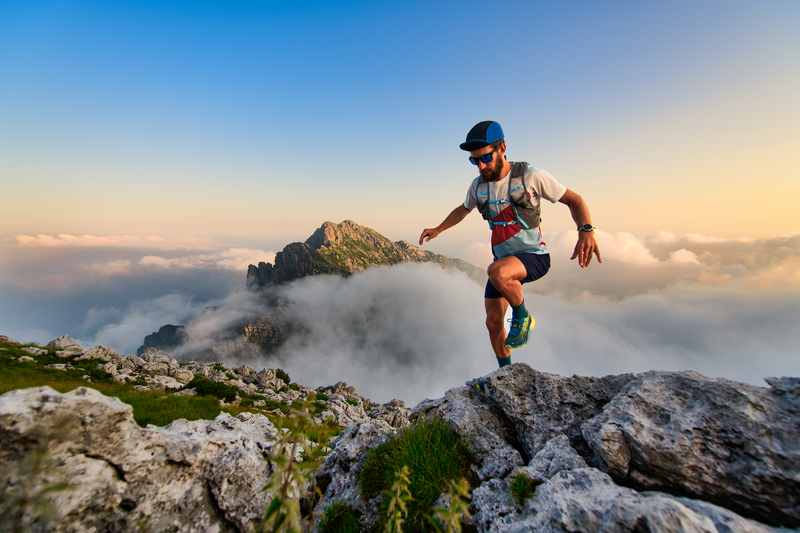
94% of researchers rate our articles as excellent or good
Learn more about the work of our research integrity team to safeguard the quality of each article we publish.
Find out more
MINI REVIEW article
Front. Bioeng. Biotechnol. , 13 July 2018
Sec. Tissue Engineering and Regenerative Medicine
Volume 6 - 2018 | https://doi.org/10.3389/fbioe.2018.00098
The importance of immunity in tissue repair and regeneration is now evident. Thus, promoting tissue healing through immune modulation is a growing and promising field. Targeting microRNAs (miRNAs) is an appealing option since they regulate immunity through post-transcriptional gene fine-tuning in immune cells. Indeed, miRNAs are involved in inflammation as well as in its resolution by controlling immune cell phenotypes and functions. In this review, we first discuss the immunoregulatory role of miRNAs during the restoration of tissue homeostasis after injury, focusing mainly on neutrophils, macrophages and T lymphocytes. As tissue examples, we present the immunoregulatory function of miRNAs during the repair and regeneration of the heart, skeletal muscles, skin and liver. Secondly, we discuss recent technological advances for designing therapeutic strategies which target miRNAs. Specifically, we highlight the possible use of miRNAs and anti-miRNAs for promoting tissue regeneration via modulation of the immune system.
Tissue injury is followed by a cascade of processes leading to the restoration of tissue homeostasis and it is well recognized that the immune system is strongly involved in tissue healing. While numerous regulators are known to coordinate the immune response following injury, microRNAs (miRNAs) have emerged as important actors. Therefore, targeting the immunoregulatory roles of miRNAs could become an attractive option for regenerative therapies. miRNAs are well conserved among species and are involved in a variety of key biological processes by controlling post-transcriptional gene expression through decreasing mRNA stability and/or inhibiting translation. Currently, 1982 human miRNAs precursors are annotated into the miRBase (release 22), each of which are thought to regulate hundreds of target genes (Bartel, 2018; Zhang et al., 2018). Their small size and relatively long half-life (Marzi et al., 2016) make them attractive agents for clinical use. Moreover, a single miRNA can modulate numerous genes, thus having a stronger output than single gene therapy. Here, we first focus on the immunoregulatory role of miRNAs during tissue repair and regeneration. Then, we discuss various delivery systems and the potential targeting of miRNAs in immune cells to promote regeneration.
After injury, immune cells trigger a phase of acute inflammation that represents the first line of defense against pathogens. Moreover, inflammation and the immune response are critical to drive tissue repair and regeneration. Nevertheless, a sustained inflammation often impairs the healing process and its resolution is necessary to restore homeostasis. Various immune cell types are mobilized following tissue injury including neutrophils, monocytes/macrophages and Tcells (Julier et al., 2017; Larouche et al., 2018). Neutrophils are rapidly recruited to the injury site and promote monocyte recruitment which differentiate into macrophages. While pro-inflammatory macrophages (commonly named M1) maintain inflammation and initiate the first steps of tissue healing, anti-inflammatory macrophages (commonly named M2) contribute to resolve inflammation and promote tissue remodeling. Tcells are also important – for instance, pro-inflammatory macrophages are able to stimulate conventional Tcells in a positive-feedback loop, which in turn inhibit tissue repair via inflammatory cytokines or cytotoxic activity. In contrast, regulatory Tcells (Tregs) help maintaining an anti-inflammatory environment.
Because neutrophils, macrophages and Tcells are strongly involved in the tissue healing process, they are interesting targets for regenerative medicine. Activities of these immune cells can be modulated by delivering cells, cytokines and biomaterials. Alternatively, targeting miRNAs provides an interesting way to modulate the immune response following injury, since miRNAs are an endogenous mechanism to fine-tune gene expression. For instance, miR-21, miR-146a and miR-155 have been intensely investigated due to their involvement in Toll-like receptor (TLR) activation and inflammation. The anti-inflammatory miR-21 and miR-146a act in a negative feedback loop with the NF-kB pathway (Hou et al., 2009; Sheedy et al., 2010). By contrast, miR-155 is widely considered as a pro-inflammatory miRNA promoting interferon signaling (O'Connell et al., 2009). Other miRNAs are linked to the regulation of innate (Aalaei-andabili and Rezaei, 2013; He et al., 2014) and adaptive immunity (Baumjohann and Ansel, 2013; Tang et al., 2014; Liang et al., 2015). Moreover, immune cells communicate with each other or with neighboring cells by delivering miRNA-containing exosomes (Fernández-Messina et al., 2015). In the next sections, we discuss the immunoregulatory roles of miRNAs in the context of tissue repair and regeneration, highlighting examples in heart, skeletal muscle, skin, and liver (Figure 1).
Figure 1. Targeting miRNA regulation to promote tissue repair and regeneration: ↑, overexpression; ⊥, inhibition; ↑↓, balanced expression. Blue and yellow cells represent Tcells and macrophages respectively. Refer to the text for the specific immune cell subtypes. miRNAs reported in blue refer to tissue-derived miRNAs affecting the related immune cell functions.
