- 1College of Pharmacy, Gachon University, Incheon, South Korea
- 2Lee Gil Ya Cancer and Diabetes Institute, Gachon University, Incheon, South Korea
- 3Gachon University Gil Medical Center, Gachon Medical and Convergence Institute, Incheon, South Korea
Loss of skeletal muscle mass and strength has recently become a hot research topic with the extension of life span and an increasingly sedentary lifestyle in modern society. Maintenance of skeletal muscle mass is considered an essential determinant of muscle strength and function. Myokines are cytokines synthesized and released by myocytes during muscular contractions. They are implicated in autocrine regulation of metabolism in the muscle as well as in the paracrine/endocrine regulation of other tissues and organs including adipose tissue, the liver, and the brain through their receptors. Till date, secretome analysis of human myocyte culture medium has revealed over 600 myokines. In this review article, we summarize our current knowledge of major identified and characterized myokines focusing on their biological activity and function, particularly in muscle mass and function.
Introduction
The muscle is a tissue composed of cells or fibers that produce force and movement of the body. They are primarily responsible for maintaining and changing body position, locomotion as well as the movement of internal organs. Different types of muscles perform different functions according to their location and type. Skeletal muscles are one of the most dynamic tissues involved in voluntary contraction according to command (Frontera and Ochala, 2015; Noto and Edens, 2018). They comprise approximately 40% of the total body weight (Frontera and Ochala, 2015; Noto and Edens, 2018). In contrast, cardiac and smooth muscles are associated with involuntary contraction without awareness (Frontera and Ochala, 2015; Hafen and Burns, 2018; Noto and Edens, 2018). Smooth muscles are found throughout the body and tightly regulate many of the body subsystems implicated in maintaining survival (Hafen and Burns, 2018).
Myokines are cytokines or peptides synthesized and released by myocytes in muscle tissue in response to muscular contractions (Pedersen et al., 2007). The term “myokine” was first introduced by a Swedish scientist, Bengt Saltin, in 2003 (Pedersen et al., 2003). Myokines are implicated in the autocrine regulation of metabolism in muscles as well as in the para/endocrine regulation of other tissues and organs including the adipose tissue, liver, and brain (Carson, 2017) through their receptors. Since myostatin was first identified as a myokine in 1997, secretome-based analysis of human myocyte culture medium has revealed over 600 myokines till date (Gorgens et al., 2015). However, the majority of these myokines are still not sufficiently characterized. Only few of them have been studied for their biological activity and function and have provided some clear evidence as being released directly from muscle contraction. Moreover, studies potentially associated with muscle atrophy barely exist. Understanding the biological and physiological roles of these myokines in skeletal muscle atrophy or weakness is important and valuable to find novel targets for therapeutic intervention.
In this review, we summarize our current knowledge focusing on myokines released directly by muscle contraction and their potential roles associated with skeletal muscle mass and function.
Myostatin
Myostatin, growth differentiation factor 8, was the first identified myokine in 1997 by Se-Jin Lee and his colleagues (McPherron et al., 1997). It is encoded by the myostatin gene and is known as a highly conserved member of the TGF beta protein family (McPherron et al., 1997). It is abundantly expressed in skeletal muscles, but is also expressed to a lesser extent in cardiac muscles and fat tissues (McPherron et al., 1997; Sharma et al., 1999). Myostatin levels in the plasma of healthy young men has been shown to significantly decrease within 24 h post-exercise when compared to pre-exercise and has also been shown to positively correlate with plasma IL-6 (Kazemi, 2016). In contrast, serum myostatin has been shown to increase in patients with spinal cord injury after aerobic exercise (Han et al., 2016). Although heavily contradicting reports exist on both sides, circulating myostatin shows an obvious increase in females rather than males during sarcopenia (Bergen et al., 2015), and a decrease in cancer-cachexia (Loumaye and Thissen, 2017) and generic neuromuscular disease patients (Awano et al., 2008; Anaya-Segura et al., 2015; Burch et al., 2017).
The effects of myostatin are mediated through the activin type IIB receptor (ActRIIB), which is expressed ubiquitously (Pistilli et al., 2011; Amthor and Hoogaars, 2012). The downstream mediators of myostatin, Smad2 and Smad3, are phosphorylated and form a complex with Smad4. This complex in turn stimulates FoxO-dependent transcription and regulates the transcription of genes associated with the proliferation and differentiation in skeletal muscle precursor cells as well as protein degradation pathways (such as the ubiquitin-proteasome processes, and autophagy) in mature myofibers (Burks and Cohn, 2011; Han et al., 2013). In addition, myostatin-mediated Smad signaling activation inhibits protein synthesis in muscle tissues by suppressing the Akt-mediated mTOR signaling pathway (Han et al., 2013). Functionally, myostatin is a negative regulator of muscle growth thereby leading to inhibition of myogenesis through muscle cell differentiation and growth (McPherron et al., 1997). Animals blocking myostatin activity with substance show significantly increased muscle mass (myofiber hypertrophy rather than hyperplasia) (Morvan et al., 2017). In myostatin-knockout mice, the muscle mass is approximately twice increased compared to that in normal mice (McPherron et al., 1997). In humans, individuals with mutations in both copies of the myostatin gene showed significantly increased muscle mass and muscle strength compared to that observed in normal individuals (Schuelke et al., 2004). Growing evidence indicates that increasing myostatin and its analog activin A contribute to the incidence of muscle atrophy (Morvan et al., 2017). Thus, myostatin is considered a promising target molecule for the treatment of muscle wasting. In the past two decades, several agents, such as follistatin (myostatin antagonist), and selective antibody-based approaches targeting ActR-IIB, myostatin, and activin A were developed to antagonize/suppress myostatin signaling. These molecules were evaluated under various pathological conditions such as muscular wasting or atrophy. For example, a myostatin antibody, MYO-029/stamulumab, was tested in broad muscle dystrophic models, including Becker’s muscular dystrophy (BMD) and facioscapulohumeral dystrophy, but failed to show clinical efficacy in elevating muscle strength (Leung et al., 2015). Overexpression of the follistatin isoform, FS344, using an AVV vector showed improved ambulation in patients with BMD and inclusion body myositis (Al-Zaidy et al., 2015; Mendell et al., 2015; Mendell et al., 2017). However, so far none of these treatments have proven to be clinically sufficient as shown in Table 1 (Cohen et al., 2015; Mariot et al., 2017). There are still obstacles (such as lack of target specificity and potential clinical toxicities) to overcome for their use in human patients. In addition, a recent study showed that activin A prominently regulates muscle mass in primates than does myostatin in rodents (Busquets et al., 2012; Cohen et al., 2015), suggesting that targeting myostatin alone may not be sufficient to treat muscle atrophy in humans.
