- 1CNRS and Sorbonne University, UMR7144 Adaptation and Diversity in Marine Environment (AD2M) Laboratory, Ecology of Marine Plankton Team, Station Biologique de Roscoff, Roscoff, France
- 2Department of Ecological Chemistry, Alfred-Wegener-Institute Helmholtz Centre for Polar and Marine Research, Bremerhaven, Germany
- 3Faculty of Biology/Chemistry, University of Bremen, Bremen, Germany
Mixotrophy, i.e., the capability of both phototrophy and phagotrophy within a single organism, is a prominent trophic mode in aquatic ecosystems. Mixotrophic strategies can be highly advantageous when feeding or photosynthesis alone does not sustain metabolic needs. In the current review, we discuss the functional types of mixotrophic marine protists (herein mixoplankton) within the context of evolution. Permanent plastids have been established in large due to gene transfer from prey and/or endosymbionts to the host cell. In some kleptoplastidic mixoplankton, prior gene transfers and active transcription of plastid related genes in the host can help maintain and extend retention of the current kleptoplast. In addition to kleptoplasts, the prey nucleus is also sometimes retained and actively transcribed to help maintain and even replicate the kleptoplasts. Endosymbiotic relations vary considerably in the extent to which hosts affect symbionts. For example, some endosymbionts are heavily modified to increase photosynthetic efficiency, or are controlled in their cell division. It can be proposed that many kleptoplasts and endosymbionts are in fact en route to becoming permanent plastids. Conditions such as increased temperature and limiting nutrients seem to favor phagotrophy in mixoplankton. However, responses of mixoplankton to changing environmental conditions like light irradiance, temperature, nutrient, and prey availability are variable and species-specific. Studying mixotrophs with temporary plastids could elucidate past and future evolutionary mechanisms and dynamics of processes such as phagotrophy and the establishment of (secondary) permanent plastids.
Introduction to Mixotrophy
All living organisms need resources (micronutrients and macronutrients) in order to sustain their structure, basic cellular functions, and their overall existence. Various strategies (e.g., phototrophy, phagotrophy, chemotrophy, or osmostrophy) have evolved in order to acquire these important resources. The two most well-known strategies for nutrient acquisition distinguish organisms into two functional categories, those that make use of light to fix carbon (phototrophs/primary producers) and those that feed on others (heterotrophs/consumers). However, there is a third category to consider – mixotrophs. As indicated by the name, mixotrophy refers to a mixed trophic mode, thereby combining both phototrophic and heterotrophic modes of nutrition in order to fulfill cellular nutrient requirements.
We focus here on marine mixotrophic protists, but mixotrophy is an important trait for organisms both on land, and in water (Selosse et al., 2017). A well-known land example being the carnivorous plants that feed on insects. In aquatic ecosystems, mixotrophy is much more prevalent and widespread than initially thought. It can be found in a plethora of different organisms, from unicellular eukaryotes to multicellular metazoa such as jellyfishes or sea slugs that use endosymbionts or acquired plastids for photosynthesis (Cruz et al., 2013; Selosse et al., 2017). Plankton research has traditionally been based on the division of plankton between photosynthetic phytoplankton and heterotrophic zooplankton. The increasing focus on mixotrophy has changed the perception of plankton dynamics and interactions within plankton communities (Flynn et al., 2013). As more examples of mixotrophic marine organisms became known, it was realized that mixotrophy is not a rare occurrence in aquatic ecosystems, but fairly common (Mitra et al., 2014; Caron, 2017).
Mixoplankton
In the last decades, mixotrophic protists were referred to using definitions combining the two contradicting terms of phytoplankton and phagotrophy. The term mixotroph was used for photosynthetic organisms that take up dissolved organic carbon by osmotrophy, as well as for those using phagotrophy (Burkholder et al., 2008; Sforza et al., 2012). An emerging need to formally define mixotrophic protists (Flynn et al., 2013) with regards to their nutritional mode led to the first efforts to categorize mixotrophs in groups with distinct features. In an attempt to group protists based on their nutritional mode and function, Mitra et al. (2016) proposed a comprehensive terminology. Following the definitions of functional groups for mixotrophic protists, Flynn et al. (2019) suggested the use of the term mixoplankton – “planktonic protists that express, or have potential to express, phototrophy and phagotrophy” – as is the nomenclature we herein follow.
The functional classification of mixoplankton is based on how the cell incorporates photosynthesis. Mixotrophic protists (i.e., mixoplankton), as defined by Mitra et al. (2016) and Flynn et al. (2019), are functionally distinguished between constitutive mixoplankton (CM) and non-constitutive mixoplankton (NCM). A CM has an inherent capability for both phototrophy and phagotrophy. Constitutive mixoplankton are found in most eukaryotic microalgal lineages (e.g., green algae, euglenophytes, cryptophytes, chrysophytes, haptophytes, and dinoflagellates; Figure 1). Non-constitutive mixoplankton, which are defined by the need to acquire their photosynthetic ability through external means, are found mostly among ciliates, dinoflagellates, Foraminifera, and Radiolaria (Figure 1). Phototrophic activity in NCM can broadly be achieved in three ways which further divide NCM into sub-groups: (1) stealing chloroplasts of (any) prey (generalist non-constitutive mixoplankton, GNCM); (2) stealing chloroplasts from specific prey (plastidic specialist non-constitutive mixoplankton pSNCM); and (3) harboring photosynthetically active endosymbionts (endosymbiotic specialist non-constitutive mixoplankton, eSNCM; acquired phototrophy reviewed in Stoecker et al., 2009). Even though, we currently make a functional separation between GNCM and pSNCM, it is possible that some pSNCM lean more toward GNCM. Often very few data are available to support the GNCM assumption, making GNCM appear as pSNCM. The main differences between GNCM and pSNCM definitions rest simply on the rate of success in utilizing plastids from prey, and on the observed specificity of the prey from which plastids can be acquired.
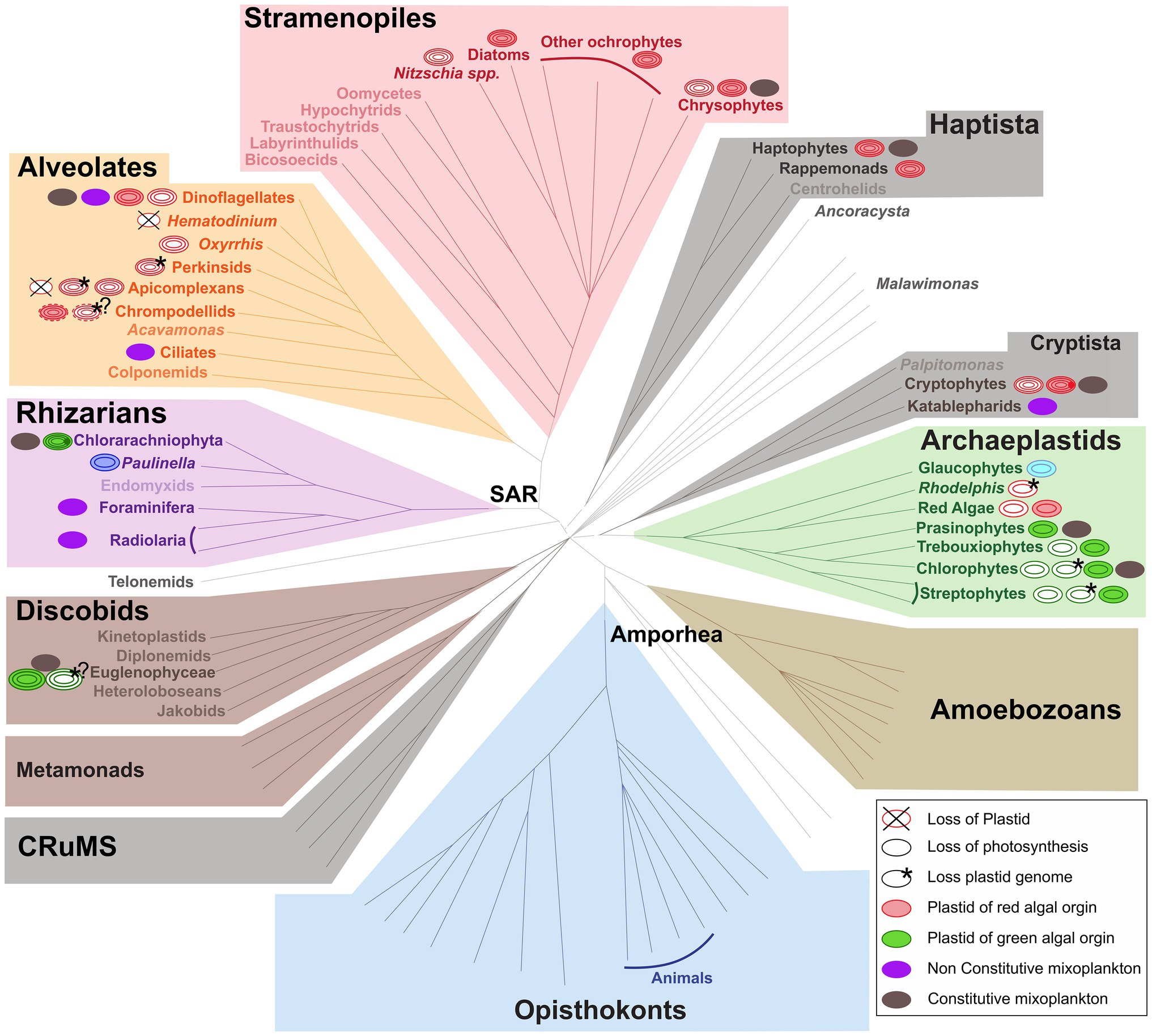
Figure 1. Distribution of (non-)photosynthetic plastids and mixoplankton among eukaryotes. The schematic representation of the eukaryotic tree of life shows the distribution of plastids and protist mixoplankton among major taxonomic lineages. The tree topology is based on (Keeling and Burki, 2019). Dashed gray lines of certain deep branches indicate yet unclear relationships. Mixoplankton indicated with a purple non-constitutive mixoplankton (NCM) or dark gray constitutive mixoplankton (CM) circle is attributed if at least one member of the lineage can be indicated as such, this does not exclude that other taxa in the lineage use trophic modes such as purely heterotrophic (phagotrophic) or phototrophic. The focus here is on mixoplankton, hence kleptoplasty and photosymbiosis in animals is not indicated. Plastids and their origins are indicated by a circle, primary plastids are bounded by two membranes, while secondary, tertiary, or alternatively quaternary plastids (complex plastids) are bounded either by three or four membranes as schematically shown by the number of circular lines. Non-photosynthetic plastids are shown in white, and the number and color of circular lines corresponds to the photosynthetic plastids from which they were derived. The primary plastids, arising from a single primary endosymbiosis, of Archaeplastida including glaucophytes (bright blue), red algae (red), green algae, and land plants (green) are bounded by two membranes (two circular lines). The plastid of Paulinella chromatophora (Rhizaria) represents an example of another independent primary endosymbiosis, and is also depicted bound by two membranes (dark blue). Complex plastids of red algal origin can be found in Stramenopiles (plastids bounded by four membranes), Alveolata (plastids bounded by three or four membranes), haptophytes and cryptophytes (both containing plastids bounded by four membranes). Both photosynthetic and non-photosynthetic plastids can be found among Stramenopiles, dinoflagellates, chrompodellids, and cryptophytes. Plastids present in Apicomplexa and perkinsid taxa, as well as Oxhhrris are all non-photosynthetic (uncertain number of membranes). Chloraracniophyta (Rhizaria; plastids bounded by four membranes) and Euglenophyceae (Discobits, Excavata; plastids bounded by three membranes) arose via two independent secondary endosymbioses of green algae. Evidence for the loss of photosynthesis and retention of non-photosynthetic plastids exists among Euglenophyceae (white circle, three green circular lines). Nucleomorphs, leftover nuclei of endosymbionts, are present between the second and the third plastid membranes of Chlorarachniophyta (dark green dot) and cryptophytes (dark red dot). Complete plastid loss (crossed circles) is found in some Apicomplexa and dinoflagellates, while genome-less non-photosynthetic plastids occur among perkinsids, and Rhodelphis (asterisk) and likely also among chrompodellids and Euglenophyceae (asterisks with question marks). The outermost fourth membrane of chrompodellids is indicated by a dashed circular line, because it is yet uncertain if colpodellids have three of four membranes. Figure modified from Keeling and Burki (2019) with permission from Elsevier. Plastid details are mainly derived from Hadariová et al. (2018) and Sibbald and Archibald (2020), see also Supplementary Table 1.