Mammalian adult heart cannot regenerate, due to the loss of cardiomyocytes proliferation capacity seven days after birth (Porrello et al., 2011). Indeed, myocardial infarction and other cardiovascular diseases leading to loss of tissue are frequently followed by fibrosis rather than the generation of new cardiomyocytes. Targeting the immune response after cardiac injury may improve current strategies, since excessive inflammation leads to further damage and infarct expansion. Macrophages are central regulators of cardiac repair (Fernández-Velasco et al., 2014; Fujiu et al., 2014) and affect fibroblast functions, which have a major role in fibrosis through extracellular matrix production (Takeda and Manabe, 2011). miR-155 has been associated with macrophage-induced cardiac hypertrophy (Heymans et al., 2013), inflammation and injury in viral heart disease (Corsten et al., 2012) and diabetic heart (Jia et al., 2017), by enhancing a pro-inflammatory phenotype. Furthermore, miR-155-enriched-exosomes from macrophages suppress fibroblast proliferation and enhance inflammation during cardiac injury (Wang et al., 2017a). Anti-inflammatory miRNAs such as miR-146a have been linked to improvement of cardiac function in sepsis mouse models (Gao et al., 2015). miR-146a reduces the expression of myocardial intercellular adhesion molecule 1 and vascular cell adhesion protein 1, thus decreasing infiltrating macrophages and neutrophils into the heart (Cavaillon and Adib-Conquy, 2005; Alves-Filho et al., 2008). Interestingly, cardiosphere-derived cells have demonstrated to have a role in macrophage polarization through exosome-mediated signaling. Exosomes secreted by cardiospheres delivered after myocardial infarction modulate macrophages toward a cardioprotective phenotype via miR-181b which is, at least partially, responsible for reduced proinflammatory signaling and enhanced phagocytosis (De Couto et al., 2017). The phagocytic activity of anti-inflammatory macrophages (efferocytosis) is likewise important for cardiac repair since it allows clearance of debris and apoptotic cardiomyocytes and neutrophils (Lörchner et al., 2015). In diabetic mouse models where efferocytosis is impaired, miR-126 overexpression rescues efferocytosis (Suresh Babu et al., 2016). This miRNA is also implicated in Treg regulation as it enhances Foxp3 expression, which drives Treg immuno-suppressive functions through interleukin (IL)-10 and transforming growth factor-β (TGF-β) production. While the role of Tcells in cardiac healing is becoming evident (Weirather et al., 2014), little is known about their regulation by miRNA in this context. Yet, in a mouse model of myocarditis, miR-155 drives Th17/Treg imbalance and its inhibition through a synthetic oligonucleotide (antagomir-155) demonstrated to be promising (Yan et al., 2016). Moreover, in patients carrying acute myocardial infarction, the number of circulating Tregs was lower and inversely correlated with miR-21, which seems to negatively regulate Tregs through the Foxp3-TGF-β1 axis (Li et al., 2015c).
Skeletal muscle regeneration is complex, relying on satellite cells (Chang and Rudnicki, 2014) along with immune cells (Bosurgi et al., 2011) and other cell types such as fibroblasts (Murphy et al., 2011). After injury, pro-inflammatory macrophages positively influence satellite cells and myoblast proliferation (Tidball, 2005; Arnold et al., 2007; Perdiguero et al., 2011). Then, anti-inflammatory macrophages induce myoblast differentiation/fusion and collagen production (Arnold et al., 2007). miR-155-deficient mice manifest delayed muscle regeneration, mainly due to unbalances between pro- and anti-inflammatory macrophages (Nie et al., 2016). This balance is also regulated by miR-21 which acts through the p38-miR-21-AKT pathway, which prevents anti-inflammatory polarization (Perdiguero et al., 2011). The importance of early inflammation controlled by miRNAs is confirmed in human where miR-146a expression, known to negatively regulate pro-inflammatory macrophage migration, is lower in polymyositis/dermatomyositis patients compared to normal subjects (Yin et al., 2016). Though many studies focused on macrophages, the role of Tcells in muscle regeneration has gained interest. For example, Burzyn and colleagues have demonstrated that Tregs enhance satellite cells colony-forming capacity and control Tcell infiltration, thus restricting the negative impact of T helper and cytotoxic Tcells on muscle repair. Treg accumulation in muscle coincides with the switch from pro- to anti-inflammatory macrophages (Burzyn et al., 2013) and IL-33 has been reported to be crucial for Tregs accumulation in muscle (Kuswanto et al., 2016). Interestingly, miR-155 appears to regulate IL-33 responsiveness in type 2 innate lymphoid cells (Johansson et al., 2017), but a direct link between miRNA regulation of Tcell functions and muscle regeneration has not yet been established.
Scarring is a common feature of wound healing and the immune system is strongly involved in this process (Larouche et al., 2018). For example, mice lacking pro-inflammatory macrophages during the first/mid-stages of wound healing show impaired repair (Lucas et al., 2010). Nevertheless, polarization of macrophages to an anti-inflammatory phenotype is needed to continue the healing process. miR-146a, miR-132, and miR-21 have been shown to be important for wound healing immune regulation. For instance, miR-132 is up-regulated during the inflammatory-to-proliferative transition phases where it is mostly induced in keratinocytes, promoting their growth and decreasing chemokines production (Li et al., 2015a). Along with miR-146a, miR-132 promotes an anti-inflammatory macrophage polarization (Essandoh et al., 2016) and is highly expressed in skin-infiltrated neutrophils (Larsen et al., 2013). miR-146a contributes to inflammation resolution acting mainly through keratinocytes. This miRNA is up-regulated upon injury via the NF-kB pathway and its long-lasting expression is necessary to down-regulate keratinocytes inflammatory cytokines expression (IL-17, IL-8, and TNF-α) (Meisgen et al., 2014; Srivastava et al., 2017). miR-21 regulates efferocytosis in macrophages, where its expression level increases after apoptotic neutrophil engulfment (Das et al., 2014). miR-21 is found overexpressed in infiltrating T helper cells in skin psoriasis, but in this context, it may contribute to inflammation by supporting Tcell survival (Meisgen et al., 2012). Further evidences regarding Tcell regulation via miRNA during skin healing principally come from two studies. miR-155 and miR-223 have been shown to worsen psoriasis conditions, potentiating Tcell response and Th17-IL-17 production (Løvendorf et al., 2015), while high level of miR-138 could ameliorate skin healing through Th2-IL4 (Fu et al., 2015).