Irisin
Irisin is a cleaved form of Fibronectin type III domain-containing protein 5 (FNDC5), which was simultaneously discovered by two independent groups in 2002 (Teufel et al., 2002; Colaianni et al., 2014) It was first reported as a potential mediator of the beneficial effect of exercise (Raschke et al., 2013b). Initially, the expression of PGC1α in muscle stimulates FNDC5 expression, which drives brown fat-like development of white fat cells named beige cells and increases thermogenesis (Bostrom et al., 2012). Although exercise-induced increase in the level of irisin in the blood is heavily debated (Pekkala et al., 2013), many reports have continuously shown an increase in FNDC5 mRNA expression upon exercise in rodent models (Dun et al., 2013; Roberts et al., 2013) and humans (Huh et al., 2012; Lecker et al., 2012), thus triggering renewed interest in exercise-induced myokines. In line with these observations, expression of mitochondrial-specific transcription factors, such as PGC-1α and mitochondrial transcription factor A, increases in C2C12 myotubes exposure to recombinant irisin for 24 h. They are all involved in elevated mitochondrial content and oxygen consumption (Vaughan et al., 2015). Moreover, irisin and myostatin are inversely secreted from skeletal muscles after physical exercise (MacKenzie et al., 2013), thereby suggesting its potential myogenic role. Reza et al. reported that irisin induced skeletal muscle hypertrophy and attenuated denervation-induced atrophy by activating IL-6 signaling in rodents (Reza et al., 2017). The effects of irisin on hypertrophy were shown to be established by the activation of muscle satellite cells and elevation of protein synthesis (Reza et al., 2017). This study substantially opened up potential research avenues on irisin with respect to muscle atrophy. Moreover, a latest study showed that circulating irisin levels were lower in women with postmenopausal sarcopenia when compared to those with pre-sarcopenia and that they negatively correlated with the quadricep cross-sectional area (Park et al., 2018), suggesting that irisin may also function as a potential pro-myogenic factor in human pathological conditions. Further studies are needed to reveal the biological effects of human irisin and the underlying mechanism in human skeletal muscles.
IL-6
Interleukin 6 (IL-6) was identified in 2000 and is the most studied myokine (Steensberg et al., 2000; Pedersen and Febbraio, 2008). It is secreted from muscles into the blood vessel in response to muscle contractions (Pedersen and Febbraio, 2008), by which skeletal muscles communicate with central and peripheral organs (Pedersen et al., 2003). The circulatory level of IL-6 is affected by both the duration and intensity of muscle contraction in humans (Steensberg et al., 2000; Helge et al., 2003). Interestingly, IL-6 is highly produced and released after post-exercise while insulin action is enhanced. However, IL-6 is also associated with obesity and insulin resistance (Pedersen and Febbraio, 2008). IL-6 has an insulin-like effect on glucose metabolism. IL-6 increases insulin-stimulated glucose disposal in humans as well as glucose uptake and fatty acid oxidation in vitro through AMP-activated protein kinase and PI3K-Akt signaling pathways (Al-Khalili et al., 2006; Carey et al., 2006). Individuals with spinal cord injury (SCI) are prone to develop metabolic diseases due to the lack of exercise-related IL-6 response, suggesting that IL-6 plays a pivotal role in regulating glucose homeostasis (Kouda et al., 2012).
On the other hand, the role of IL-6 on muscle atrophy seems to be a negative effect rather than a beneficial effect. Increased circulating angiotensin II (AngII) reduces lean body mass in chronic kidney disease. In mice, AngII infusion resulted in increased circulating IL-6 and its hepatic production, suggesting that AngII-induced inflammation might be a trigger for muscle loss (Zhang et al., 2009). In contrast, AngII-induced muscle atrophy was suppressed in IL-6-deficient mice (Zhang et al., 2009). IL-6 is overproduced in patients with Duchenne muscular dystrophy and in muscles of the mdx animal model. Inhibition of IL-6 activity with an interleukin-6 receptor (Il-6r) neutralizing antibody attenuates the dystrophic phenotype, severe muscle degeneration, inflammation, as well as accumulation of non-functional fat and fibrotic tissues (Wada et al., 2017). In addition, pharmacological inhibition of IL-6 activity in mdx male mice inhibits anti-inflammatory responses and improvement in muscle repair (Pelosi et al., 2015). Therefore, inhibition of IL-6 might be beneficial for preventing muscle loss.
Brain-Derived Neurotrophic Factor
Brain-derived neurotrophic factor (BDNF) is the second member of the neurotrophin family of growth factors, which regulates neuronal survival, plasticity, growth, and death through tropomyosin-related kinase receptor B (TrkB). It was for the first time purified from pig brain in 1982 (Barde et al., 1982). After 11 years, the BDNF gene was identified by two independent groups (Metsis et al., 1993; Binder and Scharfman, 2004). Initially, BDNF has been studied mostly in relation with nervous system development and function (Clow and Jasmin, 2010). However, the expression of several neurotrophin receptors is identified in skeletal muscles, thus implicating the certain role of BDNF. Indeed, Chevrel et al. (2006) reported that BDNF is differentially expressed in skeletal muscles according to physiological or pathological conditions. In adult skeletal muscles, BDNF is also expressed in muscle satellite cells (Mousavi et al., 2004) and is upregulated in muscle injury followed by the activation and proliferation of satellite cells, suggesting that BDNF might play an important role in mediating the satellite cell response to muscle injury (Omura et al., 2005). Jasmin et al. showed that BDNF substantially regulates satellite cell differentiation and skeletal muscle regeneration, by using BDNF null and muscle-specific BDNF KO mice (Clow and Jasmin, 2010). These results indicate that BDNF might be involved in the regulation of damaged muscles. Although there are many studies associated with the role of BDNF in muscle development and function, there is no clear evidence indicating that it is a myokine. In fact, the effect of muscle contraction on circulating BDNF levels is controversial. Some studies have reported no change in serum BDNF right after either acute or chronic exercise. On the other hand, several studies have shown that circulating BDNF increases with physical exercise (Ferris et al., 2007; Yarrow et al., 2010; Pereira et al., 2018). In skeletal muscle cells, BDNF mRNA expression is increased by contraction and increased fat oxidation through activation of AMP-activated protein kinase (Matthews et al., 2009). Overall, these studies suggest that muscle-derived BDNF is important for regulating muscle regeneration right after muscle injury. However, many key questions on the biological functions of BDNF in skeletal muscles remain unresolved. A major issue would be to elucidate the mechanism by which BDNF regulates satellite cell differentiation and skeletal muscle regeneration, and in which BDNF substantially recover muscle strength and function. Manipulating BDNF may thus represent an important therapeutic tool for alleviating dystrophic muscle atrophy.
IL-15
Interleukin-15 (IL-15) is a cytokine with a structure similar to interleukin-2 (IL-2). It was discovered by two different research groups in 1994 and was characterized as a T cell growth factor (Steel et al., 2012). Later on, several studies showed that IL-15 is accumulated in the muscles as a result of regular exercise training, indicating that it is a myokine (Pedersen, 2011; Tamura et al., 2011; Brunelli et al., 2015). Moreover, IL-15 mRNA expression is upregulated along with myoblast differentiation (Pedersen and Febbraio, 2008). Supportively, several studies showed that exogenously treated IL-15 or IL-15 overexpression promotes myoblast differentiation and increases muscle mass in the mouse C2 skeletal myogenic cell line (Quinn et al., 1995, 2002). In rats with cancer cachexia, IL-15 treatment attenuates skeletal muscle wasting by suppressing protein degradation through inhibition of the ATP-dependent ubiquitin proteolytic pathway (Carbo et al., 2000). IL-15 administration was found to improve diaphragm strength with increased muscle fiber cross-sectional area and decreased collagen accumulation in dystrophic mdx mice (Harcourt et al., 2005). In contrast, systemic infusion of IL-15 induces muscle atrophy in skeletal muscles of rodents (Pistilli and Alway, 2008). IL-15 treatment increased the glucose uptake in skeletal muscle cells via activation of the Jak3/STAT3 signaling pathway (Krolopp et al., 2016) or the AMPK signaling pathway (Gray and Kamolrat, 2011). In addition, Quinn L et al. and coworkers reported that IL-15 transgenic mice exhibited increased fat oxidation, energy expenditure and running endurance even with lower muscle mass compared to that in wild type mice. Interestingly, these mice also expressed troponin I and myosin heavy chain mRNA isoform indicating the conversion of muscles to a more oxidative phenotype (Quinn et al., 2013; Chalkiadaki et al., 2014). Collectively, the above controversial reports indicate that IL-15 acts differently according to the normal and pathological conditions. Thus, further studies should be focused on clarifying the different factors influencing the varying roles of IL-15 between different physiological conditions.