Similar to the distinction of GNCM and pSNCM, the classification of mixoplankton into functional types is greatly influenced by current knowledge or lack thereof. Often the metabolic contribution of predation vs. photosynthesis is poorly understood (Anschütz and Flynn, 2020). By default, an organism would be either phototroph or heterotroph, while to identify a mixotroph both phagotrophic and phototrophic capabilities need to have been identified (Beisner et al., 2019; Hansen et al., 2019; Ferreira and Calbet, 2020). Considering the relative ease to identify a photosynthetic organism (by fluorescence signal of plastid pigments, and/or optical and electron microscopy to characterize the cell) it is tempting to immediately classify it as a (pure) phototroph (Anderson et al., 2017; Beisner et al., 2019). In contrast, phagotrophy rarely has clear external identifiers, instead it requires meticulous experimentation to identify phagotrophic potential in an organism (Anderson et al., 2017; Beisner et al., 2019). Even then, the absence of observed phagotrophy might just imply that not all conditions were met–time, type or state of prey, or state of the potential predator. Transcriptomics and genomics-based approaches and subsequent gene-based predictive models can help indicate mixotrophic potential (Burns et al., 2018). Identification of genetic potential for both phototrophy and phagotrophy does not forego the need for physiological experimentation and knowledge of photosynthetic and feeding rates. When the potential for a nutritional mode is observed, one could expect this potential to be used or otherwise lost over evolutionary time. Though, a nutritional mode might only be used under certain conditions. An organism could rely on (yet unobserved) instances of e.g., phagotrophy in space or time, which can be exceedingly difficult confirm.
The different functional groups of mixoplankton possess traits, such as phagotrophy and acquired photosynthesis that play a major part in evolutionary processes. Studying mixotrophy in its different forms offers an opportunity to gain a better understanding of major ecological and evolutionary processes that have been crucial in the creation of life as we know it today. Mixotrophy has not only been pivotal in the past with visible effects still identifiable today (such as the establishment of permanent organelles through endosymbiosis), but also it is still a prevalent biological phenomenon with a considerable fraction of marine plankton identified as mixoplankton (Flynn et al., 2019; Schneider et al., 2020; Figure 1). In mixotrophic organisms, we may have a glimpse of how life used to look in the past, and how things could change in the future. In the following sections, we address evolutionary theories and their relation to different functional types of mixoplankton, to gain a general overview of the possible role of mixotrophy as a transient state between stable evolutionary states. Given the driving force of a changing environment in protist evolution, we further discuss environmental factors that have an impact on mixoplankton and could explain their current prevalence and success.
Evolution of Plastids in Relation to Mixotrophy
Eukaryotic life as we know it today is very likely only possible due to phagocytosis (Yutin et al., 2009). Many eukaryotic cells still possess the mechanisms for phagocytosis, that is, the engulfment and internalization of particles with a diameter bigger than 0.4 μm (Haas, 2007). Phagocytosis facilitated the evolution of permanent plastids. However, how and when phagocytosis first evolved remains a major question in evolutionary biology (as discussed in Mills, 2020) and many theories have been proposed about explaining its connection to eukaryogenesis and organellogenesis (Sagan, 1967; Martin and Müller, 1998; Cavalier-Smith, 2002; Lane, 2005; Koonin, 2010; Martijn and Ettena, 2013; Booth and Doolittle, 2015; Hampl et al., 2019). These theories are mainly orientated toward the evolution of mitochondria and will not be treated in further detail here. Phylogenetic analyses have shown the high diversity of the molecular systems involved in phagocytosis and indicate that from early phagocytosis-like engulfment modern-type phagocytosis has independently evolved within many lineages (Yutin et al., 2009). Regardless of how and when phagocytosis first appeared, it is a trait retained by a considerable portion of marine unicellular life and plays a crucial role in ecosystem dynamics (without it, there would be no microbial loop).
The primary source of photosynthesis in eukaryotes is generally agreed upon to be established by the uptake and permanent retention of a photosynthetic cyanobacterium by a eukaryote – “primary endosymbiosis” (Schimper, 1883; Mereschkowsky, 1905; Cavalier-Smith, 1987; Zimorski et al., 2014). In essence, this resulted in the first mixotroph, capable of ingestion and photosynthesis. This primary endosymbiosis gave rise to Archaeplastida, and subsequent evolution of the plastids found in both plants and algae (with exception of the recent primary plastid acquisition of Paulinella, Box 1; Figure 1; Rodríguez-Ezpeleta et al., 2005; Adl et al., 2012; Jackson and Reyes-Prieto, 2014). For discussions on the monophyly, or polyphyly of Archaeplastida, see e.g., Larkum et al., 2007; Howe et al., 2008; Kim and Maruyama, 2014. Subsequent events of secondary and tertiary symbioses (uptake of eukaryotic alga with a primary plastid and secondary plastid, respectively) in eukaryotic algae have spread chloroplasts throughout the (eukaryotic) tree of life (see also Keeling, 2013 and references therein). To this day, it remains unresolved how many independent secondary and tertiary endosymbiotic events have occurred (Sibbald and Archibald, 2020).
BOX 1 Paulinella.
The case of the photosynthetic euglyphid testate amoeba Paulinella chromatophora (Cercozoa, Rhizaria) is an interesting one. First discovered in 1894 by Robert Lauterborn, P. chromatophora was found to have one or two kidney-shaped intracellular symbionts per cell, and unlike its sister species does not seem to feed on cyanobacteria but relies solely on photosynthesis (Nowack et al., 2008). The symbionts, now called chromatophores or cyanelles, were found to be related to Synechoccous/Prochlorococcus and function as “normal” plastids (Kies and Kremer, 1979; Marin et al., 2005; Yoon et al., 2006). Furthermore, the cyanelles have a significantly reduced genome, though less than canonical plastids, and also divide synchronously with the host (Kies and Kremer, 1979; Marin et al., 2005; Yoon et al., 2006). Besides the phylogenetic origin, the different origin of cyanelles is further supported by the distinct protein targeting mechanisms used by cyanelles and plastids of Archeaeplastida (Marin et al., 2005; Nowack and Grossman, 2012; Nowack, 2014). With evidence of endosymbiont gene transfer (EGT) and protein trafficking, the cyanelle can be considered an organelle, though at a more recent evolutionary stage than canonical plastids, due to its relatively large genome and the thick wall still surrounding it (Nakayama and Ishida, 2009; Nowack, 2014). Paulinella chromatophora is now considered the most recent and only known case of independent primary plastid acquisition, other than the Archaeplastida plastids, making it a model organism for understanding the evolution of primary endosymbiotic events.
Endosymbiont Gene Transfer for the Establishment of Permanent Plastids
Endosymbiosis has indisputably played a critical role in the evolution of eukaryotes and cellular plastids (reviewed in Keeling, 2013). However, the distinction between endosymbionts and permanent plastids or organelles has become constantly more blurred (Theissen and Martin, 2006; Keeling et al., 2015). In the current review, endosymbionts are considered “organisms non-permanently and autonomously living within their host – they keep their organellar integrity and are spatially separated from the host.” The symbiosis can be beneficial for both host and symbiont, and the endosymbionts are often still viable outside of their host. To be able to discuss endosymbionts in the context of mixoplankton classifications and ecology this definition of an endosymbiont should be considered. The reader, though, needs to be aware that the difference between an endosymbiont and a permanent organelle is all but clear-cut – with various examples discovered that bridge somewhere between the two concepts.
In the evolutionary context, a permanent plastid is the result of endosymbiosis and is stably maintained in the host organism over long periods of evolutionary time. A permanent plastid is not established simply through the engulfment of another alga. For the establishment of permanent plastids (organellogenesis), it is considered a prerequisite that genes for plastid functioning are transferred (from the endosymbiont) and integrated into the host nucleus, conjointly with a reduction of the endosymbiont plastid genome, and the establishment of a protein-targeting system to move nuclear-encoded proteins into the plastid (Larkum et al., 2007; Zimorski et al., 2014; Archibald, 2015). The transfer of genes from the endosymbiont to the host nucleus is known as EGT (Timmis et al., 2004). EGT allows the establishment of a genetic connection between the functions of host and symbiont. Before plastid retention, maintenance, and continuity are achieved EGT or gene loss can total up to 90% of the endosymbiont genome (Archibald, 2015; Qiu et al., 2017). In effect, most of the transferred genes are not involved in processes associated with photosynthesis or plastid maintenance, but in other essential biosynthetic pathways. Loss of such genes creates increased metabolic integration in the host.
Why EGT to the Hosts’ Nuclear Genome?
Incorporation of genes into the nuclear genome could impart the selective advantage of decreased mutation by moving the genetic material away from the reactive oxygen species producing plastids (Allen and Raven, 1996). For organellar genes, which are present in relatively small copy numbers, detrimental mutations would spread quickly. Nuclear genes have the advantage of sexual recombination, which could aid in the repair of mutations (whereas there are no repair mechanisms in organelles) and improve fitness in ever-changing environments. The advantage of less genetic material at the plastid site is proposed to further enhance the plastids metabolic efficiency by limiting the volume occupied by DNA and ribosomes (Cavalier-Smith, 1987; Timmis et al., 2004).
Why Keep Genes in the Plastid?
A hypothesis proposed by Allen (1993), known as the co-location (of gene and gene product) for redox reaction (CoRR) hypothesis, attempts to explain why any genes are left in the plastids at all. The underlying concept behind this hypothesis is that core regulatory plastid genes have an advantage by remaining in the plastid genome. In prokaryotes, which are the origin of plastids, the redox state can regulate gene expression. The close spatial contact of genes and their product in the same intercellular compartment permits immediate feedback and regulation after a change in redox state in the light reaction centers of chloroplasts, or the mitochondrial oxidative phosphorylation system (Allen, 2017). Indeed, genes found in the organelles are essential for the proper functioning of the electron transport chain of the photosynthetic reaction centers of chloroplasts or the oxidative phosphorylation pathway in mitochondria (Pfannschmidt et al., 1999; Allen and Martin, 2016; Allen, 2017). For example, as shown in extracted chloroplasts, in vitro, there is direct and rapid redox control of chloroplast transcription at the plastoquinone site, more rapid than possible by comparable nuclear genes (Pfannschmidt et al., 1999). Björkholm et al. (2015) further proposed that selective pressure on highly hydrophobic membrane proteins caused these genes to remain in plastid genomes.
How Are Imported Gene Products Targeted to Their Destination?
Crucial for the establishment of permanent plastids is the targeting of nuclear-encoded genes toward the plastid. von Heijne (1986) hypothesized that the hydrophobicity of nuclear precursor products would pose issues in endo-cellular protein transfer among cellular components. Due to hydrophobicity, protein targeting to the plastid could be misdirected toward the wrong cellular compartment (i.e., the endoplasmic reticulum; Björkholm et al., 2015). As a result, transport of proteins would be the main obstacle for effective EGT. Yet, many examples exist of nuclear-encoded hydrophobic proteins directed to the plastids. Proteins of the light-harvesting chlorophyll b or fucoxanthin chlorophyll a/c are all hydrophobic and need to move across several membranes before reaching the thylakoids (Allen and Raven, 1996). In effect, it is not only that genes need to be transferred to the host nucleus, but also maybe more importantly, transporter and protein-targeting systems need to be established. Arguably, this is the most complex and critical step toward the establishment of permanent plastids (Cavalier-Smith, 1999; Bodył et al., 2009).
Briefly, in early evolution, protein targeting could have been achieved by early forms of the complex TIC and TOC plastid translocons of the inner and outer chloroplast membranes, respectively (Bodył et al., 2009). The subunits of TIC-TOC translocons suggest a diverse origin, with genes involved from both prokaryotic (mainly cyanobacterial) and eukaryotic origin (reviewed in Reumann et al., 2005). Further targeting mechanisms involve the transit peptides on most nucleus-encoded proteins, which serve as a signal for import to a specific cellular component. Proteins can be targeted to, and recognized by, the plastid before being post-translationally imported through the TIC-TOC translocons. Additionally, this allows import through an endomembrane system involving the endoplasmic reticulum and Golgi system, further facilitating flexibility to target multiple cellular locations (Bodył et al., 2009). Whether the initial system and driver of plastid evolution revolved around a TIC-TOC-like system or an endomembrane system is not generally agreed upon (Cavalier-Smith, 2006; Bhattacharya et al., 2007; Bodył et al., 2009).