The liver possesses a high regenerative ability and can regenerate after a partial hepatectomy (Michalopoulos, 2017). However, this capacity does not improve the outcome of many liver diseases, particularly those associated with chronic inflammation, leading to fibrosis and cirrhosis (Marcellin and Kutala, 2018). miR-122 is a liver-specific miRNA accounting for more than half of all liver miRNA species. The miRNA is abundant in hepatocytes where involved in hepatic functions and diseases (Otsuka et al., 2017) and intercellular communication through exosomes. High levels of miR-122-enriched exosomes are present in the sera of mice and healthy individuals after alcohol consumption or chronic consumption. miR-122-enriched exosomes are horizontally transferred to monocytes, sensitizing them to lipopolysaccharide and inducing inflammatory reactions (Momen-Heravi et al., 2015). Other miRNAs involved in alcoholic liver disease (ALD) progression through immune cells modulation are miR-181b, miR-155 and miR-223. miR-181b and miR-155 are, respectively, down-regulated and up-regulated in Kupffer cells of ALD mouse models resulting in sensitization of inflammatory pathways through NF-kB signaling (miR181b) (Saikia et al., 2017) and TNF-α mRNA stabilization (miR-155) (Bala et al., 2011). miR-223 acts in neutrophils, by inhibiting the oxidative stress pathway and thus reducing injury exacerbation and fibrosis by reactive oxygen species (Li et al., 2017). Decreasing the pro-inflammatory miR-155 has proven to positively affect non-alcoholic diseases. miR-155 deficiency in ischemia-reperfusion injury mice induces the development of anti-inflammatory macrophages (Tang et al., 2015), while miR-182 and miR-146a overexpression protects the liver by inactivating TLR4 pathway (Jiang et al., 2014, 2016). In non-alcoholic steatohepatitis, TLRs play important roles (Roh and Seki, 2013) and it has been demonstrated that TLR2-enhanced expression of Nod-like receptor protein 3 via NF-kB, promotes NLRP3-inflammasome activation in concert with saturated fatty acid in Kupffer cells (Miura et al., 2013). A study in a mouse model of non-alcoholic steatohepatitis identified decreased miR-144 expression in Kupffer cells with consequent induction of TLR2 (Li et al., 2015b). Regarding the immunoregulatory role of miRNA in Tcells, two studies that used a concanavalin A-treated mice model of liver injury revealed miR-155 and miR15a/16-1 as potential miRNA targets to restore liver homeostasis. Blaya et al. found an altered miR-155 expression in both liver and peripheral blood mononuclear cells, with significant lower Treg recruitment in miR-155−/− mouse (Blaya et al., 2018), while Lu et al. demonstrated that the deletion of miR15a/16-1 in CD4+ cells promotes liver regeneration through IL-22 up-regulation (Lu et al., 2018).
miRNA are emerging as a novel therapeutic approach and numerous miRNAs have reached clinical trials for the treatment of various diseases from cancer (van Zandwijk et al., 2017) to regenerative medicine (Curtin et al., 2018) (Table 1A). Therapeutic miRNA agents include a wide range of miRNA modifications, mostly regarding miRNA inhibition strategies (Table 1B). In addition, many delivery methods have been developed and tested in animal models (Table 1C).
Table 1B. miRNA modifications to achieve miRNA inhibition or upregulation, both in vitro and in vivo.
While direct injections of miRNAs or miRNA inhibitors have been widely used, this simple approach present limitations such as in vivo stability and biodistribution. The development of advanced delivery systems could overcome direct injection limitations (Zhang et al., 2013; Frith et al., 2014). Among delivery systems, exosomes have been explored to some extent, because they are the natural delivery system of miRNA in vivo. They protect miRNAs from degradation during systemic transport and can target specific cell types through membrane ligands. In addition, although they carry MHC-I and/or MHC-II peptides, allogenic exosomes have low immunogenicity. Their cargo composition is tightly regulated, but can change according to specific microenvironment conditions and this may lead to unwanted miRNA species. The main obstacles are the low amount of exosomes secreted by mammalian cells and the complex process of purification, which limit their usage in regenerative medicine. Artificial mimetic exosomes have the potential to overcome these disadvantages and avoid possible immune responses. The challenge is to engineer mimetic exosomes with components of natural exosomes that are still not well defined (Barile and Vassalli, 2017; Bjørge et al., 2018; Kim et al., 2018). Among artificial lipid-based vectors, liposomes are extensively used for in vitro application. Cationic vesicles share the advantages of exosomes and bind miRNA/anti-miRNA molecules via electrostatic interactions. Their composition can be modified to mimic exosomes, but they usually exhibit higher toxicity than their natural counterpart and can activate the complement (Szebeni, 2005; Gori et al., 2015; Peng et al., 2015). For instance, locked nucleic acid (LNA)-based anti-miR-21 and anti-miR-712 have been delivered in mouse models of atherosclerosis and nerve trauma, through liposomes or cationic lipids-coated nanoparticles (NPs), to reduce the inflammatory macrophage number (Kheirolomoom et al., 2015; Simeoli et al., 2017).
In contrast to liposomes, NPs may show less immunogenicity issues. NPs present various advantages such as small size (10–1,000 nm), high surface area, good stability in physiological media and great cellular uptake. Inorganic NPs are made of various solid materials such as gold, silicon, magnesium, silver, and iron (Gori et al., 2015; Ahmadzada et al., 2018). For example, two recent studies have used gold NPs as carriers of miRNAs mimics and antagomirs to promote mouse osteogenic differentiation and osseointegration of implants (Liu et al., 2017b; Yu et al., 2017). Organic NPs are lipid-, proteic-, or polymer- based and have been exploited in clinical studies. Cationic polymer-based NPs made of natural/synthetic polymers have a great binding affinity for miRNA and are frequently complexed with hyaluronic acid (HA), which enhances biocompatibility and gene transfection efficiency. HA-chitosan-based NPs have been used in two studies to promote osteogenesis through in vitro miRNA mimics and in vivo antagomirs transfection, in human and mice mesenchymal stem cells respectively (Wang et al., 2016; Wu et al., 2016). As another example, hyaluronic acid-poly(ethylenimine)-based NPs or polymer complexes have been used in mice to deliver miR-125 and miR-155 into macrophages (Liu et al., 2017a; Parayath et al., 2018).
As an alternative to these delivery systems, scaffold-mediated delivery is particularly interesting in the context of tissue regeneration. The scaffold can be delivered directly in the injured site and can naturally contain or be functionalized with additional pro-regenerative molecules (Peng et al., 2015; Curtin et al., 2018). For instance, a single injection of HA hydrogel-miR-302 mimics complexes in mice hearts after ischemic injury promotes cardiomyocytes proliferation (Wang et al., 2017b). As other examples, a porous collagen-nanohydroxyapatite scaffold containing the antogomir-133a has been used to promote bone regeneration via mesenchymal stem cell-mediated osteogenesis (Menciá Castanõ et al., 2016), and a polymer-based scaffold (polyethylene glycol and polyethylenimine) has been used for miR-26 mimics delivery to promote angiogenesis and osteoclast formation (Zhang et al., 2016).
Targeting miRNA still presents limitations. The complex regulatory network of a single miRNA renders the precise identification of all its target difficult, leading to possible unwanted mRNA silencing. Dosage is also critical—low level of miRNA may be insufficient to achieve the desired outcome, while high level of miRNA is likely to hold the RNA-induced silencing complex (RISC), preventing the action of endogenous miRNAs and leading to off target consequences. This is a problem in common with anti-miRNA therapy, since mature miRNAs are bound to RISC proteins and their inhibition does not allow the release of the complex. Most anti-miRNA molecules are perfectly complementary to the seed region of miRNAs and are chemically modified to increase the melting temperature of the anti-miRNA-miRNA complex. However, the inhibitory interaction under physiological conditions is less strict and anti-miRNAs are frequently unable to distinguish among miRNAs of the same family. An alternative anti-miRNA method could act at precursor stages of miRNA maturation. The longer sequence of primary (>1,000 nt) and precursor (~70 nt) miRNAs contains non-conserved regions that differ even among miRNAs of the same family. Although this strategy may be a viable approach to overcome cross-family miRNA inhibition, further efforts are necessary to develop diverse anti-miRNA species since most of them concentrate on mature miRNAs.