Myonectin (CTRP15)
Myonectin is a myokine belonging to the C1q/TNF-related protein (CTRP) family, and was discovered by Seldin et al. (2012). It is a novel nutrient-responsive myokine secreted from skeletal muscles (Seldin et al., 2012; Peterson et al., 2014). Myonectin is released into the blood stream by muscular contraction, and is functionally similar to insulin as it promotes fatty acid uptake into cells by increased expression of fatty acid transport genes (CD36, FATP1, Fabp1, and Fabp4) (Seldin and Wong, 2012; Seldin et al., 2012). In the mouse liver and cultured hepatocytes, recombinant myonectin treatment suppresses starvation-induced autophagy by inhibiting LC3-dependent autophagosome formation, p62 degradation, and other autophagy-related genes expression. Furthermore, the ability of myonectin to suppress autophagy is abolished by inhibition of the PI3K/Akt/mTOR signaling pathway (Seldin et al., 2013). Autophagy is considered to be a mechanism that induces muscle atrophy (Bonaldo and Sandri, 2013). In addition, the PI3K/Akt signaling pathway is involved in anabolic responses in the body. Therefore, these observations indicate that myonectin may play an important role in increasing muscle mass by elevating protein synthesis and inhibiting protein degradation. On a related note, mitochondrial content in muscles is an important determinant for muscle type and function. Myonectin is remarkably increased following depletion of mtDNA and increases glucose uptake and fatty acid oxidation through activation of the AMPK signaling pathway in rat skeletal myocytes (Park et al., 2009; Lim et al., 2012). Interestingly, oxidative, slow-twitch muscle fiber types express a higher level of myonectin than does glycolytic, fast-twitch muscle fiber types, suggesting that it may be involved in mitochondrial biogenesis and sensing cellular energy state (Seldin and Wong, 2012). However, there is no study associated with its biological function and mechanism on muscle mass and muscle mitochondrial biogenesis in normal physiology and in the diseased state.
Decorin
Decorin is small leucine-rich proteoglycan identified as a myokine by Kanzleiter et al. (2014). It is secreted in skeletal muscles during muscular contraction and plays an important role in muscle growth. Decorin directly binds to and inactivates myostatin (a potent inhibitor of muscle growth) in a zinc-dependent manner, and inhibits its anti-myogenic effects (El Shafey et al., 2016). In vivo over-expression of Decorin in murine skeletal muscles promotes expression of the pro-myogenic factor Mighty (Marshall et al., 2008). Mighty is ubiquitously expressed but appears to be negatively regulated by myostatin in skeletal muscles. Decorin overexpression increases the expression of Myod1 and follistatin, whereas it reduces the muscle-specific ubiquitin ligases atrogin1 and MuRF1 (Marshall et al., 2008). Thus, Decorin might act as a myogenic factor and might be a possible therapeutic target for the treatment of muscle wasting.
Fibroblast Growth Factor (FGF) 21
Fibroblast growth factors (FGFs) are signaling proteins with diverse biological functions in development and metabolism. FGFs are classified as para, intra, and endocrine according to their action manners. Paracrine FGFs mostly function as local signaling molecules in developmental processes whereas intracrine FGFs mainly serve as intracellular molecules in neuronal processes (Itoh and Ornitz, 2011). FGF21 functions as endocrinal hormone-like or local signaling molecules in metabolism. FGF21 does not have proliferative activity as other paracrine and endocrine FGFs family and is only associated with metabolism (Itoh, 2014). FGFs activate several intracellular signaling pathways including phosphatidylinositol 3-kinase (PI3K)/serine-threonine protein kinase AKT, signaling transducer and activator of transcription (STAT), mitogen activation protein kinase (MAPK), and phosphoinositide phospholipase C (PLC) γ (Itoh, 2014). Specifically, FGF21 acts through FGF receptor 1c with β-Klotho as a cofactor. Skeletal muscle-specific Akt1 transgenic mice showed skeletal muscle fiber hypertrophy with increasing Fgf21 expression in the muscle and in serum indicating that FGF21 plays an important role in regulating muscle mass (Izumiya et al., 2008). In addition, FGF21 expression is coupled to mitochondrial dysfunction and insults of various stresses in skeletal muscles. Autophagy deficiency and subsequent mitochondrial dysfunction elevates the level of FGF21 as a myokine, thereby protecting against diet-induced obesity and insulin resistance (Kim et al., 2013; Keipert et al., 2014). In cultured myoblasts, mitochondrial complex inhibitor treatment increased expression by promoting the binding of activating transcription factor 2 (ATF2) for the promoter region of the Fgf21 gene (Ribas et al., 2014). Moreover, in human brain vascular smooth muscle cells, FGF21 protects against angiotensin II-induced cerebrovascular aging by elevating mitochondrial biogenesis (Wang et al., 2016). All the above studies suggest that FGF21 may be potentially involved in switching muscle type and regulating mitophagy, thereby regulating muscle mass and function. Thus, targeting FGF21 might be an attractive approach to treat mitochondrial-based myopathy and muscle dysfunction.
Secreted Protein Acidic and Rich in Cysteine (SPARC)
Aoi et al. (2013) reported SPARC/osteonectin as a novel myokine, which is released from the skeletal muscles of both humans and mice after exercise, even though it was identified earlier (Aoi et al., 2013). Exercise-stimulated SPARC secretion was shown to inhibit colon tumorigenesis by enhancing apoptosis in colon cancer cells (Aoi et al., 2013). SPARC was also shown to be upregulated in inherited and idiopathic muscle wasting diseases such as Duchenne muscular dystrophy and congenital muscular dystrophy (Jorgensen et al., 2009). SPARC overexpression almost completely abolished myogenic differentiation in the muscle progenitor cell line, C2C12 (Petersson et al., 2013). Thus, SPARC may play a certain functional role in the repair of muscle damage in muscle satellite cells. However, very limited studies associated with the role of myokines are currently available. Further studies need to first determine and address the expression profile and role of SPARC in muscle development and regeneration. The underlying signaling pathways also need to be studied in detail.
Conclusion
Skeletal muscle atrophy is an emerging medical problem worldwide owing to increasing elderly populations and various classical reasons including genetic mutation, disease-derived cachexia, and accidents. However, although our understanding of molecular mechanisms regulating muscle atrophy/muscle weakness has substantially progressed, there is no specific treatment for muscle atrophy. Recently, a number of myokines have been identified through secretome analysis and some have proven to be very informative while searching for novel myokines (Raschke et al., 2013a; Hartwig et al., 2014; Grube et al., 2018). However, the majority of myokines are not still sufficiently characterized with regard to their biological activity and function. Only few myokines have been restrictively characterized (Figure 1) and their potential signaling pathways, implicated in muscle cell proliferation, differentiation, and growth in order to maintain muscle mass, muscle strength, and function, been identified (Figure 2). Therefore, it is important to better understand their precise role and function on skeletal muscles under normal physiological and pathophysiological conditions. Targeting novel myokines for either increasing or suppressing their functional activity in certain pathological states could be an attractive novel therapeutic tool for combating skeletal muscle atrophy.