What Are the Actual Origins of Imported Nuclear Genes?
Recently, the interestingly coined “shopping bag model” hypothesized that nuclear genes for plastid proteins encompass varying phylogenetic origins, and builds on the fact that protists readily take up cytosolic DNA into their nucleus (Larkum et al., 2007; Howe et al., 2008). This DNA can remain for significant periods of time. DNA from lysed plastids will thus not unlikely result in integrations of plastid DNA into the nuclear genome. Before the final establishment of a permanent plastid, there could thus have been genetic contributions of many different origins over time. Transient relations like kleptoplasty, where a plastid is only temporarily retained (more in section “Temporary plastids: On their way to becoming permanent?”), could result in DNA entering the host nucleus. The kleptoplastic dinoflagellate Dinophysis, or likewise the Ross Sea dinoflagellate, which itself does not harbor a permanent plastid, does harbor genes of diverse phylogenetic origin encoding for plastid-related proteins (Hehenberger et al., 2019; Hongo et al., 2019).
If a stable plastid were to be established, the genetics underlying the plastid machinery would finally be a mixture of the current stably established plastid and from previously acquired DNA. Cases of such chimeric genomes can be found in a great diversity of taxa (Dorrell et al., 2017). Certain dinoflagellates, for example, express isoforms for plastid-targeted proteins from several different phylogenetic origins, proteins such as cysteine synthase and psbU (Karlodinium, Patron et al., 2006), or isoprenoid and heem biosynthesis (Dinophysis, Hongo et al., 2019). Likewise, for the diatom genome, Morozov and Galachyants (2019), and earlier cases discussed in Dagan and Martin (2009) and Deschamps and Moreira (2012), illustrated supposed ancient gene transfers from green algae, in addition to genetic information from the current red algal plastid.
Eukaryotic cells readily take up DNA into the nucleus. Obviously, though, not all foreign DNA is integrated, nor do all endosymbionts become permanent plastids. The conversion of an endosymbiont to permanent organelle is a long evolutionary process that requires specific and complex protein transport mechanisms. The simple transfer of genes is not enough. The apparent complexity of the needed protein transport mechanisms makes it that plastids are less likely to become permanent and be sustained, than is endosymbiosis or temporal plastid retention in the host.
Secondary Loss of Trophic Functions
Photosynthesis
The acquisition of photosynthetic ability is considered to be well established in (plankton) evolution, yet, it is by no means always permanent (Cavalier-Smith, 1987). Secondary reduction of photosynthetic plastids has been recorded many times. When heterotrophy is retained, phototrophy may not be essential to a cell’s survival anymore. Consequently, the loss of a plastid can occur when the ecological situation changes and the functions of the plastid are no longer entirely needed, or even potentially detrimental (Williams and Keeling, 2003; Burki, 2016). Transcriptomic surveys of heterotrophic and mixotrophic chrysophytes have revealed genetic adaptations to a gradual loss of chloroplasts (Beisser et al., 2017; Graupner et al., 2018). The gene expression in these cases tends to be downregulated for photosynthesis-related processes. The gradual reduction of plastids, both in genome and size, leads to the formation of non-photosynthetic structures that could be described as “cryptic” plastids (reviewed in Hadariová et al., 2018).
Non-photosynthetic plastids are not uncommon and have been recorded across the eukaryotic tree, from Alveolata and Stramenophiles to cryptophytes, Euglenopycae, red and green algae, as well as (parasitic) land plants (Hadariová et al., 2018; Figure 1). For example, several diatoms, mostly of the genus Nitzschia, are apochlorotic, that is with non-photosynthetic plastids (Li and Volcani, 1987). Though the plastids are genomically reduced and no longer photosynthetically active, transcriptomics show that the plastids still play an indispensable role in among others glycolysis and gluconeogenesis (Kamikawa et al., 2017). The reduced chloroplasts–the apicoplasts–of plasmodium parasites no longer function for photosynthesis but carry out other biosynthetic processes for which the cell is dependent on the plastids (McFadden and Yeh, 2017; Janouskovec et al., 2019). Likewise, the recently described phagotrophic genus Rhodelphis was found to still host cryptic plastids involved in heem synthesis (Gawryluk et al., 2019). Here, the involvement in the heem synthesis pathway is likely the reason the plastids have not been entirely lost.
Even though reduction of plastids has been reported in many taxa, the complete loss of a plastid seems more difficult due to the importance of the plastid in many biochemical/metabolic pathways (Barbrook et al., 2006). Though it is rare, cases of complete plastid loss have been suggested for taxa belonging to oomycetes, ciliates, and dinoflagellates (Saldarriaga et al., 2001; Archibald, 2008; Reyes-Prieto et al., 2008). In the case of ciliates, the presence of proteins of plastid-origin suggests gene transfer from either algal prey or a putative photosynthetic history; however, in most cases evidence of a plastid harboring ancestor is not clear (Reyes-Prieto et al., 2008). Complete plastid loss is only unambiguously defined for several apicomplexan group parasites, e.g., Cryptosporidium and Hematodinium (Abrahamsen et al., 2004; Figure 1). While apicomplexans are evolutionarily descended from a mixotroph through the primary endosymbiotic event, the current parasitic live style could have been a major cause for the loss of dependency on the photosynthetic functions of the plastid (Archibald, 2015). Hematodinium, and also Amoebophrya, independently retain limited functions from their ancestral plastidic pathways, but as they live and fulfill their metabolic needs from their hosts the plastid would be redundant and is lost entirely (Gornik et al., 2015; John et al., 2019). Interestingly, free-living organisms tend to retain their plastid, possibly indicating that replacing lost metabolic pathways is more challenging (Janouskovec et al., 2017). Some cases are also known for dinoflagellates where, over evolutionary time, plastid loss is followed by the replacement with another (Keeling, 2013). This shows the relevant adaptability and evolutionary transitions between nutritional modes as a response to changing environmental factors.
Phagocytosis
In essence, all eukaryotic microalgal lineages (e.g., cryptophytes, haptophytes, Euglenophyceae, Stramenopiles, or dinoflagellates) have the capacity of predation, though it may have been lost in species of each lineage. Phagocytosis was lost on multiple occasions, proposed as owing to the lack of need for this mode of nutritional uptake, i.e., when photosynthetic plastids were acquired. Indeed, extant red algae or glaucophyptes harboring primary plastids, as well as diatoms, have lost their phagotrophic ability (Raven et al., 2009). Phagocytosis is most often kept in those taxa harboring secondary or tertiary plastids – many of which are mixoplankton. The fact that it is specifically those species with secondary and tertiary plastid that retain phagotrophy has been hypothesized to be an artifact of time (Kim and Maruyama, 2014). That is to say, these secondary plastids species are younger and current selective pressures keep these species mixotrophic, whereas this might not have been the case after the establishment of primary plastids.
Temporary Plastids: On Their Way to Becoming Permanent?
“The mitochondria and plastids we see today may, accordingly, have only been the luckiest of a longstanding series of doomed endosymbionts who were saved by transfer of genes to the nucleus.” – Keeling et al. (2015)
GNCM and pSNCM: Stolen Plastids
Unlike a permanent plastid, a stolen plastid or kleptoplast is transient. Kleptoplasty refers to the acquisition of solely the plastid from a plastid-containing prey. Kleptoplasty is a relatively common phenomenon that has been observed in various protists taxa including dinoflagellates, ciliates, and Foraminifera (Stoecker et al., 2009; Pillet and Pawlowski, 2013; Park et al., 2014). An exceptional case of animal kleptoplasty is of sacoglossan sea slugs (Pierce et al., 2003) and some flatworms (Van Steenkiste et al., 2019). The critical aspect of the kleptoplasty definition is that plastids remain only transiently functional within the kleptoplastic host. Depending on the taxa, the stolen plastids exhibit a wide range of origins and varying retention times. Retention time can be from days to months, after which the photosynthetic activity is lost and/or the plastid digested. Both the ability of the host to control the upkeep of the plastid, and the inherent stability of the original plastid can affect the retention time (Green et al., 2005). Even though kleptoplasts can have a positive impact on the energy budget of the host, there are also “side effects” due to the functioning of kleptoplasts. The host has to deal with the stress invoked due to the generation of reactive oxygen species as well as maintain the plastid with the perspective of assuring its functionality (Uzuka et al., 2019).
Studies of the Dinophysis-Mesodinium-Cryptophyta complex have contributed substantially to our understanding of the dynamics of kleptoplasty and the route toward a potential tertiary plastid establishment. Kleptoplastidic Mesodinium sp. (ciliate) does not only steal the plastid (kleptoplasts) from its cryptophyte prey but also the nucleus (kleptokaryon; Hansen et al., 2013, 2016). The kleptoplastids significantly contribute to the energetic budget of the cell with a large proportion of the total carbon needs (Hansen et al., 2013). The ability to retain fully functional plastids for a long period of time is in large part due to the additional retention of the prey nucleus (Johnson et al., 2007; Kim et al., 2017). The kleptokaryon becomes enlarged, and recent advances in transcriptomic and genomic data from both the ciliate and cryptophyte suggest that the sequestered nucleus is transcriptionally active and could account for approximately half of the total transcriptome of the ciliate (Altenburger et al., 2020). The kleptokaryon expresses genes responsible for both the maintenance of chloroplasts and the biosynthesis of metabolites for which Mesodinium lacks the genetic toolkit (Lasek-Nesselquist et al., 2015; Kim et al., 2016). This allows Mesodinium to replicate and exploit the plastids for a few months before being degraded (Smith and Hansen, 2007; Hansen et al., 2013). Interestingly, no photosynthesis-related genes were found to be transcribed by the genome of the ciliate (Altenburger et al., 2020). Instead, differential gene expression analysis indicates Mesodinium alters the gene expression of the kleptokaryon compared to when the nucleus is in its original host (Lasek-Nesselquist et al., 2015; Kim et al., 2016).
Kleptoplasts and kleptokaryon usage have also been observed in the (freshwater) dinoflagellate Nusuttodinium aeruginosum (Onuma and Horiguchi, 2015). The nucleus of cryptomonad origin undergoes extensive remodeling, accompanied by the enlargement of the kleptoplast (Onuma et al., 2020). The remodeling includes polyploidization, upregulated gene expression of pathways that involve among others, metabolism, gene translation, and DNA replication, as well as downregulation of certain genes like those involved in motility. In both Mesodinium rubrum and Nusuttodinium aeroginosum the transcriptional regulation of the kleptokaryon is no longer affected by light-regime. These transcriptional changes, and especially polyploidization, are common ground among kleptoplastic organisms and are also seen in permanently established plastids (Bendich, 1987). This suggests that polyploidization and transcriptional regulation could be a prerequisite in plastid evolution and prospective organelles. These modifications are also observed in the permanent diatom endosymbiont of Durinskia baltica – made possible by its polyploidy – the diatom nucleus can undergo karyostenosis during cell division (Tippit and Pickett Heaps, 1976; Yamada et al., 2019).
Dinophysis (Dinoflagellata) are known to sequester plastids of cryptophyte origin, but not the nucleus (Park et al., 2014). The difference to other kleptoplastidic organisms is that in this case the acquisition is indirect and solely after the consumption of Mesodinium sp. (note that Mesodinium sp. itself has a kleptoplast; Park et al., 2014; Hansen et al., 2016). Several Dinophysis nuclear transcripts are involved in photosynthesis-related processes including plastid maintenance or pigment biosynthesis (Hongo et al., 2019). Even though the acquired plastids originate from a cryptophyte, genes involved in their maintenance originate from: haptophytes, dinoflagellates, chlorarachniophytes, cyanobacteria, and cryptophytes (Hongo et al., 2019). This indicates the complexity of gene transfer to the nuclear genome of the host and gives an idea about their evolutionary past, and previous interactions, and also advocates for the “shopping bag” hypothesis (see section “Endosymbiont gene transfer for the establishment of permanent plastids”). The aforementioned NCM highlight different methods for the control of acquired plastids. The first one involves the acquisition of the prey nucleus, which is actively expressed and allows extended use and even replication of the kleptoplasts, without EGT of plastid related genes (the case of Mesodinium). The second strategy does involve EGT. Host-encoded genes facilitate extended use of the plastid, and possible plastid division, instead of a kleptokaryon (the case of Dinophysis; Rusterholz et al., 2017). The incapability to enslave the nucleus of the prey could have resulted in stronger selective forces for EGT. Yet, both strategies ultimately yield an increased survival in absence of food (Hansen et al., 2013), and could potentially evolve host-governed organelles.