Examples of systems that specifically deliver miRNAs/anti-miRNAs to immune cells are still sparse. The use of ligands, peptides or antibodies with nanotechnology allow targeting specific cells. For instance, lipid nanoparticles coated with a single-chain antibody specific for a dendritic cell receptor enable the release of siRNAs to a subset of dendritic cells (Katakowski et al., 2016). Interesting nanocarriers are for example nucleic acid aptamers, single-stranded DNA or RNA oligonucleotides that bind the target molecule with high affinity. They are remarkably stable, present a very low immunogenicity and allow extensive site-specific chemical modification, offering a wide range of targets such as proteins, nucleic acids, carbohydrates or whole cells (Zhou and Rossi, 2018). For example, an aptamer-siRNA conjugate has been developed to target Tcells, thus releasing anti-HIV siRNAs in HIV-infected mice (Zhou et al., 2013). This approach to engineering miRNA-carriers that target specific immune cells could be used for regenerative medicine applications.
In conclusion, further advances are necessary to better understand the complex regulatory network of miRNAs and to predict the outcome of miRNA replacement or inhibition in vivo. The development of novel and specific miRNA delivery strategies to immune cells could create new opportunities to promote tissue regeneration via immune regulation.
CP, ZJ, and MM wrote the manuscript. CP made the figure and table. MM supervised the writing.
The authors declare that the research was conducted in the absence of any commercial or financial relationships that could be construed as a potential conflict of interest.
This work was supported in part by the Australian Research Council (DE170100398), the National Health and Medical Research Council (APP1140229) to MM and the Swiss National Science Foundation (P2ELP3_175071) to ZJ.
Aalaei-andabili, S. H., and Rezaei, N. (2013). Toll like receptor (TLR)-induced differential expression of microRNAs (MiRs) promotes proper immune response against infections: a systematic review. J. Infect. 67, 251–264. doi: 10.1016/j.jinf.2013.07.016
Ahmadzada, T., Reid, G., and McKenzie, D. R. (2018). Fundamentals of siRNA and miRNA therapeutics and a review of targeted nanoparticle delivery systems in breast cancer. Biophys. Rev. 10, 69–86. doi: 10.1007/s12551-017-0392-1
Alves-Filho, J. C., de Freitas, A., Spiller, F., Souto, F. O., and Cunha, F. Q. (2008). The role of neutrophils in severe sepsis. Shock 30 (Suppl 1), 3–9. doi: 10.1097/SHK.0b013e3181818466
Arnold, L., Henry, A., Poron, F., Baba-Amer, Y., van Rooijen, N., Plonquet, A., et al. (2007). Inflammatory monocytes recruited after skeletal muscle injury switch into antiinflammatory macrophages to support myogenesis. J. Exp. Med. 204, 1057–1069. doi: 10.1084/jem.20070075
Bala, S., Marcos, M., Kodys, K., Csak, T., Catalano, D., Mandrekar, P., et al. (2011). Up-regulation of microRNA-155 in macrophages contributes to increased Tumor Necrosis Factor α (TNFα) production via increased mRNA half-life in alcoholic liver disease. J. Biol. Chem. 286, 1436–1444. doi: 10.1074/jbc.M110.145870
Barile, L., and Vassalli, G. (2017). Exosomes: therapy delivery tools and biomarkers of diseases. Pharmacol. Ther. 174, 63–78. doi: 10.1016/j.pharmthera.2017.02.020
Baumjohann, D., and Ansel, K. M. (2013). MicroRNA regulation of T helper cell differentiation and plasticity. Nat. Rev. Immunol. 13, 666–678. doi: 10.1038/nri3494.MicroRNA
Bjørge, I. M., Kim, S. Y., Mano, J. F., Kalionis, B., and Chrzanowski, W. (2018). Extracellular vesicles, exosomes and shedding vesicles in regenerative medicine – a new paradigm for tissue repair. Biomater. Sci. 6, 60–78. doi: 10.1039/C7BM00479F
Blaya, D., Aguilar-Bravo, B., Hao, F., Casacuberta-Serra, S., Coll, M., Perea, L., et al. (2018). Expression of microRNA-155 in inflammatory cells modulates liver injury. Hepatology doi: 10.1002/hep.29833 [Epub ahead of print]
Bosurgi, L., Manfredi, A. A., and Rovere-Querini, P. (2011). Macrophages in injured skeletal muscle: a perpetuum mobile causing and limiting fibrosis, prompting or restricting resolution and regeneration. Front. Immunol. 2:62. doi: 10.3389/fimmu.2011.00062
Burzyn, D., Kuswanto, W., Kolodin, D., Shadrach, J. L., Cerletti, M., Jang, Y., et al. (2013). A special population of regulatory T cells potentiates muscle repair. Cell 155, 1282–1295. doi: 10.1016/j.cell.2013.10.054
Cavaillon, J.-M., and Adib-Conquy, M. (2005). Monocytes/macrophages and sepsis. Crit. Care Med. 33, S506–S509. doi: 10.1097/01.CCM.0000185502.21012.37
Chang, N. C., and Rudnicki, M. A. (2014). Satellite cells: the architects of skeletal muscle. Curr. Top. Dev. Biol. 107, 161–181. doi: 10.1016/B978-0-12-416022-4.00006-8
Corsten, M. F., Papageorgiou, A., Verhesen, W., Carai, P., Lindow, M., Obad, S., et al. (2012). MicroRNA profiling identifies microRNA-155 as an adverse mediator of cardiac injury and dysfunction during acute viral myocarditis. Circ. Res. 111, 415–425. doi: 10.1161/CIRCRESAHA.112.267443
Curtin, C. M., Castaño, I. M., and O'Brien, F. J. (2018). Scaffold-based microRNA therapies in regenerative medicine and cancer. Adv. Healthc. Mater. 7, 1–22. doi: 10.1002/adhm.201700695
Das, A., Ganesh, K., Khanna, S., Sen, C. K., and Roy, S. (2014). Engulfment of apoptotic cells by macrophages: a role of microRNA-21 in the resolution of wound inflammation. J. Immunol. 192, 1120–1129. doi: 10.4049/jimmunol.1300613
De Couto, G., Gallet, R., Cambier, L., Jaghatspanyan, E., Makkar, N., Dawkins, J. F., et al. (2017). Exosomal microRNA transfer into macrophages mediates cellular postconditioning. 136, 200–214. doi: 10.1161/CIRCULATIONAHA.116.024590
Ebert, M. S., and Sharp, P. A. (2010). MicroRNA sponges: progress and possibilities. RNA 16, 2043–2050. doi: 10.1261/rna.2414110
Eding, J. E., Demkes, C. J., Lynch, J. M., Seto, A. G., Montgomery, R. L., Semus, H. M., et al. (2017). The efficacy of cardiac anti-miR-208a therapy is stress dependent. Mol. Ther. 25, 694–704. doi: 10.1016/j.ymthe.2017.01.012
