard the quality of each article we publish.