A recent study by Hehenberger et al. (2019) has provided substantial insights into the fine lines between temporary and permanent plastids. The kleptoplastic Antarctic Ross Sea dinoflagellate belongs to the Kareniaceae lineage, but unlike its sister taxa, it does not have permanent plastids (Gast et al., 2007). The unusual long retention (up to 22 months) of the kleptoplast in the host suggests the presence of genes to stabilize the plastid (Sellers et al., 2014). Transcriptomic analysis of the Ross Sea dinoflagellate revealed host-encoded genes for kleptoplast-targeted proteins (Hehenberger et al., 2019). This includes photosynthesis related genes that are also found in permanent plastids harboring relatives of the Ross Sea dinoflagellate. Interestingly, such plastid-targeted genes appear to come from various phylogenetic sources, the majority of which do not share origin with the kleptoplast, indicating ancestral establishment of plastid-targeting mechanisms. The early establishment of such mechanisms would facilitate the further usage and possible integration of subsequent plastids (of different origin), such as the haptophyte kleptoplast of the Ross Sea dinoflagellate. A critical factor for the permanent integration of the kleptoplast, as indicated in Hehenberger et al. (2019), is the level of transcriptional regulation of the host on plastid-targeted nuclear genes. The Ross Sea dinoflagellate only has transcriptional regulation for a subset of plastid-related genes, as depicted by the response (or lack thereof) to different light and temperature conditions. Indications of the establishment of transcriptional regulation mechanisms, which are important for the control of modern-day plastids, would suggest progress toward permanent plastid integration.
eSNCM: Plastids Through Photosymbiosis
The internalization of a phototroph by a heterotrophic organism characterizes one of the first steps toward a permanent plastid (section “Endosymbiont gene transfer for the establishment of permanent plastids”). Many extant examples exist of such endosymbioses, without per se being permanent plastids (reviewed in Stoecker et al., 2009). Upon entering a host cell an endosymbiont is encapsulated in a vacuole-like unit called a symbiosome. Endosymbionts can (most of the time) divide within the host, foregoing the need for continuous replenishment of photosymbionts. Well-known endosymbioses are cnidarians, like corals, with dinoflagellate endosymbionts. Other (photo)endosymbioses include among others: marine Rhizaria, bivalves, and gastropods with dinoflagellate symbionts; Acantharia with haptophytes and a dinoflagellate (Noctiluca scintillans) with green prasinophyte algal symbionts (Pedimonas noctilucae; Anderson, 2012; Decelle et al., 2015; Clavijo et al., 2018).
In a photosymbiosis (or other endosymbiosis), where the symbiont provides energy or photosynthates, a prerequisite for a stable relation is that the interaction is beneficial to the host. The host must be dependent on the symbiont, otherwise, the symbiont would be expendable and the relation forfeited. The relation would thus naturally be driven toward exploitation of the symbiont by the host (Keeling and McCutcheon, 2017). For the symbiosis to be evolutionarily viable to the endosymbiont it needs to be able to reproduce itself. Indeed, many photosymbionts have been extracted and cultured from their host systems, illustrating that they are capable of independent existence and reproduction (Trench and Thinh, 1995; Probert et al., 2014). However, this is not always the case, thus creating an evolutionary dead-end for the symbiont.
In the Acantharia-Phaeocystis symbiosis, the Acantharia host cell recruits environmental Phaeocystis as endosymbionts. The acquisition of symbionts is suspected to be purely horizontal, and symbionts need to be reacquired for each individual. The symbionts in hospite are modified in several physiological aspects, among which are an increased number and volume of plastids, and more dense thylakoids, thereby increasing the photosynthetic efficiency (Decelle et al., 2019). The symbiosome of mature symbionts intrudes into the symbiont cell, further indicating a permanent change of the endosymbiont (Uwizeye et al., 2020). These modifications are thought to be a major reason as to why the symbionts are not viable after extraction from the host. The modifications made to the symbiont in this symbiosis show several similarities to the plastid acquisition of Paulinella, possibly representing steps toward more complete plastid integration. Genomic or transcriptomic studies of this symbiosis are still lacking; hence it remains unknown if there have been EGT events. Evidence of EGT could show further integration and hijacking of the functions of the symbiont besides the already observed modifications.
In endosymbiotic dinotoms – dinoflagellates in symbiosis with diatoms – like other endosymbioses, the symbiotic diatom is surrounded by a membrane, a symbiosome that separates it spatially from the cytosol of the host (Tomas et al., 1973; Jeffrey and Vesk, 1976). It should be noted that in this endosymbiosis, the diatom is not fully intact as it loses its silica frustule during uptake, and unlike a permanent plastid, genome reduction and EGT are lacking, and major cellular components of the diatom remain (Hehenberger et al., 2016; Yamada et al., 2019). The symbionts of endosymbiotic dinotoms, e.g., Durinksia kwazulunatalensis, D. baltica, and Glenodinium foliaceum, are structurally and transcriptionally autonomous from the host dinoflagellate and can replicate independently, yet DNA replication of the diatom endosymbiont seems to coincide closely with the cell division of the host (Figueroa et al., 2009; Yamada et al., 2019). This allows permanent retention of the diatom symbiont and vertical symbiont transfer. In contrast, in the kleptoplastidic dinotom Durinskia capensis, an internalized diatom gradually loses most of its organelles leaving only the plastids until those are also degraded (Yamada et al., 2019). The diatoms cannot be permanently retained as no nucleus control mechanisms are developed in the host, thus converting them into temporary kleptoplasts that will need to be reobtained in subsequent generations. The synchronized karyokinesis of host and endosymbiont, as in D. baltica or D. kwazulunatalensis, seems critical for the development of a more stable relationship between host and endosymbiont.
NCM as a Transition to Permanent Plastids
Bodył (2018) hypothesized that red algal derived secondary plastids, like those of dinoflagellates, evolved not necessarily by phagocytotic uptake, but via kleptoplastidy. In fact, it can be proposed that many of the previously explored kleptoplastidic and endosymbiotic relations are in effect new plastids en route to becoming permanent plastids, a “work in progress” situation. The establishment of permanent plastids is preceded by genomic reduction, and increased control of the host on its symbiont to the point that the symbiont is no longer able to reproduce in hospite nor ex hospite once released from the host. Progressively there would be increased genetic integration (EGT) of photosynthetic genes from the transient symbiont/kleptoplast associations (see targeting-ratchet model in Keeling, 2013). Dinophysis and Mesodinium both use a kleptoplast obtained from the same source, though EGT has yet only occurred in Dinophysis. Apart from EGT, the Ross Sea dinoflagellate exemplifies the importance of establishing transcriptional regulation mechanisms for transferred genes. The acantharian symbiont Phaeocytis has already been suggested to possibly be a form of kleptoplasty instead of symbiosis, due to the restrictions in proliferation and modifications on the symbiont (Decelle et al., 2012, 2019). Similar modifications are observed for the kleptoplast of Nusuttodinium. Dinotoms have varying levels of endosymbiont integration and karyokinesis in concert with their own (Yamada et al., 2019). Considering the insights into gene transfer, metabolic connectivity and protein-targeting between plastid/endosymbiont and host, we could hypothesize that kleptoplastic or endosymbiotic mixotrophs are an evolutionary intermediate toward a permanent plastid (Figure 2; Keeling, 2013; Keeling and McCutcheon, 2017; Hehenberger et al., 2019). However, this is not to say that all these systems are heading in the same direction, but could offer a chance to compare potential stages of evolving systems.
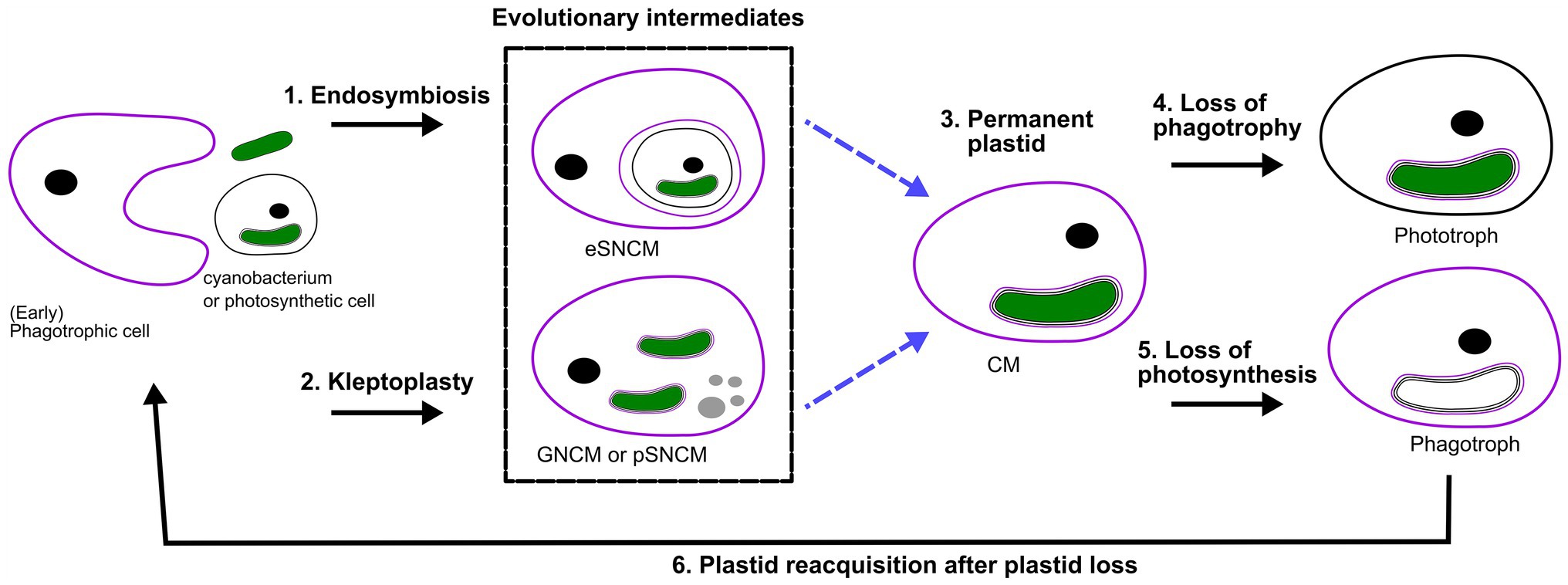
Figure 2. Perspective of protist plastid evolution and the role of mixotrophy. Conceptual illustration of plastid evolution depicting non-constitutive mixoplankton as hypothetical intermediate stages to permanent plastids. The cell nucleus is shown as a black circle, kleptokaryons as gray circles, and photosynthetic plastids in green. For the initial primary plastid acquisition, the plastid came from a cyanobacterium (green oval; see also “Box 1 Paulinella” for a possible exception), the acquired primary plastid would be bound by only two membranes as depicted in the photosynthetic cell on the left. While complex permanent plastids gained by secondary or tertiary endosymbiosis are bound by three or four membranes, respectively as illustrated by the number of lines around the plastid. In secondary and higher plastid acquisition, the photosynthetic cell can be either a CM or phototroph with primary, secondary or higher-order plastid. Illustrated here is secondary plastid acquisition with the host membrane in purple. Blue dashed lines are the hypothetical route toward permanent plastid retention. (1) Uptake of a photosynthetic alga and internalization of the entire organism as a symbiont (in a symbiosome) e.g., Radiolaria, Noctiluca. (2) Kleptoplasty shows the phagotrophic uptake of photosynthetic algae (prey), keeping the chloroplasts temporarily functional. The photosynthetic prey can be with primary, secondary (or higher) plastids. For example, the kleptoplastic ciliates like Strombidium sp. In some cases, the prey nucleus (gray circles) is also retained e.g., Mesodinium rubrum (which feeds on secondary plastid holding cryptophytes), and Nusuttodinium aeroginosum. (3) The establishment of permanent plastids. Primary plastids by uptake of a cyanobacterium, with the plastid bound by only two membranes, e.g., as retained in most Archaeplastida including glaucophytes, red and green algae, as well as land plants and the more recent primary plastid of Paulinella chromatophora. Secondary plastids bound by three or more membranes are found in Euglenophycaea, haptophytes, cryptophytes, and SAR organisms. Tertiary plastid acquisitions have only been clearly documented in Dinoflagellates. (4) Indicated here is the secondary loss of phagotrophy, we assume all pure phototrophs lost phagotrophy somewhere along their phylogeny e.g., diatoms. (5) Loss of photosynthesis, though only in rare cases is the plastid completely lost, the non-photosynthetic plastid is depicted lacking green color. Evidence for such primary plastid loss is found in e.g., Rhodelphis sp., Helicosporidium sp. Loss of photosynthesis after secondary (or higher) permanent plastid acquisition can be found, for example, in some Euglenophycaea, Stramenopiles like apochlorotic diatoms, dinoflagellates, cryptophytes, perkinsids, and Apicomplexa like Cryptosporidium. Complete plastid loss can, for example, be seen in Polytoma sp., Cryptosporidium, and Hematodinium (see also Figure 1). (6) Plastid reacquisition after loss of phototrophy is only known in dinoflagellates.