Essandoh, K., Li, Y, Huo, J., and Fan, G. C. (2016). MiRNA-mediated macrophage polarization and its potential role in the regulation of inflammatory response. Shock 46, 122–131. doi: 10.1097/SHK.0000000000000604
Esau, C. C. (2008). Inhibition of microRNA with antisense oligonucleotides. Methods 44, 55–60. doi: 10.1016/j.ymeth.2007.11.001
Fernández-Messina, L., Gutiérrez-Vázquez, C., Rivas-García, E., Sánchez-Madrid, F., and de la Fuente, H. (2015). Immunomodulatory role of microRNAs transferred by extracellular vesicles. Biol. Cell 107, 61–77. doi: 10.1111/boc.201400081
Fernandez-Piñeiro, I., Badiola, I., and Sanchez, A. (2017). Nanocarriers for microRNA delivery in cancer medicine. Biotechnol. Adv. 35, 350–360. doi: 10.1016/j.biotechadv.2017.03.002
Fernández-Velasco, M., González-Ramos, S., and Boscá, L. (2014). Involvement of monocytes/macrophages as key factors in the development and progression of cardiovascular diseases. Biochem. J. 458, 187–193. doi: 10.1042/BJ20131501
Frith, J. E., Porrello, E. R., and Cooper-White, J. J. (2014). Concise review: new frontiers in MicroRNA-based tissue regeneration. Stem Cells Transl. Med. 3, 969–976. doi: 10.5966/sctm.2014-0032
Fu, D., Yu, W., Li, M., Wang, H., Liu, D., Song, X., et al. (2015). MicroRNA-138 regulates the balance of Th1/Th2 via targeting RUNX3 in psoriasis. Immunol. Lett. 166, 55–62. doi: 10.1016/j.imlet.2015.05.014
Fu, P. P., Xia, Q., Hwang, H.-M., Ray, P. C., and Yu, H. (2014). Mechanisms of nanotoxicity: generation of reactive oxygen species. J. Food Drug Anal. 22, 64–75. doi: 10.1016/j.jfda.2014.01.005
Fujiu, K., Wang, J., and Nagai, R. (2014). Cardioprotective function of cardiac macrophages. Cardiovasc. Res. 102, 232–239. doi: 10.1093/cvr/cvu059
Gao, M., Wang, X., Zhang, X., Ha, T., Ma, H., Liu, L., et al. (2015). Attenuation of cardiac dysfunction in polymicrobial sepsis by MicroRNA-146a is mediated via targeting of IRAK1 and TRAF6 expression. J. Immunol. 195, 672–682. doi: 10.4049/jimmunol.1403155
Gori, M., Trombetta, M., Santini, D., and Rainer, A. (2015). Tissue engineering and microRNAs: future perspectives in regenerative medicine. Expert Opin. Biol. Ther. 15, 1601–1622. doi: 10.1517/14712598.2015.1071349
He, X., Jing, Z., and Cheng, G. (2014). MicroRNAs: new regulators of Toll-like receptor signaling pathways. Biomed Res. Int. 2014:945169. doi: 10.1155/2014/945169
Heymans, S., Corsten, M. F., Verhesen, W., Carai, P., Van Leeuwen, R. E., Custers, K., et al. (2013). Macrophage MicroRNA-155 promotes cardiac hypertrophy and failure. Circulation 128, 1420–1432. doi: 10.1161/CIRCULATIONAHA.112.001357
Hou, J., Wang, P., Lin, L., Liu, X., Ma, F., An, H., et al. (2009). MicroRNA-146a Feedback Inhibits RIG-I-dependent type I IFN production in macrophages by targeting TRAF6, IRAK1, and IRAK2. J. Immunol. 183, 2150–2158. doi: 10.4049/jimmunol.0900707
Hullinger, T. G., Montgomery, R. L., Seto, A. G., Dickinson, B. A., Semus, H. M., Lynch, J. M., et al. (2012). Inhibition of miR-15 protects against cardiac ischemic injurynovelty and significance. Circ. Res. 110, 71–81. doi: 10.1161/CIRCRESAHA.111.244442
Jia, H., Chen, H., Wei, M., Chen, X., Zhang, Y., Cao, L., et al. (2017). Gold nanoparticle-based miR155 antagonist macrophage delivery restores the cardiac function in ovariectomized diabetic mouse model. Int. J. Nanomed. 12, 4963–4979. doi: 10.2147/IJN.S138400
Jiang, W., Kong, L., Ni, Q., Lu, Y., Ding, W., Liu, G., et al. (2014). miR-146a ameliorates liver ischemia/reperfusion injury by suppressing IRAK1 and TRAF6. PLoS ONE 9:e101530. doi: 10.1371/journal.pone.0101530
Jiang, W., Liu, G., and Tang, W. (2016). microrna-182-5p ameliorates liver ischemia-reperfusion injury by suppressing toll-like receptor 4. Transplant. Proc. 48, 2809–2814. doi: 10.1016/j.transproceed.2016.06.043
Johansson, K., Malmhäll, C., Ramos-Ramírez, P., and Rådinger, M. (2017). MicroRNA-155 is a critical regulator of type 2 innate lymphoid cells and IL-33 signaling in experimental models of allergic airway inflammation. J. Allergy Clin. Immunol. 139, 1007–1016.e9. doi: 10.1016/j.jaci.2016.06.035
Julier, Z., Park, A. J., Briquez, P. S., and Martino, M. M. (2017). Promoting tissue regeneration by modulating the immune system. Acta Biomater. 53, 13–28. doi: 10.1016/j.actbio.2017.01.056
Katakowski, J. A., Mukherjee, G., Wilner, S. E., Maier, K. E., Harrison, M. T., DiLorenzo, T. P., et al. (2016). Delivery of siRNAs to dendritic cells using DEC205-targeted lipid nanoparticles to inhibit immune responses. Mol. Ther. 24, 146–155. doi: 10.1038/mt.2015.175
Kheirolomoom, A., Kim, C. W., Seo, J. W., Kumar, S., Son, D. J., Gagnon, M. K., et al. (2015). Multifunctional nanoparticles facilitate molecular targeting and mirna delivery to inhibit atherosclerosis in apoe−/− mice. ACS Nano. 9, 8885–8897. doi: 10.1021/acsnano.5b02611
Kim, Y. S., Ahn, J. S., Kim, S., Kim, H. J., Kim, S. H., and Kang, J. S. (2018). The potential theragnostic (diagnostic+therapeutic) application of exosomes in diverse biomedical fields. Korean J. Physiol. Pharmacol. 22, 113–125. doi: 10.4196/kjpp.2018.22.2.113
Koval, E. D., Shaner, C., Zhang, P., du Maine, X., Fischer, K., Tay, J., et al. (2013). Method for widespread microRNA-155 inhibition prolongs survival in ALS-model mice. Hum. Mol. Genet. 22, 4127–4135. doi: 10.1093/hmg/ddt261
Krützfeldt, J., Kuwajima, S., Braich, R., Rajeev, K. G., Pena, J., Tuschl, T., et al. (2007). Specificity, duplex degradation and subcellular localization of antagomirs. Nucleic Acids Res. 35, 2885–2892. doi: 10.1093/nar/gkm024
Krützfeldt, J., Rajewsky, N., Braich, R., Rajeev, K. G., Tuschl, T., Manoharan, M., et al. (2005). Silencing of microRNAs in vivo with ‘antagomirs.’ Nature 438, 685–689. doi: 10.1038/nature04303
Kuswanto, W., Burzyn, D., Panduro, M., Wang, K. K., Jang, Y. C., Wagers, A. J., et al. (2016). Poor repair of skeletal muscle in aging mice reflects a defect in local, interleukin-33-dependent accumulation of regulatory T cells. Immunity 44, 355–367. doi: 10.1016/j.immuni.2016.01.009
Larouche, J., Sheoran, S., Maruyama, K., and Martino, M. M. (2018). Immune regulation of skin wound healing: mechanisms and novel therapeutic targets. Adv. Wound Care Wound 2017:761. doi: 10.1089/wound.2017.0761