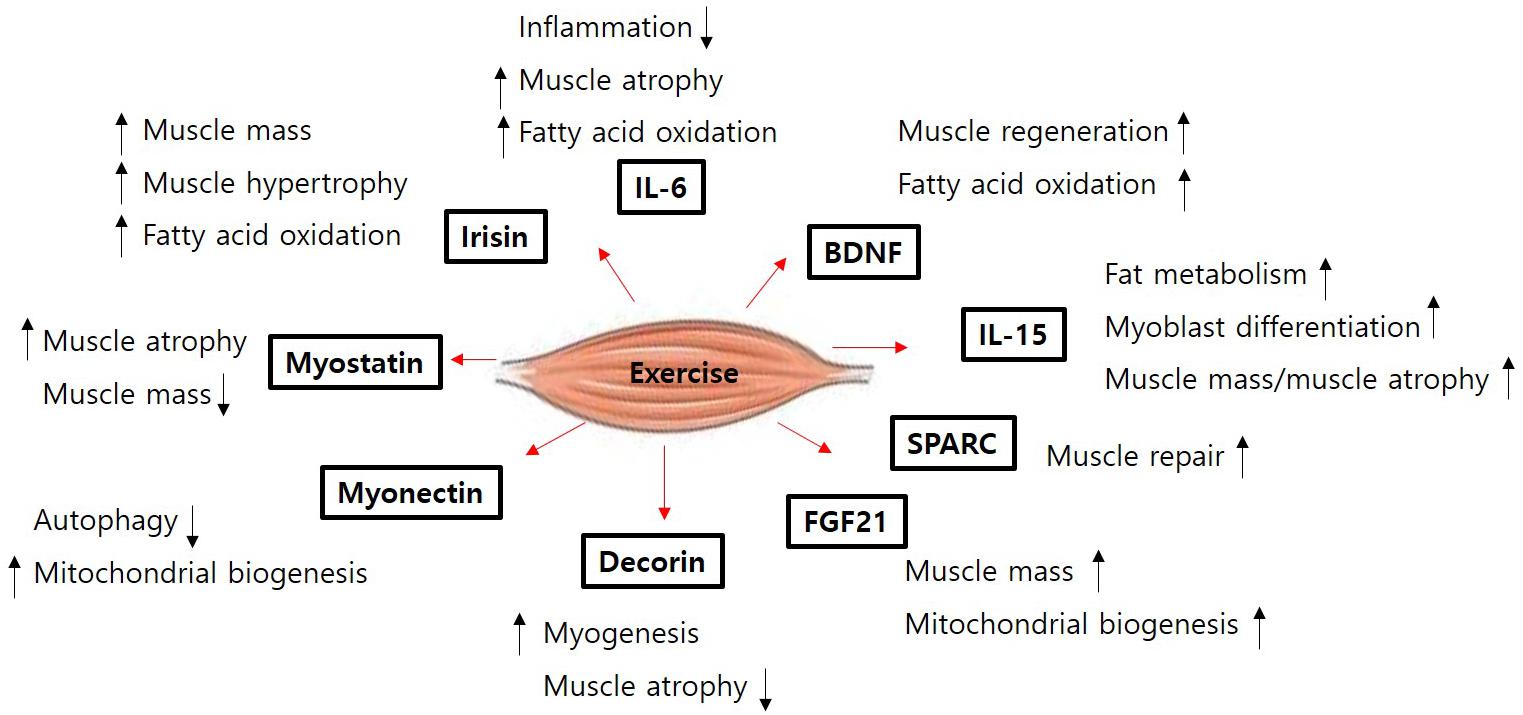
Figure 1. The function of muscle contraction-induced myokines. The figure shows the selected the functions for each myokines released from muscle contraction (exercise) in muscle. BDNF, brain-derived neurotrophic factor; FGF21, fibroblast growth factor 21; SPARC, secreted protein acidic and rich in cysteine; IL, interleukin.
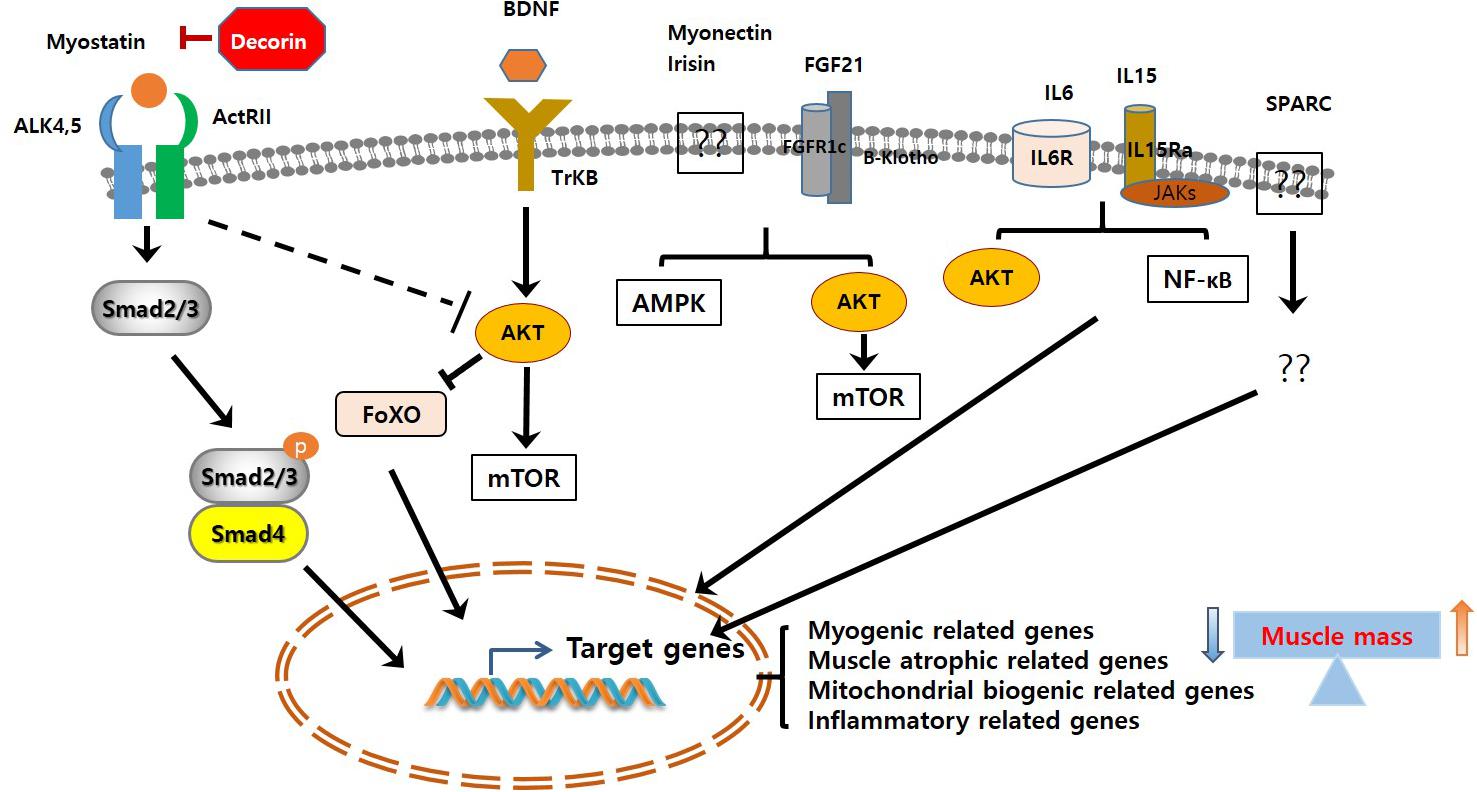
Figure 2. Signaling pathways of muscle contraction-induced myokines. The myokine-mediated signaling pathways lead to its target genes expression, which in turn regulate muscle cell proliferation, differentiation, and growth. It ultimately increases/decreases muscle mass. ALK, activin receptor-like kinase; ActRIIB, activin type IIB receptor; BDNF, brain-derived neurotrophic factor; TrKB, tropomyosin-related kinase receptor B; FGF21, fibroblast growth factor 21; SPARC, secreted protein acidic and rich in cysteine.