Alternatively, temporary plastids could be an advantageous adaptation as opposed to permanent organelles. Unlike genetic adaptation, and thus the establishment of permanent plastids, temporary plastids could allow for more plasticity within an environmental range. This could be thought of in light of Acantharia that acquire and maintain locally adapt Phaeocystis symbionts as directed by the environment (Decelle et al., 2012; Mars Brisbin et al., 2018). Corals can also shuffle their symbiont populations when stress events mandate it (Lewis and Coffroth, 2004; LaJeunesse et al., 2009, 2010). This allows the host to better adapt to changing environments by shuffling and swapping symbionts. Additionally, acquired plastids possibly impose less energy investment and could be converted into host biomass, whereas this is not possible for permanent plastids (Flynn and Hansen, 2013).
Why, When, and How to Mixotroph
Protists are often limited in their growth capabilities, pure phototrophs by nutrients or light, and pure phagotrophs by prey availability. This holds particularly true in oligotrophic ecosystems, where mixotrophy proved to be more beneficial (Stoecker et al., 2017). In such nutrient poor environments, mixotrophy can thus be especially widespread. Since mixoplankton can meet their metabolic demands through a mixture of photosynthesis and prey ingestion, they can supplement one nutrient source with another.
Trade-Offs
Even though mixotrophy offers flexibility in nutrient and energy acquisition, mixoplankton are not prevailing everywhere. Therefore, certain trade-offs (benefits and costs) to mixed nutrition seem apparent (Raven, 1997). Both phototrophy and phagotrophy involve mechanisms and structures that incur costs. In the case of a phototroph, this is primarily the maintenance of the photosynthetic machinery. For phagotrophs, it includes costs related to all the required mechanisms for the internalization of food particles and/or production of secondary metabolites, like toxins that mediate prey capture. Investments into the photosynthetic (or phagotrophic) machinery involve nutrient costs, estimated as up to 50% (or 10%) of the cell’s nutrient currency (e.g., carbon, nitrogen, and phosphorus; Raven, 1984, 1997). Resources used to maintain chloroplasts could have otherwise been used for other processes, like growth or reproduction. This is likewise the case for traits associated with, for example, phagotrophy, dissolved nutrient uptake, prey detection, capture or defense (Andersen et al., 2015). This inevitably has an impact on the growth rate, as “costs” are to be paid in order to make use of each of these processes. Costs that are also incurred by the fundamentals such as DNA, RNA, and ribosome maintenance and multiplication (Flynn and Mitra, 2009; Stoecker et al., 2009; Ward et al., 2011).
Besides the basic trade-offs in cell maintenance, more plastid-specific trade-offs have also been researched. Giovanardi et al. (2017) showed more tightly compressed thylakoids in plastids of mixotrophic Neochloris oleoabundans than in autotrophically grown samples, thereby the compressed thylakoids would lose on fluidity. It has been hypothesized that more appressed thylakoids would preserve or delay the degradation of the photosystems (Giovanardi et al., 2017). However, in turn, decreased fluidity could impair plasticity for stressors such as temperature change (Mansour et al., 2018).
Along with phototrophy come high-affinity nutrient transporters for the import of dissolved nutrients into the cell. An increase in nutrient uptake sites for dissolved nutrients, facilitates higher affinity and an increase in nutrient uptake. Simultaneously, these sites could also be costly due to being potential entry points for viruses (Menge and Weitz, 2009). From that perspective, a lower affinity to dissolved nutrients could mitigate viral entry. For mixotrophs, the loss of these sites and the involved costs would be minimized when nutrient uptake can be achieved and supplemented through phagotrophy. Predation, in turn, requires encounters with other cells, which could also incur indirect costs due to increased encounters with potential predators (Broglio et al., 2001; Kiørboe, 2011). Phagotrophy would further exclude a rigid cell wall and thereby limit protection, as in diatoms with rigid cell walls but having lost phagotrophic capability.
Mixotrophic Gradient Under Changing Biotic and Abiotic Factors
An increasing number of both field and laboratory studies have contributed to our understanding of the dynamics and different aspects of mixotrophy. A mixotrophic lifestyle is often flexible. The biological investment in phagotrophy or phototrophy can vary depending on the prevailing biotic and abiotic environmental conditions. The reliance on either photosynthesis or prey consumption can be partial or complete. We thus refer to a gradient of mixotrophy, toward either more phototrophy or phagotrophy, considering their relative contribution to an organism’s metabolic needs (Figure 3). This mixotrophic spectrum is further affected by the degree of investment into nutrient uptake (Andersen et al., 2015), to which previously discussed potential trade-offs apply. How mixotrophy and mixotrophic communities change in response to environmental changes is a current scientific challenge (González-Olalla et al., 2019). Environmental conditions discussed here include light irradiance, temperature, and nutrients in the perspective of feeding and growth.
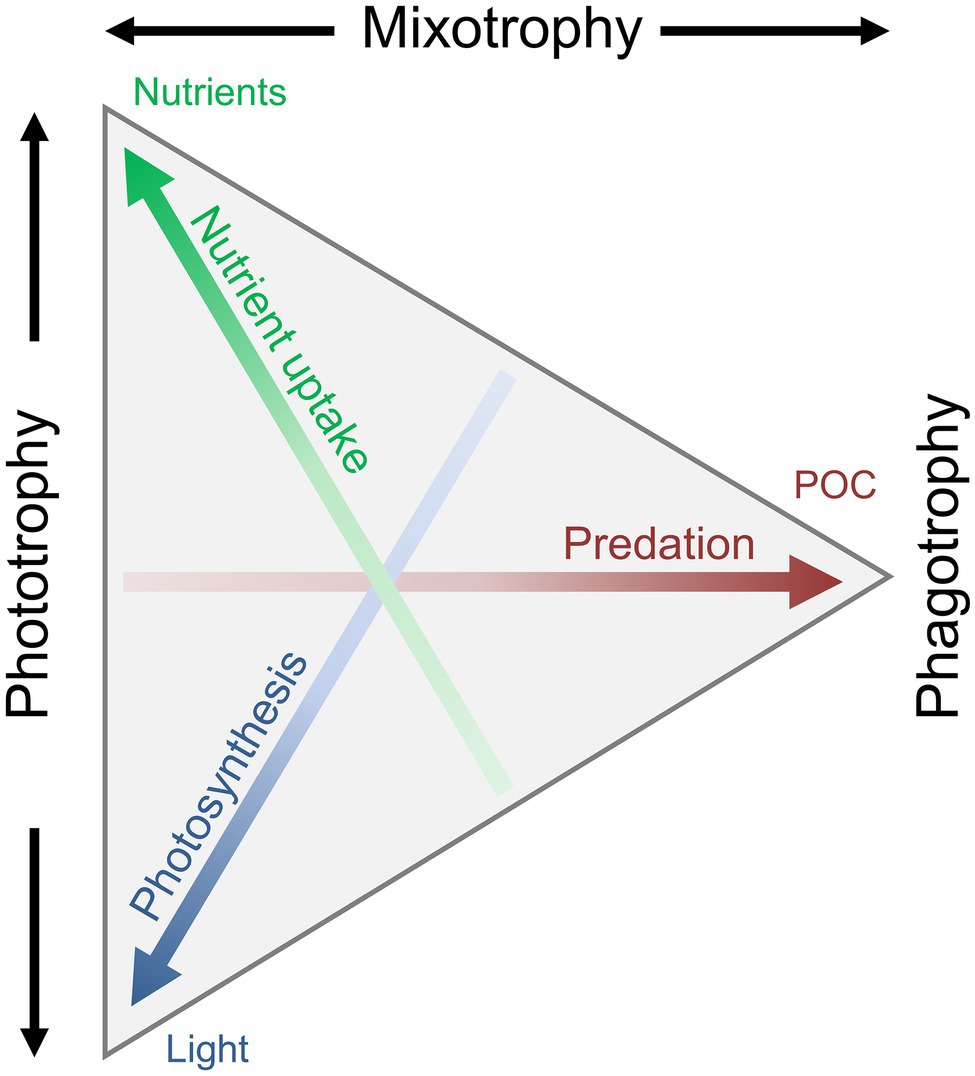
Figure 3. Conceptual illustration of the trophic continuum from phototrophy over mixotrophy to pure phagotrophy. The mixotrophic spectrum is defined by the allocation into three traits: nutrient uptake, photosynthesis, and predation/phagotrophy, leading to the uptake of dissolved inorganic nutrients, CO2, and particulate organic matter. A specific organisms’ trophic strategy is defined as a point within the triangle: an organism somewhere along the left side will be a pure phototroph, an organism at the right tip would be a pure heterotroph, and any organism somewhere between these two extremes is a mixotroph. Redrawn and modified from Andersen et al. (2015), with permission from Oxford University Press.
Light
The ability to photosynthesize prerequisites the availability of light in order to grow. Irradiance generally increases growth up to a point of saturation, though often the highest growth rates are achieved if prey is available (Li et al., 1999). There are, however, exceptions of mixoplankton that rely mainly on either photosynthesis (e.g., Gymnodinium resplendens; Skovgaard, 2000) or phagotrophic feeding (e.g., Fragilidium sp. or Ochromonas; Skovgaard, 1996; Flöder et al., 2006). In these cases, mixotrophy seems to be a survival strategy for when a single trophic mode does not suffice (Keller et al., 1994; Flöder et al., 2006; Anderson et al., 2018), for example, during long polar winters (Stoecker and Lavrentyev, 2018).
Under low light conditions, two different isolates of Ochromonas showed contrasting physiological responses. Whereas one isolate moved toward an increased focus on photosynthesis by compensating the lower light intensity with more cellular chlorophyll a, the other isolate substituted the focus on photosynthesis with an increased rate of bacterivory (Wilken et al., 2020), as is similarly seen for Heterocapsa rotundata (Millette et al., 2017). Increased irradiance has been shown to cause a positive feedback chain for Karlodinium veneficum, where higher light increased photosynthesis as well as feeding rate; the increased phagotrophy in turn increased photosynthetic capacity (Li et al., 1999). In contrast, the feeding rate of M. rubrum is not affected by irradiance (Smith and Hansen, 2007). Karlodinium veneficum, and some other dinoflagellates, cannot grow (or are only sustained) in the dark even when prey is available, that is to say, it is an obligate phototroph. Other dinoflagellate species, or chrysophytes such as Ochromonas sp., can grow in constant darkness if supplied with food (Caron et al., 1993; Ok et al., 2019). Thus, predation can, but does not always, compensate for the inability to use chloroplasts in the dark. Fischer et al. (2017) investigated the effect of light on the success of mixotrophic flagellates, and showed that mixotrophic bacterivorous flagellates prevail in high light and low nutrients conditions. Oppositely, pure heterotrophs and pure autotrophs prevail in low-light and nutrient-rich ecosystems.
Nutrients
Research has mostly focused on the availability of non-carbon elements such as nitrogen and phosphorus. When one or both of these nutrients are inadequate mixotrophy can be vital, as prey ingestion is the only alternative to obtain missing nutrients. Many examples are recorded of species feeding on either bacteria or eukaryotic prey under short- or long-term starvations of nitrogen and phosphorus. Nephroselmis pyriformis, for example, can achieve normal growth even when nutrient-limited by compensation via bacterivory (Anderson et al., 2018). Other seemingly non-phagotrophic species can be induced to perform phagotrophy by nutrient-limited treatments, e.g., in dinoflagellates (Smalley et al., 2003; Johnson, 2015), haptophytes (Chan et al., 2019), and cryptophytes (González-Olalla et al., 2019). The use of diluted media to provoke starvation indicates the complexity in understanding in detail the principal factors that could induce phagotrophy and identify mixotrophy.