Larsen, M. T., Hother, C., Häger, M., Pedersen, C. C., Theilgaard-Mönch, K., Borregaard, N., et al. (2013). MicroRNA profiling in human neutrophils during bone marrow granulopoiesis and in vivo exudation. PLoS One 8:e58454. doi: 10.1371/journal.pone.0058454
Lennox, K. A., Owczarzy, R., Thomas, D. M., Walder, J. A., and Behlke, M. A. (2013). Improved performance of anti-miRNA oligonucleotides using a novel non-nucleotide modifier. Mol. Ther. Nucleic Acids 2:e117. doi: 10.1038/mtna.2013.46
Li, D., Wang, A., Liu, X., Meisgen, F., Grünler, J., Botusan, I. R., et al. (2015a). MicroRNA-132 enhances transition from inflammation to proliferation during wound healing. J. Clin. Invest. 125, 3008–3026. doi: 10.1172/JCI79052
Li, D., Wang, X., Lan, X., Li, Y., Liu, L., Yi, J., et al. (2015b). Down-regulation of miR-144 elicits proinflammatory cytokine production by targeting toll-like receptor 2 in nonalcoholic steatohepatitis of high-fat-diet-induced metabolic syndrome E3 rats. Mol. Cell. Endocrinol. 402, 1–12. doi: 10.1016/j.mce.2014.12.007
Li, M., He, Y., Zhou, Z., Ramirez, T., Gao, Y., Gao, Y., et al. (2017). MicroRNA-223 ameliorates alcoholic liver injury by inhibiting the IL-6-p47phox-oxidative stress pathway in neutrophils. Gut 66, 705–715. doi: 10.1136/gutjnl-2016-311861
Li, S., Fan, Q., He, S., Tang, T., Liao, Y., and Xie, J. (2015c). MicroRNA-21 negatively regulates treg cells through a TGF-β1/smad-independent pathway in patients with coronary heart disease. Cell. Physiol. Biochem. 37, 866–878. doi: 10.1159/000430214
Liang, Y., Pan, H.-F., and Ye, D.-Q. (2015). microRNAs function in CD8+T cell biology. J. Leukoc. Biol. 97, 487–497. doi: 10.1189/jlb.1RU0814-369R
Liu, L., Yi, H., He, H., Pan, H., Cai, L., and Ma, Y. (2017a). Tumor associated macrophage-targeted microRNA delivery with dual-responsive polypeptide nanovectors for anti-cancer therapy. Biomaterials 134, 166–179. doi: 10.1016/j.biomaterials.2017.04.043
Liu, X., Tan, N., Zhou, Y., Wei, H., Ren, S., Yu, F., et al. (2017b). Delivery of antagomiR204-conjugated gold nanoparticles from PLGA sheets and its implication in promoting osseointegration of titanium implant in type 2 diabetes mellitus. Int. J. Nanomed. 12, 7089–7101. doi: 10.2147/IJN.S124584
Lörchner, H., Pöling, J., Gajawada, P., Hou, Y., Polyakova, V., Kostin, S., et al. (2015). Myocardial healing requires Reg3β-dependent accumulation of macrophages in the ischemic heart. Nat. Med. 21, 353–362. doi: 10.1038/nm.3816
Løvendorf, M. B., Mitsui, H., Zibert, J. R., Røpke, M. A., Hafner, M., Dyring-Andersen, B., et al. (2015). Laser capture microdissection followed by next-generation sequencing identifies disease-related microRNAs in psoriatic skin that reflect systemic microRNA changes in psoriasis. Exp. Dermatol. 24, 187–193. doi: 10.1111/exd.12604
Lu, Z., Liu, J., Liu, X., Huang, E., Yang, J., Qian, J., et al. (2018). MicroRNA 15a/16-1 suppresses aryl hydrocarbon receptor-dependent interleukin-22 secretion in CD4+T cells and contributes to immune-mediated organ injury. Hepatology 67, 1027–1040. doi: 10.1002/hep.29573
Lucas, T., Waisman, A., Ranjan, R., Roes, J., Krieg, T., Müller, W., et al. (2010). Differential roles of macrophages in diverse phases of skin repair. J. Immunol. 184, 3964–3977. doi: 10.4049/jimmunol.0903356
Marcellin, P., and Kutala, B. K. (2018). Liver diseases: a major, neglected global public health problem requiring urgent actions and large-scale screening. Liver Int. 38 (Suppl 1), 2–6. doi: 10.1111/liv.13682
Marzi, M. J., Ghini, F., Cerruti, B., de Pretis, S., Bonetti, P., Giacomelli, C., et al. (2016). Degradation dynamics of microRNAs revealed by a novel pulse-chase approach. Genome Res. 26, 554–565. doi: 10.1101/gr.198788.115
Meisgen, F., Xu Landén, N., Wang, A., Réthi, B., Bouez, C., Zuccolo, M., et al. (2014). MiR-146a negatively regulates TLR2-induced inflammatory responses in keratinocytes. J. Invest. Dermatol. 134, 1931–1940. doi: 10.1038/jid.2014.89
Meisgen, F., Xu, N., Wei, T., Janson, P. C., Obad, S., Broom, O., et al. (2012). MiR-21 is up-regulated in psoriasis and suppresses T cell apoptosis. Exp. Dermatol. 21, 312–314. doi: 10.1111/j.1600-0625.2012.01462.x
Menciá Castanõ, I., Curtin, C. M., Duffy, G. P., and O'Brien, F. J. (2016). Next generation bone tissue engineering: Non-viral miR-133a inhibition using collagen-nanohydroxyapatite scaffolds rapidly enhances osteogenesis. Sci. Rep. 6:27941. doi: 10.1038/srep27941
Michalopoulos, G. K. (2017). Hepatostat: liver regeneration and normal liver tissue maintenance. Hepatology 65, 1384–1392. doi: 10.1002/hep.28988
Miura, K., Yang, L., van Rooijen, N., Brenner, D. A., Ohnishi, H., and Seki, E. (2013). Toll-like receptor 2 and palmitic acid cooperatively contribute to the development of nonalcoholic steatohepatitis through inflammasome activation in mice. Hepatology 57, 577–589. doi: 10.1002/hep.26081
Momen-Heravi, F., Bala, S., Kodys, K., and Szabo, G. (2015). Exosomes derived from alcohol-treated hepatocytes horizontally transfer liver specific miRNA-122 and sensitize monocytes to LPS. Sci. Rep. 5:9991. doi: 10.1038/srep09991
Montgomery, R. L., Hullinger, T. G., Semus, H. M., Dickinson, B. A., Seto, A. G., Lynch, J. M., et al. (2011). Therapeutic inhibition of miR-208a improves cardiac function and survival during heart failure. Circulation 124, 1537–1547. doi: 10.1161/CIRCULATIONAHA.111.030932
Mook, O. R., Vreijling, J., Wengel, S., Wengel, J., Zhou, C., Chattopadhyaya, J., et al. (2010). In vivo efficacy and off-target effects of Locked Nucleic Acid (LNA) and Unlocked Nucleic Acid (UNA) modified siRNA and small internally segmented interfering RNA (sisiRNA) in mice bearing human tumor xenografts. Artif. DNA PNA XNA 1, 36–44. doi: 10.4161/adna.1.1.12204
Murphy, M. M., Lawson, J. A., Mathew, S. J., Hutcheson, D. A., and Kardon, G. (2011). Satellite cells, connective tissue fibroblasts and their interactions are crucial for muscle regeneration. Development 138, 3625–3637. doi: 10.1242/dev.064162