Author Contributions
Both authors conceived and wrote the manuscript and approved it for publication.
Funding
This work was supported by a Basic Science Research Program grant of the National Research Foundation of Korea, the Ministry of Education of South Korea (2017R1D1A1B03036210 to JL) and the Korea Health Technology R&D Project through the Korea Health Industry Development Institute (KHIDI), funded by the Ministry of Health and Welfare, South Korea (HI14C1135 to H-SJ).
Conflict of Interest Statement
The authors declare that the research was conducted in the absence of any commercial or financial relationships that could be construed as a potential conflict of interest.
References
Al-Khalili, L., Bouzakri, K., Glund, S., Lonnqvist, F., Koistinen, H. A., and Krook, A. (2006). Signaling specificity of interleukin-6 action on glucose and lipid metabolism in skeletal muscle. Mol. Endocrinol. 20, 3364–3375. doi: 10.1210/me.2005-0490
Al-Zaidy, S. A., Sahenk, Z., Rodino-Klapac, L. R., Kaspar, B., and Mendell, J. R. (2015). Follistatin gene therapy improves ambulation in becker muscular dystrophy. J. Neuromuscul. Dis. 2, 185–192. doi: 10.3233/JND-150083
Amthor, H., and Hoogaars, W. M. (2012). Interference with myostatin/ActRIIB signaling as a therapeutic strategy for Duchenne muscular dystrophy. Curr. Gene Ther. 12, 245–259. doi: 10.2174/156652312800840577
Anaya-Segura, M. A., Garcia-Martinez, F. A., Montes-Almanza, L. A., Diaz, B. G., Avila-Ramirez, G., Alvarez-Maya, I., et al. (2015). Non-invasive biomarkers for duchenne muscular dystrophy and carrier detection. Molecules 20, 11154–11172. doi: 10.3390/molecules200611154
Aoi, W., Naito, Y., Takagi, T., Tanimura, Y., Takanami, Y., Kawai, Y., et al. (2013). A novel myokine, secreted protein acidic and rich in cysteine (SPARC), suppresses colon tumorigenesis via regular exercise. Gut 62, 882–889. doi: 10.1136/gutjnl-2011-300776
Awano, H., Takeshima, Y., Okizuka, Y., Saiki, K., Yagi, M., and Matsuo, M. (2008). Wide ranges of serum myostatin concentrations in Duchenne muscular dystrophy patients. Clin. Chim. Acta 391, 115–117. doi: 10.1016/j.cca.2008.01.024
Barde, Y. A., Edgar, D., and Thoenen, H. (1982). Purification of a new neurotrophic factor from mammalian brain. EMBO J. 1, 549–553. doi: 10.1002/j.1460-2075.1982.tb01207.x
Bergen, H. R. III, Farr, J. N., Vanderboom, P. M., Atkinson, E. J., White, T. A., Singh, R. J., et al. (2015). Myostatin as a mediator of sarcopenia versus homeostatic regulator of muscle mass: insights using a new mass spectrometry-based assay. Skeletal Muscle 5:21. doi: 10.1186/s13395-015-0047-5
Binder, D. K., and Scharfman, H. E. (2004). Brain-derived neurotrophic factor. Growth Factors 22, 123–131. doi: 10.1080/08977190410001723308
Bonaldo, P., and Sandri, M. (2013). Cellular and molecular mechanisms of muscle atrophy. Dis. Models Mech. 6, 25–39. doi: 10.1242/dmm.010389
Bostrom, P., Wu, J., Jedrychowski, M. P., Korde, A., Ye, L., Lo, J. C., et al. (2012). A PGC1-alpha-dependent myokine that drives brown-fat-like development of white fat and thermogenesis. Nature 481, 463–468. doi: 10.1038/nature10777
Brunelli, D. T., Chacon-Mikahil, M. P., Gaspari, A. F., Lopes, W. A., Bonganha, V., Bonfante, I. L., et al. (2015). Combined training reduces subclinical inflammation in obese middle-age men. Med. Sci. Sports Exerc. 47, 2207–2215. doi: 10.1249/MSS.0000000000000658
Burch, P. M., Pogoryelova, O., Palandra, J., Goldstein, R., Bennett, D., Fitz, L., et al. (2017). Reduced serum myostatin concentrations associated with genetic muscle disease progression. J. Neurol. 264, 541–553. doi: 10.1007/s00415-016-8379-6
Burks, T. N., and Cohn, R. D. (2011). Role of TGF-beta signaling in inherited and acquired myopathies. Skeletal Muscle 1:19. doi: 10.1186/2044-5040-1-19
Busquets, S., Toledo, M., Orpi, M., Massa, D., Porta, M., Capdevila, E., et al. (2012). Myostatin blockage using actRIIB antagonism in mice bearing the Lewis lung carcinoma results in the improvement of muscle wasting and physical performance. J. Cachexia Sarcopenia Muscle 3, 37–43. doi: 10.1007/s13539-011-0049-z
Carbo, N., Lopez-Soriano, J., Costelli, P., Busquets, S., Alvarez, B., Baccino, F. M., et al. (2000). Interleukin-15 antagonizes muscle protein waste in tumour-bearing rats. Br. J. Cancer 83, 526–531. doi: 10.1054/bjoc.2000.1299
Carey, A. L., Steinberg, G. R., Macaulay, S. L., Thomas, W. G., Holmes, A. G., Ramm, G., et al. (2006). Interleukin-6 increases insulin-stimulated glucose disposal in humans and glucose uptake and fatty acid oxidation in vitro via AMP-activated protein kinase. Diabetes 55, 2688–2697. doi: 10.2337/db05-1404
Carson, B. P. (2017). The potential role of contraction-induced myokines in the regulation of metabolic function for the prevention and treatment of type 2 diabetes. Front. Endocrinol. 8:97. doi: 10.3389/fendo.2017.00097
Chalkiadaki, A., Igarashi, M., Nasamu, A. S., Knezevic, J., and Guarente, L. (2014). Muscle-specific SIRT1 gain-of-function increases slow-twitch fibers and ameliorates pathophysiology in a mouse model of duchenne muscular dystrophy. PLoS Genet. 10:e1004490. doi: 10.1371/journal.pgen.1004490
Chevrel, G., Hohlfeld, R., and Sendtner, M. (2006). The role of neurotrophins in muscle under physiological and pathological conditions. Muscle Nerve 33, 462–476. doi: 10.1002/mus.20444
Clow, C., and Jasmin, B. J. (2010). Brain-derived neurotrophic factor regulates satellite cell differentiation and skeltal muscle regeneration. Mol. Biol. Cell 21, 2182–2190. doi: 10.1091/mbc.E10-02-0154
Cohen, S., Nathan, J. A., and Goldberg, A. L. (2015). Muscle wasting in disease: molecular mechanisms and promising therapies. Nat. Rev. Drug Discov. 14, 58–74. doi: 10.1038/nrd4467