Nutrients that are usually found in low concentrations, such as iron, micronutrients, and vitamins are mostly understudied (Anderson et al., 2018), but can have major effects on growth rates (Reich et al., 2020). It is believed that (N)CM obtain micro-nutrients mainly through the ingestion of prey (Hansen et al., 2019). However, there remains a lot to learn about nutrient acquisition dynamics in NCM, and the role of endosymbionts in acquiring dissolved nutrients.
Temperature
The general theory of metabolism in ecology suggests a shift to heterotrophy when temperature increases (Allen et al., 2005; Rose and Caron, 2007; Franzè and Lavrentyev, 2014). As in many biological systems, the relation of phagotrophy and temperature is Gaussian, and delimited by an organism’s temperature tolerance. Higher temperature implies higher metabolic rates and concomitantly an increased need for energy, and possible stress incurrence. Studies have indeed shown higher ingestion rates in response to temperature increase in many species (Princiotta et al., 2016; Cabrerizo et al., 2019; Ok et al., 2019). This validates the applicability of the general theory of metabolism in marine protists. A combined increase of phagotrophy and a decrease in photosynthetic capacity with higher temperatures strongly decreased the relative contribution of phototrophy in mixotrophic Ochromonas sp., allowing growth rates to keep increasing under temperatures where photosynthetic growth rate already declined (Wilken et al., 2013). This effect of temperature on the autotrophic, mixotrophic, and heterotrophic growth of Ochromonas sp. has highlighted the trade-offs in its trophic strategy and temperature tolerance. The increased temperature can be considered as a stressor that needs to be alleviated by reduced photosynthetic activity, and/or compensated for with nutrients from phagotrophy (Cabrerizo et al., 2019). This might be achieved by using nutrients from phagotrophy to repair or maintain photosystems.
Role of Mixotrophy in a Future Scenario
We found the physiological research on mixoplankton is generally biased toward CM. Yet, within this group there is already a large taxonomic and physiological diversity resulting in ambiguous responses to environmental factors. Especially among dinoflagellates – which can be CM, NCM, pure phagotrophs, or pure phototrophs – there is a wide spectrum of responses to food availability and abiotic conditions (Hansen, 2011). Efforts to understand the effects of varying conditions, such as nutrients, light irradiance, temperature, and prey availability have given diverse results, thus making it difficult to highlight general patterns.
Changing environmental conditions can shift mixoplankton toward a higher prevalence of either phototrophy or phagotrophy (Figure 4). Such shifts have apparent effects on the plankton community composition and the energy transfer between trophic levels (Rose and Caron, 2007; O’Connor et al., 2009). Though not unambiguous, the general trends seem to show mostly a phagotrophic increase with increased temperature. Decreased nutrients can induce or promote phagotrophy, oppositely, phototrophy seems preferred when sufficient nutrients are available. Whereas light is directly fueling phototrophy, it can promote both phototrophy and phagotrophy. In some cases, limiting inorganic nutrients is a critical factor for expressing mixotrophy, while in other cases mixotrophy is a de-facto state independent of nutrient regime (Johnson, 2015). The combination of low nutrients and high light is suggested to favor mixotrophy, as it is suboptimal for either pure phagotrophy or phototrophy (Mitra et al., 2014; Hansen et al., 2019). Seasonally such conditions can already show increased mixoplankton abundance, e.g., in summer (mature ecosystem states; Hansen et al., 2019). It has also been proposed that polar regions, characterized by extremes in solar irradiance and nutrient-limitation, select for mixotrophy (Stoecker and Lavrentyev, 2018). The grand écart hypothesis proposed by Selosse et al. (2017) further attempts to explain the ubiquity of mixotrophy. The open ocean ecosystem is usually limited in non-carbon elements, and summer stratification limits the recycling of sinking particles or nutrients back into the water column and upper layers. The biological carbon pump further exports carbon and other nutrients from the upper photic layers. This results in light being available in the upper layers, while other essential nutrients are separated toward deeper layers. This imbalance in resources (light and nutrients) favors and selects for mixotrophs that can supplement the lack of resources by feeding.
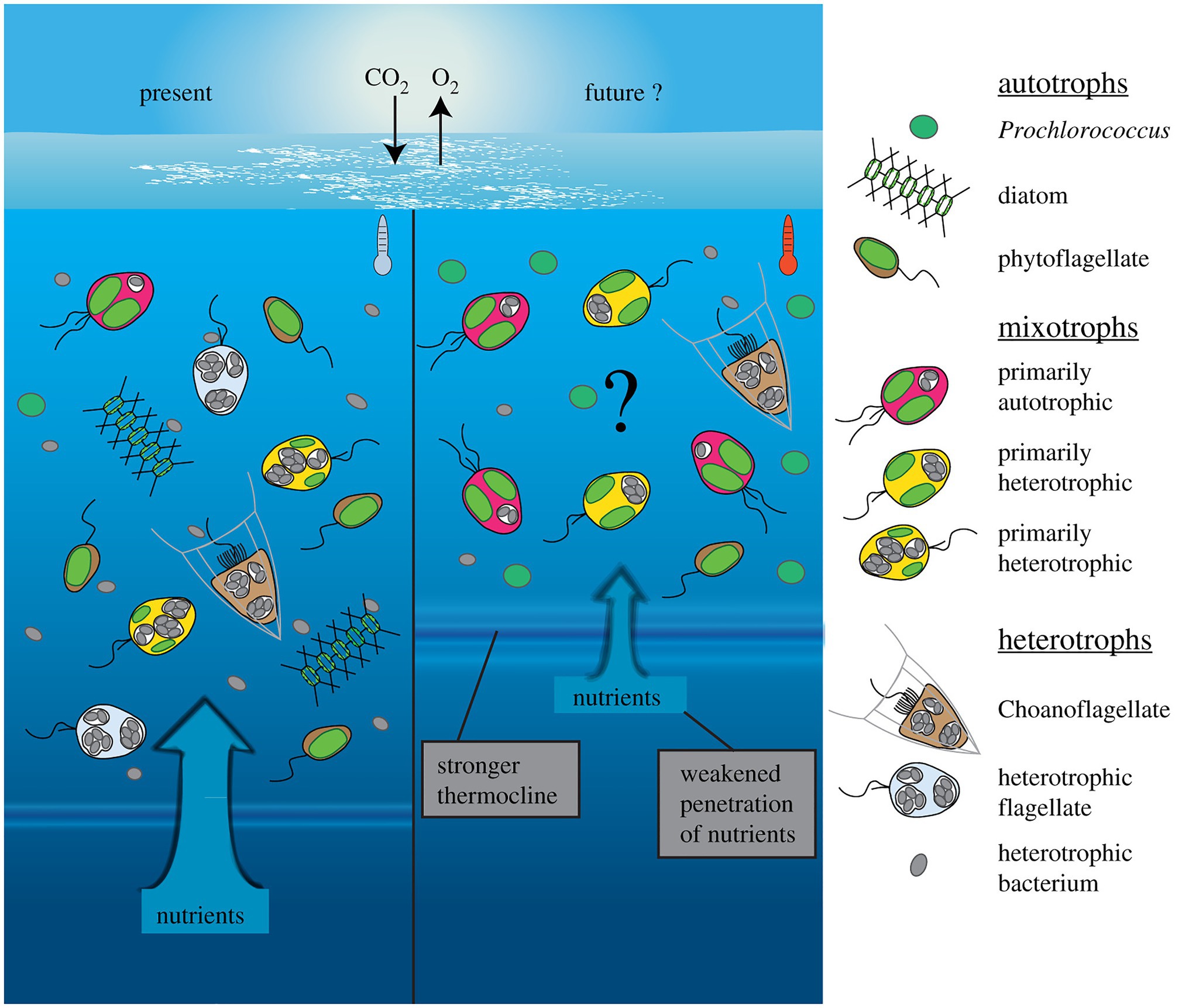
Figure 4. Conceptual depiction of plankton community shift under a climate change scenario in the open ocean. Future changes involve warmer, more oligotrophic water and higher light irradiance, due to stronger water stratification and a shallower mixed layer depth (right). Protists with photosynthetic ability contain plastids (green), while phagotrophic ability is indicated by food vacuoles containing bacterial prey (gray). The limiting nutrients in this future scenario are expected to favor more nutrient competitive species like Prochlorococcus (cyanobacteria), while no generalized effects can yet be accurately predicted for mixoplankton. Generally, the flexibility in nutritional modes by mixoplankton renders them more competitive than pure photototrophs or heterotrophs, thus a general increase in relative mixoplankton abundance could be expected. Species-specific responses could shift over the mixotrophic continuum either toward more heterotrophy (in red) or more photosynthesis (in yellow). Reprinted from Wilken et al. (2019), with permission from Royal Society.
Conclusion
Phagotrophy rests at the origin of the different functional types of mixoplankton. The complete digestion of prey, the retention of prey organelles, or whole cells as endosymbionts indicates the diversity of responses after phagocytosis of “external” material. Constitutive mixoplankton benefits from having alternative ways of nutrient acquisition that each can be exploited under certain conditions. In non-constitutive mixoplankton, undigested organelles can either be perceived as the inability of the host to degrade (or digest) the organelles (incidental retention), or as the active retention of them for further use (deliberate retention). The longevity of an organelle within the host as well as the level of usage, integration, and connectivity is species-specific and variable (Gast et al., 2007; Stoecker et al., 2009; Yamada et al., 2019). The broad diversity in exploitation and handling of acquired organelles, as well as the acquisition and/or loss of phagocytosis or photosynthesis, is indicative of the adaptation of organisms to environmental conditions over their evolutionary history.
Mixotrophs with temporary plastids could depict evolutionary intermediates of permanent plastids (Figure 2). In species with temporary plastids, evolutionary force could still drive toward fixing phototrophic potential. That is, of course, given that the trade-offs for having potential for both photosynthesis and phagotrophy do not pose too strong a counterforce. With ever-changing environmental conditions, even seasonally, temporary plastids can give more flexibility by allowing a change to better-adapted plastids and are thereby more beneficial. Conversely, when either organic matter or light is constantly available, maintaining phagotrophy as well as photosynthesis can be costly. When the costs for maintaining multiple structures do not offset the benefits, evolution might be driven toward permanent plastids in lieu of temporary plastids, or toward pure heterotrophy. It is thus interesting to note that mixoplankton have been associated with oligotrophic environments (Mitra et al., 2014; Hansen et al., 2019), as the imbalance in resource availability “forces” more efficient use and alternative means of resource acquisition.
So far, eco-physiological studies to understand the role of environmental conditions in the prevalence of mixotrophy are mostly done on cultures. Many taxa thus remain understudied due to difficulties in culturing them. This is especially true for fragile protists and grazers (often NCM), where the successful isolation of both the predator and the prey is required. Field experiments are thus especially needful as the effects of parameters such as light, temperature, prey, and nutrients availability can be tested on understudied uncultured taxa. New technologies including single-cell genomics (Labarre et al., 2020), transcriptomics (Cooney et al., 2020), and chemical imaging (Decelle et al., 2020) allow us to study the biological role of individual cells. The potential of such techniques could reinforce the study of rare and unculturable NCM taxa. Comparative genomics has already helped to better understand how temporary plastids become permanent (Sibbald and Archibald, 2020). Combined knowledge from comparative genomics and new eco-physiological studies on mixotrophs could give further insights in the evolutionary fate of extant temporary plastids and NCM, as well as resolve evolutionary histories and their early intermediates. Jointly, knowledge of the evolutionary fate of such trophic dynamics shaping traits will assist in predictions of oceanic ecosystems.
Author Contributions
All authors listed have made a substantial, direct and intellectual contribution to the work, and approved it for publication.
Funding
This work was supported by European Union’s Horizon 2020 research and innovation programme under grant agreement No. 766327.
Conflict of Interest
The authors declare that the research was conducted in the absence of any commercial or financial relationships that could be construed as a potential conflict of interest.
Acknowledgments
We thank the MixITiN consortium (www.mixotroph.org) for helpful comments and criticism on early versions of the manuscript, in particular Fabrice Not, Uwe John, Maira Maselli, Lisa Schneider, Claudia Traboni, Kevin Flynn, and Aditee Mitra. We also want to thank the editor and the reviewers of the manuscript for their constructive comments.