Nie, M., Liu, J., Yang, Q., Seok, H. Y., Hu, X., Deng, Z. L., et al. (2016). MicroRNA-155 facilitates skeletal muscle regeneration by balancing pro- and anti-inflammatory macrophages. Cell Death Dis. 7:e2261. doi: 10.1038/cddis.2016.165
O'Connell, R. M., Chaudhuri, A. A., Rao, D. S., and Baltimore, D. (2009). Inositol phosphatase SHIP1 is a primary target of miR-155. Proc. Natl. Acad. Sci. U.S. A. 106, 7113–7118. doi: 10.1073/pnas.0902636106
Obad, S., dos Santos, C. O., Petri, A., Heidenblad, M., Broom, O., Ruse, C., et al. (2011). Silencing of microRNA families by seed-targeting tiny LNAs. Nat. Genet. 43, 371–378. doi: 10.1038/ng.786
Oh, S. Y., Ju, Y., and Park, H. (2009). A highly effective and long-lasting inhibition of miRNAs with PNA-based antisense oligonucleotides. Mol. Cells 28, 341–345. doi: 10.1007/s10059-009-0134-8
Otsuka, M., Kishikawa, T., Yoshikawa, T., Yamagami, M., Ohno, M., Takata, A., et al. (2017). MicroRNAs and liver disease. J. Hum. Genet. 62, 75–80. doi: 10.1038/jhg.2016.53
Parayath, N. N., Parikh, A., and Amiji, M. M. (2018). Repolarization of tumor-associated macrophages in a genetically engineered nonsmall cell lung cancer model by intraperitoneal administration of hyaluronic acid-based nanoparticles encapsulating microRNA-125b. Nano Lett. ACS.Nanolett. 18, 3571–3579. doi: 10.1021/acs.nanolett.8b00689
Peng, B., Chen, Y., and Leong, K. W. (2015). MicroRNA delivery for regenerative medicine. Adv. Drug Deliv. Rev. 88, 108–122. doi: 10.1016/j.addr.2015.05.014
Perdiguero, E., Sousa-Victor, P., Ruiz-Bonilla, V., Jard,í, M., Caelles, C., Serrano, A. L., et al. (2011). p38/MKP-1-regulated AKT coordinates macrophage transitions and resolution of inflammation during tissue repair. J. Cell Biol. 195, 307–322. doi: 10.1083/jcb.201104053
Porrello, E. R., Mahmoud, A. I., Simpson, E., Hill, J. A., James, A., Olson, E. N., et al. (2011). Transient regeneration potential of the neonatal mouse heart. Science 331, 1078–1080. doi: 10.1126/science.1200708.Transient
Rebustini, I. T., Vlahos, M., Packer, T., Kukuruzinska, M. A., and Maas, R. L. (2016). An integrated miRNA functional screening and target validation method for organ morphogenesis. Sci. Rep. 6:23215. doi: 10.1038/srep23215
Roh, Y. S., and Seki, E. (2013). Toll-like receptors in alcoholic liver disease, non-alcoholic steatohepatitis and carcinogenesis. J. Gastroenterol. Hepatol. 28 (Suppl 1), 38–42. doi: 10.1111/jgh.12019
Saikia, P., Bellos, D., McMullen, M. R., Pollard, K. A., de la Motte, C., and Nagy, L. E. (2017). MicroRNA 181b-3p and its target importin α5 regulate toll-like receptor 4 signaling in Kupffer cells and liver injury in mice in response to ethanol. Hepatology 66, 602–615. doi: 10.1002/hep.29144
Sheedy, F. J., Palsson-McDermott, E., Hennessy, E. J., Martin, C., O'Leary, J. J., Ruan, Q., et al. (2010). Negative regulation of TLR4 via targeting of the proinflammatory tumor suppressor PDCD4 by the microRNA miR-21. Nat. Immunol. 11, 141–147. doi: 10.1038/ni.1828
Sherman, L. S., Condé-Green, A., Sandiford, O. A., and Rameshwar, P. (2015). A discussion on adult mesenchymal stem cells for drug delivery: pros and cons. Ther. Deliv. 6, 1335–1346. doi: 10.4155/tde.15.80
Simeoli, R., Montague, K., Jones, H. R., Castaldi, L., Chambers, D., Kelleher, J. H., et al. (2017). Exosomal cargo including microRNA regulates sensory neuron to macrophage communication after nerve trauma. Nat. Commun. 8:1778. doi: 10.1038/s41467-017-01841-5
Srivastava, A., Nikamo, P., Lohcharoenkal, W., Li, D., Meisgen, F., Xu Landén, N., et al. (2017). MicroRNA-146a suppresses IL-17–mediated skin inflammation and is genetically associated with psoriasis. J. Allergy Clin. Immunol. 139, 550–561. doi: 10.1016/j.jaci.2016.07.025
Suresh Babu, S., Thandavarayan, R. A., Joladarashi, D., Jeyabal, P., Krishnamurthy, S., Bhimaraj, A., et al. (2016). MicroRNA-126 overexpression rescues diabetes-induced impairment in efferocytosis of apoptotic cardiomyocytes. Sci. Rep. 6:36207. doi: 10.1038/srep36207
Szebeni, J. (2005). Complement activation-related pseudoallergy: a new class of drug-induced acute immune toxicity. Toxicology 216, 106–121. doi: 10.1016/j.tox.2005.07.023
Takeda, N., and Manabe, I. (2011). Cellular interplay between cardiomyocytes and nonmyocytes in cardiac remodeling. Int. J. Inflam. 2011:535241. doi: 10.4061/2011/535241
Tang, B., Wang, Z., Qi, G., Yuan, S., Yu, S., Li, B., et al. (2015). MicroRNA-155 deficiency attenuates ischemia-reperfusion injury after liver transplantation in mice. Transpl. Int. 28, 751–760. doi: 10.1111/tri.12528
Tang, X., Tang, R., Xu, Y., Wang, Q., Hou, Y., Shen, S., et al. (2014). MicroRNA networks in regulatory T cells. J. Physiol. Biochem. 70, 869–875. doi: 10.1007/s13105-014-0348-x
Tay, F. C., Lim, J. K., Zhu, H., Hin, L. C., and Wang, S. (2015). Using artificial microRNA sponges to achieve microRNA loss-of-function in cancer cells. Adv. Drug Deliv. Rev. 81, 117–127. doi: 10.1016/j.addr.2014.05.010
Tidball, J. G. (2005). Inflammatory processes in muscle injury and repair. Am. J. Physiol. Regul. Integr. Comp. Physiol. 288, R345–R353. doi: 10.1152/ajpregu.00454.2004
van Zandwijk, N., Pavlakis, N., Kao, S. C., Linton, A., Boyer, M. J., Clarke, S., et al. (2017). Safety and activity of microRNA-loaded minicells in patients with recurrent malignant pleural mesothelioma: a first-in-man, phase 1, open-label, dose-escalation study. Lancet Oncol. 18, 1386–1396. doi: 10.1016/S1470-2045(17)30621-6
Wang, C., Zhang, C., Liu, L., Xi, A., Chen, B., Li, Y., et al. (2017a). Macrophage-derived mir-155-containing exosomes suppress fibroblast proliferation and promote fibroblast inflammation during cardiac injury. Mol. Ther. 25, 192–204. doi: 10.1016/j.ymthe.2016.09.001
Wang, L. L., Liu, Y., Chung, J. J., Wang, T., Gaffey, A. C., Lu, M., et al. (2017b). Sustained miRNA delivery from an injectable hydrogel promotes cardiomyocyte proliferation and functional regeneration after ischaemic injury. Nat. Biomed. Eng. 1, 983–992. doi: 10.1038/s41551-017-0157-y
Wang, Z. (2009). “miRNA Mimic Technology,” in MicroRNA Interference Technologies, ed Z. Wang (Berlin; Heidelberg: Springer), 93–100.