Colaianni, G., Cuscito, C., Mongelli, T., Oranger, A., Mori, G., Brunetti, G., et al. (2014). Irisin enhances osteoblast differentiation in vitro. Int. J. Endocrinol. 2014:902186. doi: 10.1155/2014/902186
Dun, S. L., Lyu, R. M., Chen, Y. H., Chang, J. K., Luo, J. J., and Dun, N. J. (2013). Irisin-immunoreactivity in neural and non-neural cells of the rodent. Neuroscience 240, 155–162. doi: 10.1016/j.neuroscience.2013.02.050
El Shafey, N., Guesnon, M., Simon, F., Deprez, E., Cosette, J., Stockholm, D., et al. (2016). Inhibition of the myostatin/Smad signaling pathway by short decorin-derived peptides. Exp. Cell Res. 341, 187–195. doi: 10.1016/j.yexcr.2016.01.019
Ferris, L. T., Williams, J. S., and Shen, C. L. (2007). The effect of acute exercise on serum brain-derived neurotrophic factor levels and cognitive function. Med. Sci. Sports Exerc. 39, 728–734. doi: 10.1249/mss.0b013e31802f04c7
Frontera, W. R., and Ochala, J. (2015). Skeletal muscle: a brief review of structure and function. Calcified Tissue Int. 96, 183–195. doi: 10.1007/s00223-014-9915-y
Gorgens, S. W., Eckardt, K., Jensen, J., Drevon, C. A, and Eckel, J. (2015). Exercise and regulation of adipokine and myokine production. Prog. Mol. Biol. Transl. Sci. 135, 313–336. doi: 10.1016/bs.pmbts.2015.07.002
Gray, S. R, and Kamolrat, T. (2011). The effect of exercise induced cytokines on insulin stimulated glucose transport in C2C12 cells. Cytokine 55, 221–228. doi: 10.1016/j.cyto.2011.04.019
Grube, L., Dellen, R., Kruse, F., Schwender, H., Stuhler, K., and Poschmann, G. (2018). Mining the secretome of C2C12 muscle cells: data dependent experimental approach to analyze protein secretion using label-free quantification and peptide based analysis. J. Proteome Res. 17, 879–890. doi: 10.1021/acs.jproteome.7b00684
Han, D. S., Hsiao, M. Y., Wang, T. G., Chen, S. Y., and Yang, W. S. (2016). Association of serum myokines and aerobic exercise training in patients with spinal cord injury: an observational study. BMC Neurol. 16:142. doi: 10.1186/s12883-016-0661-9
Han, H. Q., Zhou, X., Mitch, W. E., and Goldberg. A. L. (2013). Myostatin/activin pathway antagonism: molecular basis and therapeutic potential. Int. J. Biochem. Cell Biol. 45, 2333–2347. doi: 10.1016/j.biocel.2013.05.019
Harcourt, L. J., Holmes, A. G., Gregorevic, P., Schertzer, J. D., Stupka, N., Plant. D. R., and Lynch, G. S. (2005). Interleukin-15 administration improves diaphragm muscle pathology and function in dystrophic mdx mice. Am. J. Pathol. 166, 1131–1141. doi: 10.1016/S0002-9440(10)62333-4
Hartwig, S., Raschke, S., Knebel, B., Scheler, M., Irmler, M., Passlack, W., et al. (2014). Secretome profiling of primary human skeletal muscle cells. Biochim. Biophys. Acta 1844, 1011–1017. doi: 10.1016/j.bbapap.2013.08.004
Helge, J. W., Stallknecht, B., Pedersen, B. K., Galbo, H., Kiens, B., and Richter, E. A. (2003). The effect of graded exercise on IL-6 release and glucose uptake in human skeletal muscle. J. Physiol. 546(Pt 1), 299–305. doi: 10.1113/jphysiol.2002.030437
Huh, J. Y., Panagiotou, G., Mougios, V., Brinkoetter, M., Vamvini, M. T., Schneider, B. E., et al. (2012). FNDC5 and irisin in humans: I. Predictors of circulating concentrations in serum and plasma and II. mRNA expression and circulating concentrations in response to weight loss and exercise. Metabolism 61, 1725–1738. doi: 10.1016/j.metabol.2012.09.002
Itoh, N. (2014). FGF21 as a hepatokine, adipokine, and myokine in metabolism and diseases. Front. Endocrinol. 5:107. doi: 10.3389/fendo.2014.00107
Itoh, N., and Ornitz, D. M. (2011). Fibroblast growth factors: from molecular evolution to roles in development, metabolism and disease. J. Biochem. 149, 121–130. doi: 10.1093/jb/mvq121
Izumiya, Y., Bina, H. A., Ouchi, N., Akasaki, Y., Kharitonenkov, A., and Walsh, K. (2008). FGF21 is an Akt-regulated myokine. FEBS Lett. 582, 3805–3810. doi: 10.1016/j.febslet.2008.10.021
Jorgensen, L. H., Petersson, S. J., Sellathurai, J., Andersen, D. C., Thayssen, S., Sant, D. J., et al. (2009). Secreted protein acidic and rich in cysteine (SPARC) in human skeletal muscle. J. Histochem. Cytochem. 57, 29–39. doi: 10.1369/jhc.2008.951954
Kanzleiter, T., Rath, M., Gorgens, S. W., Jensen, J., Tangen, D. S., Kolnes, A. J., et al. (2014). The myokine decorin is regulated by contraction and involved in muscle hypertrophy. Biochem. Biophys. Res. Commun. 450, 1089–1094. doi: 10.1016/j.bbrc.2014.06.123
Kazemi, F. (2016). The correlation of resistance exercise-induced myostatin with insulin resistance and plasma cytokines in healthy young men. J. Endocrinol. Investigat. 39, 383–388. doi: 10.1007/s40618-015-0373-9
Keipert, S., Ost, M., Johann, K., Imber, F., Jastroch, M., van Schothorst, E. M., et al. (2014). Skeletal muscle mitochondrial uncoupling drives endocrine cross-talk through the induction of FGF21 as a myokine. Am. J. Physiol. Endocrinol. Metab. 306, E469–E482. doi: 10.1152/ajpendo.00330.2013
Kim, K. H., Jeong, Y. T., Oh, H., Kim, S. H., Cho, J. M., Kim, Y. N., et al. (2013). Autophagy deficiency leads to protection from obesity and insulin resistance by inducing Fgf21 as a mitokine. Nat. Med. 19, 83–92. doi: 10.1038/nm.3014
Kouda, K., Furusawa, K., Sugiyama, H., Sumiya, T., Ito, T., Tajima, F., et al. (2012). Does 20-min arm crank ergometer exercise increase plasma interleukin-6 in individuals with cervical spinal cord injury? Eur. J. Appl. Physiol. 112, 597–604. doi: 10.1007/s00421-011-2004-2
Krolopp, J. E., Thornton, S. M., and Abbott, M. J. (2016). IL-15 activates the Jak3/STAT3 signaling pathway to mediate glucose uptake in skeletal muscle cells. Front. Physiol. 7:626. doi: 10.3389/fphys.2016.00626
Lecker, S. H., Zavin, A., Cao, P., Arena, R., Allsup, K., Daniels, K. M., et al. (2012). Expression of the irisin precursor FNDC5 in skeletal muscle correlates with aerobic exercise performance in patients with heart failure. Circ. Heart Failure 5, 812–818. doi: 10.1161/CIRCHEARTFAILURE.112.969543