Supplementary Material
The Supplementary Material for this article can be found online at: https://www.frontiersin.org/articles/10.3389/fmars.2021.666160/full#supplementary-material
References
Abrahamsen, M. S., Templeton, T. J., Enomoto, S., Abrahante, J. E., Zhu, G., Lancto, C. A., et al. (2004). Complete genome sequence of the apicomplexan, Cryptosporidium parvum. Science 304, 441–445. doi: 10.1126/science.1094786
Adl, S. M., Simpson, A. G. B., Lane, C. E., Lukeš, J., Bass, D., Bowser, S. S., et al. (2012). The revised classification of eukaryotes. J. Eukaryot. Microbiol. 59, 429–493. doi: 10.1111/j.1550-7408.2012.00644.x
Allen, J. F. (1993). Control of gene expression by redox potential and the requirement for chloroplast and mitochondrial genomes. J. Theor. Biol. 165, 609–631. doi: 10.1006/jtbi.1993.1210
Allen, J. F. (2017). The CoRR hypothesis for genes in organelles. J. Theor. Biol. 434, 50–57. doi: 10.1016/j.jtbi.2017.04.008
Allen, A. P., Gillooly, J. F., and Brown, J. H. (2005). Linking the global carbon cycle to individual metabolism. Funct. Ecol. 19, 202–213. doi: 10.1111/j.1365-2435.2005.00952.x
Allen, J. F., and Martin, W. F. (2016). Why have organelles retained genomes? Cell Syst. 2, 70–72. doi: 10.1016/j.cels.2016.02.007
Allen, J. F., and Raven, J. A. (1996). Free-radical-induced mutation vs redox regulation: costs and benefits of genes in organelles. J. Mol. Evol. 42, 482–492. doi: 10.1007/BF02352278
Altenburger, A., Cai, H., Li, Q., Drumm, K., Kim, M., Zhu, Y., et al. (2020). Limits to the cellular control of sequestered cryptophyte prey in the marine ciliate Mesodinium rubrum. ISME J. 15, 1056–1072. doi: 10.1038/s41396-020-00830-9
Andersen, K. H., Aksnes, D. L., Berge, T., Fiksen, Ø., and Visser, A. (2015). Modelling emergent trophic strategies in plankton. J. Plankton Res. 37, 862–868. doi: 10.1093/plankt/fbv054
Anderson, O. R. (2012). Living together in the plankton: a survey of marine protist symbioses. Acta Protozool. 52, 1–10. doi: 10.4467/16890027AP.13.006.1085
Anderson, R., Charvet, S., and Hansen, P. (2018). Mixotrophy in chlorophytes and haptophytes—effect of irradiance, macronutrient, micronutrient and vitamin limitation. Front. Microbiol. 9:1704. doi: 10.3389/FMICB.2018.01704
Anderson, R., Jürgens, K., and Hansen, P. J. (2017). Mixotrophic phytoflagellate bacterivory field measurements strongly biased by standard approaches: a case study. Front. Microbiol. 8:1398. doi: 10.3389/fmicb.2017.01398
Anschütz, A. A., and Flynn, K. J. (2020). Niche separation between different functional types of mixoplankton: results from NPZ-style N-based model simulations. Mar. Biol. 167, 1–21. doi: 10.1007/s00227-019-3612-3
Archibald, J. M. (2008). Plastid evolution: remnant algal genes in ciliates. Curr. Biol. 18, R663–R665. doi: 10.1016/j.cub.2008.06.031
Archibald, J. M. (2015). Endosymbiosis and eukaryotic cell evolution. Curr. Biol. 25, R911–R921. doi: 10.1016/j.cub.2015.07.055
Barbrook, A. C., Howe, C. J., and Purton, S. (2006). Why are plastid genomes retained in non-photosynthetic organisms? Trends Plant Sci. 11, 101–108. doi: 10.1016/j.tplants.2005.12.004
Beisner, B. E., Grossart, H. P., and Gasol, J. M. (2019). A guide to methods for estimating phago-mixotrophy in nanophytoplankton. J. Plankton Res. 41, 77–89. doi: 10.1093/plankt/fbz008
Beisser, D., Graupner, N., Bock, C., Wodniok, S., Grossmann, L., Vos, M., et al. (2017). Comprehensive transcriptome analysis provides new insights into nutritional strategies and phylogenetic relationships of chrysophytes. PeerJ 5:e2832. doi: 10.7717/peerj.2832
Bendich, A. J. (1987). Why do chloroplasts and mitochondria contain so many copies of their genome? Bioessays 6, 279–282. doi: 10.1002/bies.950060608
Bhattacharya, D., Archibald, J. M., Weber, A. P. M., and Reyes-Prieto, A. (2007). How do endosymbionts become organelles? Understanding early events in plastid evolution. Bioessays 29, 1239–1246. doi: 10.1002/bies.20671
Björkholm, P., Harish, A., Hagström, E., Ernst, A. M., and Andersson, S. G. E. (2015). Mitochondrial genomes are retained by selective constraints on protein targeting. Proc. Natl. Acad. Sci. U. S. A. 112, 10154–10161. doi: 10.1073/pnas.1421372112
Bodył, A. (2018). Did some red alga-derived plastids evolve via kleptoplastidy? A hypothesis. Biol. Rev. Camb. Philos. Soc. 93, 201–222. doi: 10.1111/brv.12340
Bodył, A., Mackiewicz, P., and Stiller, J. W. (2009). Early steps in plastid evolution: current ideas and controversies. Bioessays 31, 1219–1232. doi: 10.1002/bies.200900073
Booth, A., and Doolittle, W. F. (2015). Eukaryogenesis, how special really? Proc. Natl. Acad. Sci. U. S. A. 112, 10278–10285. doi: 10.1073/pnas.1421376112
Brisbin, M. M., Mesrop, L. Y., Grossmann, M. M., and Mitarai, S. (2018). Intra-host symbiont diversity and extended symbiont maintenance in photosymbiotic Acantharea (clade F). Front. Microbiol. 9:1998. doi: 10.3389/fmicb.2018.01998
Broglio, E., Johansson, M., and Jonsson, P. R. (2001). Trophic interaction between copepods and ciliates: effects of prey swimming behavior on predation risk. Mar. Ecol. Prog. Ser. 220, 179–186. doi: 10.3354/meps220179
Burkholder, J. A. M., Glibert, P. M., and Skelton, H. M. (2008). Mixotrophy, a major mode of nutrition for harmful algal species in eutrophic waters. Harmful Algae 8, 77–93. doi: 10.1016/j.hal.2008.08.010
Burki, F. (2016). Mitochondrial evolution: going, going, gone. Curr. Biol. 26, R410–R412. doi: 10.1016/j.cub.2016.04.032
Burns, J. A., Pittis, A. A., and Kim, E. (2018). Gene-based predictive models of trophic modes suggest asgard archaea are not phagocytotic. Nat. Ecol. Evol. 2, 697–704. doi: 10.1038/s41559-018-0477-7
Cabrerizo, M. J., González-Olalla, J. M., Hinojosa-López, V. J., Peralta-Cornejo, F. J., and Carrillo, P. (2019). A shifting balance: responses of mixotrophic marine algae to cooling and warming under UVR. New Phytol. 221, 1317–1327. doi: 10.1111/nph.15470
Caron, D. A. (2017). Acknowledging and incorporating mixed nutrition into aquatic protistan ecology, finally. Environ. Microbiol. Rep. 9, 41–43. doi: 10.1111/1758-2229.12514
Caron, D. A., Sanders, R. W., Lim, E. L., Marrasé, C., Amaral, L. A., Whitney, S., et al. (1993). Light-dependent phagotrophy in the freshwater mixotrophic chrysophyte Dinobryon cylindricum. Microb. Ecol. 25, 93–111. doi: 10.1007/BF00182132
Cavalier-Smith, T. (1987). The simultaneous symbiotic origin of mitochondria, chloroplasts, and microbodies. Ann. N. Y. Acad. Sci. 503, 55–71. doi: 10.1111/j.1749-6632.1987.tb40597.x
Cavalier-Smith, T. (1999). Principles of protein and lipid targeting in secondary symbiogenesis: euglenoid, dinoflagellate, and sporozoan plastid origins and the eukaryote family tree. J. Eukaryot. Microbiol. 46, 347–366. doi: 10.1111/j.1550-7408.1999.tb04614.x
Cavalier-Smith, T. (2002). The phagotrophic origin of eukaryotes and phylogenetic classification on protozoa. Int. J. Syst. Evol. Microbiol. 52, 297–354. doi: 10.1099/00207713-52-2-297
Cavalier-Smith, T. (2006). Origin of mitochondria by intracellular enslavement of a photosynthetic purple bacterium. Proc. Biol. Sci. 273, 1943–1952. doi: 10.1098/rspb.2006.3531
Chan, Y. F., Chiang, K. P., Ku, Y., and Gong, G. C. (2019). Abiotic and biotic factors affecting the ingestion rates of mixotrophic nanoflagellates (Haptophyta). Microb. Ecol. 77, 607–615. doi: 10.1007/s00248-018-1249-2
Clavijo, J. M., Donath, A., Serôdio, J., and Christa, G. (2018). Polymorphic adaptations in metazoans to establish and maintain photosymbioses. Biol. Rev. Camb. Philos. Soc. 93, 2006–2020. doi: 10.1111/brv.12430
Cooney, E. C., Okamoto, N., Cho, A., Hehenberger, E., Richards, T. A., Santoro, A. E., et al. (2020). Single cell transcriptomics of Abedinium reveals a new early-branching dinoflagellate lineage. Genome Biol. Evol. 12, 2417–2428. doi: 10.1093/gbe/evaa196
Cruz, S., Calado, R., Serôdio, J., and Cartaxana, P. (2013). Crawling leaves: photosynthesis in sacoglossan sea slugs. J. Exp. Bot. 64, 3999–4009. doi: 10.1093/jxb/ert197
Dagan, T., and Martin, W. (2009). Seeing green and red in diatom genomes. Science 324, 1651–1652. doi: 10.1126/science.1175765
Decelle, J., Colin, S., and Foster, R. A. (2015). “Photosymbiosis in marine planktonic protists” in Marine Protists. eds. S. Ohtsuka, T. Suzaki, T. Horiguchi, N. Suzuki, and F. Not (Tokyo, Japan: Springer), 465–500.