Wang, Z., Wu, G., Wei, M., Liu, Q., Zhou, J., Qin, T., et al. (2016). Improving the osteogenesis of human bone marrow mesenchymal stem cell sheets by microRNA-21-loaded chitosan/hyaluronic acid nanoparticles via reverse transfection. Int. J. Nanomed. 11, 2091–2105. doi: 10.2147/IJN.S104851
Warren, T. K., Shurtleff, A. C., and Bavari, S. (2012). Advanced morpholino oligomers: a novel approach to antiviral therapy. Antiviral Res. 94, 80–88. doi: 10.1016/j.antiviral.2012.02.004
Weirather, J., Hofmann, U. D. W., Beyersdorf, N., Ramos, G. C., Vogel, B., Frey, A., et al. (2014). Foxp3+ CD4+ T cells improve healing after myocardial infarction by modulating monocyte/macrophage differentiation. Circ. Res. 115, 55–67. doi: 10.1161/CIRCRESAHA.115.303895
Wu, G., Feng, C., Hui, G., Wang, Z., Tan, J., Luo, L., et al. (2016). Improving the osteogenesis of rat mesenchymal stem cells by chitosan-based-microRNA nanoparticles. Carbohydr. Polym. 138, 49–58. doi: 10.1016/j.carbpol.2015.11.044
Yan, L., Hu, F., Yan, X., Wei, Y., Ma, W., Wang, Y., et al. (2016). Inhibition of microRNA-155 ameliorates experimental autoimmune myocarditis by modulating Th17/Treg immune response. J. Mol. Med. 94, 1063–1079. doi: 10.1007/s00109-016-1414-3
Yang, N. (2015). An overview of viral and nonviral delivery systems for microRNA. Int. J. Pharm. Investig. 5, 179–181. doi: 10.4103/2230-973X.167646
Yin, Y., Li, F., Shi, J., Li, S., Cai, J., and Jiang, Y. (2016). MiR-146a regulates inflammatory infiltration by macrophages in polymyositis/dermatomyositis by targeting TRAF6 and affecting IL-17/ICAM-1 pathway. Cell. Physiol. Biochem. 40, 486–498. doi: 10.1159/000452563
Yu, M., Lei, B., Gao, C., Yan, J., and Ma, P. X. (2017). Optimizing surface-engineered ultra-small gold nanoparticles for highly efficient miRNA delivery to enhance osteogenic differentiation of bone mesenchymal stromal cells. Nano Res. 10, 49–63. doi: 10.1007/s12274-016-1265-9
Zhang, X., Li, Y., Chen, Y. E., Chen, J., and Ma, P. X. (2016). Cell-free 3D scaffold with two-stage delivery of miRNA-26a to regenerate critical-sized bone defects. Nat. Commun. 7:10376. doi: 10.1038/ncomms10376
Zhang, Y., Wang, Z., and Gemeinhart, R. A. (2013). Progress in microRNA delivery. J. Control. Release 172, 962–974. doi: 10.1016/j.jconrel.2013.09.015
Zhang, Y., Yun, Z., Gong, L., Qu, H., Duan, X., Jiang, Y., et al. (2018). Comparison of miRNA evolution and function in plants and animals. MicroRNA. 7, 4–10. doi: 10.2174/2211536607666180126163031.
Zhou, J., Neff, C. P., Swiderski, P., Li, H., Smith, D. D., Aboellail, T., et al. (2013). Functional in vivo delivery of multiplexed anti-HIV-1 siRNAs via a chemically synthesized aptamer with a sticky bridge. Mol. Ther. 21, 192–200. doi: 10.1038/mt.2012.226
Keywords: microRNAs, regeneration, inflammation, immune system, biomaterials, neutrophils, macrophages, Tcells
Citation: Piotto C, Julier Z and Martino MM (2018) Immune Regulation of Tissue Repair and Regeneration via miRNAs—New Therapeutic Target. Front. Bioeng. Biotechnol. 6:98. doi: 10.3389/fbioe.2018.00098
Received: 30 May 2018; Accepted: 26 June 2018;
Published: 13 July 2018.
Edited by:
Martijn van Griensven, Technische Universität München, GermanyReviewed by:
Carolina Balbi, Cardiocentro Ticino, SwitzerlandCopyright © 2018 Piotto, Julier and Martino. This is an open-access article distributed under the terms of the Creative Commons Attribution License (CC BY). The use, distribution or reproduction in other forums is permitted, provided the original author(s) and the copyright owner(s) are credited and that the original publication in this journal is cited, in accordance with accepted academic practice. No use, distribution or reproduction is permitted which does not comply with these terms.
*Correspondence: Mikaël M. Martino, bWlrYWVsLm1hcnRpbm9AbW9uYXNoLmVkdQ==
Disclaimer: All claims expressed in this article are solely those of the authors and do not necessarily represent those of their affiliated organizations, or those of the publisher, the editors and the reviewers. Any product that may be evaluated in this article or claim that may be made by its manufacturer is not guaranteed or endorsed by the publisher.
Research integrity at Frontiers
Learn more about the work of our research integrity team to safeguard the quality of each article we publish.