Leung, D. G., Carrino, J. A., Wagner, K. R., and Jacobs, M. A. (2015). Whole-body magnetic resonance imaging evaluation of facioscapulohumeral muscular dystrophy. Muscle Nerve 52, 512–520. doi: 10.1002/mus.24569
Lim, S., Choi, S. H., Koo, B. K., Kang, S. M., Yoon, J. W., Jang, H. C., et al. (2012). Effects of aerobic exercise training on C1q tumor necrosis factor alpha-related protein isoform 5 (myonectin): association with insulin resistance and mitochondrial DNA density in women. J. Clin. Endocrinol. Metab. 97, E88–E93. doi: 10.1210/jc.2011-1743
Loumaye, A., and Thissen, J. P. (2017). Biomarkers of cancer cachexia. Clin. Biochem. 50, 1281–1288. doi: 10.1016/j.clinbiochem.2017.07.011
MacKenzie, M. G., Hamilton, D. L., Pepin, M., Patton, A., and Baar, K. (2013). Inhibition of myostatin signaling through Notch activation following acute resistance exercise. PLoS One 8:e68743. doi: 10.1371/journal.pone.0068743
Mariot, V., Joubert. R., Hourde, C., Feasson, L., Hanna, M., Muntoni, F., et al. (2017). Downregulation of myostatin pathway in neuromuscular diseases may explain challenges of anti-myostatin therapeutic approaches. Nat. Commun. 8:1859. doi: 10.1038/s41467-017-01486-4
Marshall, A., Salerno, M. S., Thomas, M., Davies, T., Berry, C., Dyer, K., et al. (2008). Mighty is a novel promyogenic factor in skeletal myogenesis. Exp. Cell Res. 314, 1013–1029. doi: 10.1016/j.yexcr.2008.01.004
Matthews, V. B., Astrom, M. B., Chan, M. H., Bruce, C. R., Krabbe, K. S., Prelovsek, O., et al. (2009). Brain-derived neurotrophic factor is produced by skeletal muscle cells in response to contraction and enhances fat oxidation via activation of AMP-activated protein kinase. Diabetologia 52, 1409–1418. doi: 10.1007/s00125-009-1364-1
McPherron, A. C., Lawler, A. M., and Lee, S. J. (1997). Regulation of skeletal muscle mass in mice by a new TGF-beta superfamily member. Nature 387, 83–90. doi: 10.1038/387083a0
Mendell, J. R., Sahenk. Z., Al-Zaidy, S., Rodino-Klapac, L. R., Lowes, L. P., Alfano, L. N., et al. (2017). Follistatin gene therapy for sporadic inclusion body myositis improves functional outcomes. Mol. Ther. 25, 870–879. doi: 10.1016/j.ymthe.2017.02.015
Mendell, J. R., Sahenk, Z., Malik, V., Gomez, A. M., Flanigan, K. M., Lowes, L. P., et al. (2015). A phase 1/2a follistatin gene therapy trial for becker muscular dystrophy. Mol. Ther. 23, 192–201. doi: 10.1038/mt.2014.200
Metsis, M., Timmusk, T., Arenas, E., and Persson, H. (1993). Differential usage of multiple brain-derived neurotrophic factor promoters in the rat brain following neuronal activation. Proc. Natl. Acad. Sci. U.S.A. 90, 8802–8806. doi: 10.1073/pnas.90.19.8802
Morvan, F., Rondeau, J. M., Zou, C., Minetti, G., Scheufler, C., Scharenberg, M., et al. (2017). Blockade of activin type II receptors with a dual anti-ActRIIA/IIB antibody is critical to promote maximal skeletal muscle hypertrophy. Proc. Natl. Acad. Sci. U.S.A. 114, 12448–12453. doi: 10.1073/pnas.1707925114
Mousavi, K., Parry, D. J., and Jasmin, B. J. (2004). BDNF rescues myosin heavy chain IIB muscle fibers after neonatal nerve injury. Am. J. Physiol. Cell Physiol. 287, C22–C29. doi: 10.1152/ajpcell.00583.2003
Omura, T., Sano, M., Omura, K., Hasegawa, T., Doi, M., Sawada, T., and Nagano, A. (2005). Different expressions of BDNF, NT3, and NT4 in muscle and nerve after various types of peripheral nerve injuries. J. Peripheral Nerv. Syst. 10, 293–300. doi: 10.1111/j.1085-9489.2005.10307.x
Park, H. S., Kim, H. C., Zhang, D., Yeom, H., and Lim, S. K. (2018). The novel myokine irisin: clinical implications and potential role as a biomarker for sarcopenia in postmenopausal women. Endocrine doi: 10.1007/s12020-018-1814-y [Epub ahead of print].
Park, S. Y., Choi, J. H., Ryu, H. S., Pak, Y. K., Park, K. S., Lee, H. K., and Lee. W. (2009). C1q tumor necrosis factor alpha-related protein isoform 5 is increased in mitochondrial DNA-depleted myocytes and activates AMP-activated protein kinase. J. Biol. Chem. 284, 27780–27789. doi: 10.1074/jbc.M109.005611
Pedersen, B. K. (2011). Muscles and their myokines. J. Exp. Biol. 214(Pt 2), 337–346. doi: 10.1242/jeb.048074
Pedersen, B. K., and Febbraio, M. A. (2008). Muscle as an endocrine organ: focus on muscle-derived interleukin-6. Physiol. Rev. 88, 1379–1406. doi: 10.1152/physrev.90100.2007
Pedersen, B. K., Akerstrom, T. C., Nielsen, A. R., and Fischer, C. P. (2007). Role of myokines in exercise and metabolism. J. Appl. Physiol. 103, 1093–1098. doi: 10.1152/japplphysiol.00080.2007
Pedersen, B. K., Steensberg, A., Fischer, C., Keller, C., Keller, P., Plomgaard, P., et al. (2003). Searching for the exercise factor: is IL-6 a candidate? J. Muscle Res. Cell Motil. 24, 113–119. doi: 10.1023/A:1026070911202
Pekkala, S., Wiklund, P. K., Hulmi, J. J., Ahtiainen, J. P., Horttanainen, M., Pollanen, E., et al. (2013). Are skeletal muscle FNDC5 gene expression and irisin release regulated by exercise and related to health? J. Physiol. 591, 5393–5400. doi: 10.1113/jphysiol.2013.263707
Pelosi, L., Berardinelli, M. G., De Pasquale, L., Nicoletti, C., D’Amico, A., Carvello, F., et al. (2015). Functional and morphological improvement of dystrophic muscle by interleukin 6 receptor blockade. eBioMedicine 2, 285–293. doi: 10.1016/j.ebiom.2015.02.014
Pereira, E. S., Krause Neto, W., Calefi, A. S., Georgetti, M., Guerreiro, L., Zocoler, C. A. S., et al. (2018). Significant acute response of brain-derived neurotrophic factor following a session of extreme conditioning program is correlated with volume of specific exercise training in trained men. Front. Physiol. 9:823. doi: 10.3389/fphys.2018.00823.
Peterson, J. M., Mart, R., and Bond, C. E. (2014). Effect of obesity and exercise on the expression of the novel myokines, Myonectin and Fibronectin type III domain containing 5. PeerJ 2:e605. doi: 10.7717/peerj.605
Petersson, S. J., Jorgensen, L. H., Andersen, D. C., Norgaard, R. C., Jensen, C. H., and Schroder, H. D. (2013). SPARC is up-regulated during skeletal muscle regeneration and inhibits myoblast differentiation. Histol. Histopathol. 28, 1451–1460. doi: 10.14670/HH-28.1451
Pistilli, E. E., and Alway, S. E. (2008). Systemic elevation of interleukin-15 in vivo promotes apoptosis in skeletal muscles of young adult and aged rats. Biochem. Biophys. Res. Commun. 373, 20–24. doi: 10.1016/j.bbrc.2008.05.188
Pistilli, E. E., Bogdanovich, S., Goncalves, M. D., Ahima, R. S., Lachey, J., Seehra, J., et al. (2011). Targeting the activin type IIB receptor to improve muscle mass and function in the mdx mouse model of Duchenne muscular dystrophy. Am. J. Pathol. 178, 1287–1297. doi: 10.1016/j.ajpath.2010.11.071
Quinn, L. S., Anderson, B. G., Conner, J. D., and Wolden-Hanson, T. (2013). IL-15 overexpression promotes endurance, oxidative energy metabolism, and muscle PPARdelta, SIRT1, PGC-1alpha, and PGC-1beta expression in male mice. Endocrinology 154, 232–245. doi: 10.1210/en.2012-1773
Quinn, L. S., Anderson, B. G., Drivdahl, R. H., Alvarez, B., and Argiles, J. M. (2002). Overexpression of interleukin-15 induces skeletal muscle hypertrophy in vitro: implications for treatment of muscle wasting disorders. Exp. Cell Res. 280, 55–63. doi: 10.1006/excr.2002.5624
Quinn, L. S.,Haugk, K. L., and Grabstein, K. H. (1995). Interleukin-15: a novel anabolic cytokine for skeletal muscle. Endocrinology 136, 3669–3672. doi: 10.1210/endo.136.8.7628408
Raschke, S., Eckardt, K., Bjorklund Holven, K., Jensen, J., and Eckel. J. (2013a). Identification and validation of novel contraction-regulated myokines released from primary human skeletal muscle cells. PLoS One 8:e62008. doi: 10.1371/journal.pone.0062008
Raschke, S., Elsen, M., Gassenhuber, H., Sommerfeld, M., Schwahn, U., Brockmann, B., et al. (2013b). Evidence against a beneficial effect of irisin in humans. PLoS One 8:e73680. doi: 10.1371/journal.pone.0073680
Reza, M. M., Subramaniyam, N., Sim, C. M., Ge, X., Sathiakumar, D., McFarlane, C., et al. (2017). Irisin is a pro-myogenic factor that induces skeletal muscle hypertrophy and rescues denervation-induced atrophy. Nat. Commun. 8:1104. doi: 10.1038/s41467-017-01131-0
Ribas, F., Villarroya, J., Hondares, E., Giralt, M., and Villarroya, F. (2014). FGF21 expression and release in muscle cells: involvement of MyoD and regulation by mitochondria-driven signalling. Biochem. J. 463, 191–199. doi: 10.1042/BJ20140403
Roberts, M. D., Bayless, D. S., Company, J. M., Jenkins, N. T., Padilla. J., Childs, T. E., et al. (2013). Elevated skeletal muscle irisin precursor FNDC5 mRNA in obese OLETF rats. Metabolism 62, 1052–1056. doi: 10.1016/j.metabol.2013.02.002
Schuelke, M., Wagner, K. R., Stolz, L. E., Hubner, C., Riebel, T., Komen, W., et al. (2004). Myostatin mutation associated with gross muscle hypertrophy in a child. N. Engl. J. Med. 350, 2682–2688. doi: 10.1056/NEJMoa040933
Seldin, M. M., and Wong, G. W. (2012). Regulation of tissue crosstalk by skeletal muscle-derived myonectin and other myokines. Adipocyte 1, 200–202. doi: 10.4161/adip.20877
Seldin, M. M., Lei, X., Tan, S. Y., Stanson, K. P., Wei, Z., and Wong, G. W. (2013). Skeletal muscle-derived myonectin activates the mammalian target of rapamycin (mTOR) pathway to suppress autophagy in liver. J. Biol. Chem. 288, 36073–36082. doi: 10.1074/jbc.M113.500736
Seldin, M. M., Peterson, J. M., Byerly, M. S., Wei, Z., and Wong, G. W. (2012). Myonectin (CTRP15), a novel myokine that links skeletal muscle to systemic lipid homeostasis. J. Biol. Chem. 287, 11968–11980. doi: 10.1074/jbc.M111.336834
Sharma, M., Kambadur, R., Matthews, K. G., Somers, W. G., Devlin, G. P., Conaglen, J. V., et al. (1999). Myostatin, a transforming growth factor-beta superfamily member, is expressed in heart muscle and is upregulated in cardiomyocytes after infarct. J. Physiol. 180, 1–9.
Steel, J. C., Waldmann, T. A., and Morris, J. C. (2012). Interleukin-15 biology and its therapeutic implications in cancer. Trends Pharmacol. Sci. 33, 35–41. doi: 10.1016/j.tips.2011.09.004
Steensberg, A., van Hall, G., Osada, T., Sacchetti, M., Saltin, B., and Klarlund Pedersen, B. (2000). Production of interleukin-6 in contracting human skeletal muscles can account for the exercise-induced increase in plasma interleukin-6. J. Physiol. 529(Pt 1), 237–242. doi: 10.1111/j.1469-7793.2000.00237.x
Tamura, Y., Watanabe, K., Kantani, T., Hayashi, J., Ishida, N., and Kaneki, M. (2011). Upregulation of circulating IL-15 by treadmill running in healthy individuals: is IL-15 an endocrine mediator of the beneficial effects of endurance exercise? Endocrine J. 58, 211–215. doi: 10.1507/endocrj.K10E-400
Teufel, A., Malik, N., Mukhopadhyay, M., and Westphal, H. (2002). Frcp1 and Frcp2, two novel fibronectin type III repeat containing genes. Gene 297, 79–83. doi: 10.1016/S0378-1119(02)00828-4
Vaughan, R. A., Gannon, N. P., Mermier, C. M., and Conn, C. A. (2015). Irisin, a unique non-inflammatory myokine in stimulating skeletal muscle metabolism. J. Physiol. Biochem. 71, 679–689. doi: 10.1007/s13105-015-0433-9
Wada, E., Tanihata, J., Iwamura, A., Takeda, S., Hayashi, Y. K., and Matsuda, R. (2017). Treatment with the anti-IL-6 receptor antibody attenuates muscular dystrophy via promoting skeletal muscle regeneration in dystrophin-/utrophin-deficient mice. Skeletal Muscle 7:23. doi: 10.1186/s13395-017-0140-z
Wang, X. M., Xiao, H., Liu, L. L., Cheng, D., Li, X. J., and Si. L. Y. (2016). FGF21 represses cerebrovascular aging via improving mitochondrial biogenesis and inhibiting p53 signaling pathway in an AMPK-dependent manner. Exp. Cell Res. 346, 147–156. doi: 10.1016/j.yexcr.2016.06.020
Yarrow, J. F., White, L. J., McCoy, S. C., and Borst, S. E. (2010). Training augments resistance exercise induced elevation of circulating brain derived neurotrophic factor (BDNF). Neurosci. Lett. 479, 161–165. doi: 10.1016/j.neulet.2010.05.058
Keywords: muscular contraction, myocytes, myokines, skeletal muscle mass, muscle strength
Citation: Lee JH and Jun H-S (2019) Role of Myokines in Regulating Skeletal Muscle Mass and Function. Front. Physiol. 10:42. doi: 10.3389/fphys.2019.00042
Received: 05 November 2018; Accepted: 14 January 2019;
Published: 30 January 2019.
Edited by:
Marilia Seelaender, University of São Paulo, BrazilReviewed by:
Kunihiro Sakuma, Tokyo Institute of Technology, JapanNicolas J. Pillon, Karolinska Institute (KI), Sweden
Copyright © 2019 Lee and Jun. This is an open-access article distributed under the terms of the Creative Commons Attribution License (CC BY). The use, distribution or reproduction in other forums is permitted, provided the original author(s) and the copyright owner(s) are credited and that the original publication in this journal is cited, in accordance with accepted academic practice. No use, distribution or reproduction is permitted which does not comply with these terms.
*Correspondence: Jong Han Lee, jhleecw@gachon.ac.kr Hee-Sook Jun, hsjun@gachon.ac.kr