Decelle, J., Probert, I., Bittner, L., Desdevises, Y., Colin, S., de Vargas, C., et al. (2012). An original mode of symbiosis in open ocean plankton. Proc. Natl. Acad. Sci. U. S. A. 109, 18000–18005. doi: 10.1073/pnas.1212303109
Decelle, J., Stryhanyuk, H., Gallet, B., Veronesi, G., Schmidt, M., Balzano, S., et al. (2019). Algal remodeling in a ubiquitous planktonic photosymbiosis. Curr. Biol. 29, 968–978. doi: 10.1016/j.cub.2019.01.073
Decelle, J., Veronesi, G., Gallet, B., Stryhanyuk, H., Benettoni, P., Schmidt, M., et al. (2020). Subcellular chemical imaging: new avenues in cell biology. Trends Cell Biol. 30, 173–188. doi: 10.1016/j.tcb.2019.12.007
Deschamps, P., and Moreira, D. (2012). Reevaluating the green contribution to diatom genomes. Genome Biol. Evol. 4, 683–688. doi: 10.1093/gbe/evs053
Dorrell, R. G., Gile, G., McCallum, G., Méheust, R., Bapteste, E. P., Klinger, C. M., et al. (2017). Chimeric origins of ochrophytes and haptophytes revealed through an ancient plastid proteome. elife 6:e23717. doi: 10.7554/eLife.23717
Ferreira, G. D., and Calbet, A. (2020). Caveats on the use of rotenone to estimate mixotrophic grazing in the oceans. Sci. Rep. 10:3899. doi: 10.1038/s41598-020-60764-2
Figueroa, R. I., Bravo, I., Fraga, S., Garcés, E., and Llaveria, G. (2009). The life history and cell cycle of Kryptoperidinium foliaceum, a dinoflagellate with two eukaryotic nuclei. Protist 160, 285–300. doi: 10.1016/j.protis.2008.12.003
Fischer, R., Giebel, H.-A., Hillebrand, H., and Ptacnik, R. (2017). Importance of mixotrophic bacterivory can be predicted by light and loss rates. Oikos 126, 713–722. doi: 10.1111/oik.03539
Flöder, S., Hansen, T., and Ptacnik, R. (2006). Energy-dependent bacterivory in Ochromonas minima—a strategy promoting the use of substitutable resources and survival at insufficient light supply. Protist 157, 291–302. doi: 10.1016/j.protis.2006.05.002
Flynn, K. J., and Hansen, P. J. (2013). Cutting the canopy to defeat the “selfish gene”; conflicting selection pressures for the integration of phototrophy in mixotrophic protists. Protist 164, 811–823. doi: 10.1016/j.protis.2013.09.002
Flynn, K. J., and Mitra, A. (2009). Building the “perfect beast”: modelling mixotrophic plankton. J. Plankton Res. 31, 965–992. doi: 10.1093/plankt/fbp044
Flynn, K. J., Mitra, A., Anestis, K., Anschütz, A. A., Calbet, A., Ferreira, G. D., et al. (2019). Mixotrophic protists and a new paradigm for marine ecology: where does plankton research go now? J. Plankton Res. 41, 375–391. doi: 10.1093/plankt/fbz026
Flynn, K. J., Stoecker, D. K., Mitra, A., Raven, J. A., Glibert, P. M., Hansen, P. J., et al. (2013). Misuse of the phytoplankton-zooplankton dichotomy: the need to assign organisms as mixotrophs within plankton functional types. J. Plankton Res. 35, 3–11. doi: 10.1093/plankt/fbs062
Franzè, G., and Lavrentyev, P. J. (2014). Microzooplankton growth rates examined across a temperature gradient in the Barents Sea. PLoS One 9:e86429. doi: 10.1371/journal.pone.0086429
Gast, R. J., Moran, D. M., Dennett, M. R., and Caron, D. A. (2007). Kleptoplasty in an antarctic dinoflagellate: caught in evolutionary transition? Environ. Microbiol. 9, 39–45. doi: 10.1111/j.1462-2920.2006.01109.x
Gawryluk, R. M. R., Tikhonenkov, D. V., Hehenberger, E., Husnik, F., Mylnikov, A. P., and Keeling, P. J. (2019). Non-photosynthetic predators are sister to red algae. Nature 572, 240–243. doi: 10.1038/s41586-019-1398-6
Giovanardi, M., Poggioli, M., Ferroni, L., Lespinasse, M., Baldisserotto, C., Aro, E. M., et al. (2017). Higher packing of thylakoid complexes ensures a preserved photosystem II activity in mixotrophic Neochloris oleoabundans. Algal Res. 25, 322–332. doi: 10.1016/j.algal.2017.05.020
González-Olalla, J. M., Medina-Sánchez, J. M., and Carrillo, P. (2019). Mixotrophic trade-off under warming and UVR in a marine and a freshwater alga. J. Phycol. 55, 1028–1040. doi: 10.1111/jpy.12865
Gornik, S. G., Cassin, A. M., MacRae, J. I., Ramaprasad, A., Rchiad, Z., McConville, M. J., et al. (2015). Endosymbiosis undone by stepwise elimination of the plastid in a parasitic dinoflagellate. Proc. Natl. Acad. Sci. U. S. A. 112, 5767–5772. doi: 10.1073/pnas.1423400112
Graupner, N., Jensen, M., Bock, C., Marks, S., Rahmann, S., Beisser, D., et al. (2018). Evolution of heterotrophy in chrysophytes as reflected by comparative transcriptomics. FEMS Microbiol. Ecol. 94, 1–11. doi: 10.1093/femsec/fiy039
Green, B. J., Fox, T. C., and Rumpho, M. E. (2005). Stability of isolated algal chloroplasts that participate in a unique mollusc/kleptoplast association. Symbiosis 40, 31–40.
Haas, A. (2007). The phagosome: compartment with a license to kill. Traffic 8, 311–330. doi: 10.1111/j.1600-0854.2006.00531.x
Hadariová, L., Vesteg, M., Hampl, V., and Krajčovič, J. (2018). Reductive evolution of chloroplasts in non-photosynthetic plants, algae and protists. Curr. Genet. 64, 365–387. doi: 10.1007/s00294-017-0761-0
Hampl, V., Čepička, I., and Eliáš, M. (2019). Was the mitochondrion necessary to start eukaryogenesis? Trends Microbiol. 27, 96–104. doi: 10.1016/j.tim.2018.10.005
Hansen, P. J. (2011). The role of photosynthesis and food uptake for the growth of marine mixotrophic dinoflagellates. J. Eukaryot. Microbiol. 58, 203–214. doi: 10.1111/j.1550-7408.2011.00537.x
Hansen, P. J., Anderson, R., Stoecker, D. K., Decelle, J., Altenburger, A., Blossom, H. E., et al. (2019). “Mixotrophy among freshwater and marine protists,” in Encyclopedia of Microbiology. ed. T. M. Schmidt (Amsterdam, Netherlands: Elsevier Ltd.), 199–210.
Hansen, P. J., Nielsen, L. T., Johnson, M., Berge, T., and Flynn, K. J. (2013). Acquired phototrophy in Mesodinium and Dinophysis—a review of cellular organization, prey selectivity, nutrient uptake and bioenergetics. Harmful Algae 28, 126–139. doi: 10.1016/j.hal.2013.06.004
Hansen, P. J., Ojamäe, K., Berge, T., Trampe, E. C. L., Nielsen, L. T., Lips, I., et al. (2016). Photoregulation in a kleptochloroplastidic dinoflagellate, Dinophysis acuta. Front. Microbiol. 7:785. doi: 10.3389/fmicb.2016.00785
Hehenberger, E., Burki, F., Kolisko, M., and Keeling, P. J. (2016). Functional relationship between a dinoflagellate host and its diatom endosymbiont. Mol. Biol. Evol. 33, 2376–2390. doi: 10.1093/molbev/msw109
Hehenberger, E., Gast, R. J., and Keeling, P. J. (2019). A kleptoplastidic dinoflagellate and the tipping point between transient and fully integrated plastid endosymbiosis. Proc. Natl. Acad. Sci. U. S. A. 116, 17934–17942. doi: 10.1073/pnas.1910121116
Hongo, Y., Yabuki, A., Fujikura, K., and Nagai, S. (2019). Genes functioned in kleptoplastids of Dinophysis are derived from haptophytes rather than from cryptophytes. Sci. Rep. 9, 1–11. doi: 10.1038/s41598-019-45326-5
Howe, C. J., Barbrook, A. C., Nisbet, R. E. R., Lockhart, P. J., and Larkum, A. W. D. (2008). The origin of plastids. Philos. Trans. R. Soc. B Biol. Sci. 363, 2675–2685. doi: 10.1098/rstb.2008.0050
Jackson, C. J., and Reyes-Prieto, A. (2014). The mitochondrial genomes of the glaucophytes gloeochaete wittrockiana and cyanoptyche gloeocystis: multilocus phylogenetics suggests amonophyletic archaeplastida. Genome Biol. Evol. 6, 2774–2785. doi: 10.1093/gbe/evu218
Janouskovec, J., Gavelis, G. S., Burki, F., Dinh, D., Bachvaroff, T. R., Gornik, S. G., et al. (2017). Major transitions in dinoflagellate evolution unveiled by phylotranscriptomics. Proc. Natl. Acad. Sci. U. S. A. 114, E171–E180. doi: 10.1073/pnas.1614842114
Janouskovec, J., Paskerova, G. G., Miroliubova, T. S., Mikhaiiov, K. V., Birley, T., Aieoshin, V. V., et al. (2019). Apicomplexan-like parasites are polyphyletic and widely but selectively dependent on cryptic plastid organelles. elife 8:e49662. doi: 10.7554/eLife.49662
Jeffrey, S. W., and Vesk, M. (1976). Further evidence for a membrane-bound endosymbiont within the dinoflagellate Peridinium foliaceum. J. Phycol. 12, 450–455. doi: 10.1111/j.1529-8817.1976.tb02872.x
John, U., Lu, Y., Wohlrab, S., Groth, M., Janouškovec, J., Kohli, G. S., et al. (2019). An aerobic eukaryotic parasite with functional mitochondria that likely lacks a mitochondrial genome. Sci. Adv. 5, 1–12. doi: 10.1126/sciadv.aav1110
Johnson, M. D. (2015). Inducible mixotrophy in the dinoflagellate Prorocentrum minimum. J. Eukaryot. Microbiol. 62, 431–443. doi: 10.1111/jeu.12198
Johnson, M. D., Oldach, D., Delwiche, C. F., and Stoecker, D. K. (2007). Retention of transcriptionally active cryptophyte nuclei by the ciliate Myrionecta rubra. Nature 445, 426–428. doi: 10.1038/nature05496
Kamikawa, R., Moog, D., Zauner, S., Tanifuji, G., Ishida, K. I., Miyashita, H., et al. (2017). A non-photosynthetic diatom reveals early steps of reductive evolution in plastids. Mol. Biol. Evol. 34, 2355–2366. doi: 10.1093/molbev/msx172
Keeling, P. J. (2013). The number, speed, and impact of plastid endosymbioses in eukaryotic evolution. Annu. Rev. Plant Biol. 64, 583–607. doi: 10.1146/annurev-arplant-050312-120144
Keeling, P. J., and Burki, F. (2019). Progress towards the tree of eukaryotes. Curr. Biol. 29, R808–R817. doi: 10.1016/j.cub.2019.07.031
Keeling, P. J., and McCutcheon, J. P. (2017). Endosymbiosis: the feeling is not mutual. J. Theor. Biol. 434, 75–79. doi: 10.1016/j.jtbi.2017.06.008
Keeling, P. J., McCutcheon, J. P., and Doolittle, W. F. (2015). Symbiosis becoming permanent: survival of the luckiest. Proc. Natl. Acad. Sci. U. S. A. 112, 10101–10103. doi: 10.1073/pnas.1513346112
Keller, M. D., Shapiro, L. P., Haugen, E. M., Cucci, T. L., Sherr, E. B., and Sherr, B. F. (1994). Phagotrophy of fluorescently labeled bacteria by an oceanic phytoplankter. Microb. Ecol. 28, 39–52. doi: 10.1007/BF00170246
Kies, L., and Kremer, B. P. (1979). Function of cyanelles in the thecamoeba Paulinella chromatophora. Naturwissenschaften 66, 578–579. doi: 10.1007/BF00368819
Kim, M., Drumm, K., Daugbjerg, N., and Hansen, P. J. (2017). Dynamics of sequestered cryptophyte nuclei in Mesodinium rubrum during starvation and refeeding. Front. Microbiol. 8:423. doi: 10.3389/fmicb.2017.00423
Kim, G. H., Han, J. H., Kim, B., Han, J. W., Nam, S. W., Shin, W., et al. (2016). Cryptophyte gene regulation in the kleptoplastidic, karyokleptic ciliate Mesodinium rubrum. Harmful Algae 52, 23–33. doi: 10.1016/j.hal.2015.12.004
Kim, E., and Maruyama, S. (2014). A contemplation on the secondary origin of green algal and plant plastids. Acta Soc. Bot. Pol. 83, 331–336. doi: 10.5586/asbp.2014.040
Kiørboe, T. (2011). How zooplankton feed: mechanisms, traits and trade-offs. Biol. Rev. 86, 311–339. doi: 10.1111/j.1469-185X.2010.00148.x
Koonin, E. V. (2010). The origin and early evolution of eukaryotes in the light of phylogenomics. Genome Biol. 11:209. doi: 10.1186/gb-2010-11-5-209
Labarre, A., Obiol, A., Wilken, S., Forn, I., and Massana, R. (2020). Expression of genes involved in phagocytosis in uncultured heterotrophic flagellates. Limnol. Oceanogr. 65, S149–S160. doi: 10.1002/lno.11379
LaJeunesse, T. C., Smith, R. T., Finney, J., and Oxenford, H. (2009). Outbreak and persistence of opportunistic symbiotic dinoflagellates during the 2005 Caribbean mass coral “bleaching” event. Proc. R. Soc. B Biol. Sci. 276, 4139–4148. doi: 10.1098/rspb.2009.1405
LaJeunesse, T. C., Smith, R., Walther, M., Pinzón, J., Pettay, D. T., McGinley, M., et al. (2010). Host-symbiont recombination versus natural selection in the response of coral-dinoflagellate symbioses to environmental disturbance. Proc. Biol. Sci. 277, 2925–2934. doi: 10.1098/rspb.2010.0385
Lane, N. (2005). Power, Sex, Suicide: Mitochondria and the Meaning of Life. Oxford, United Kingdom: Oxforsd University Press.
Larkum, A. W. D., Lockhart, P. J., and Howe, C. J. (2007). Shopping for plastids. Trends Plant Sci. 12, 189–195. doi: 10.1016/j.tplants.2007.03.011
Lasek-Nesselquist, E., Wisecaver, J. H., Hackett, J. D., and Johnson, M. D. (2015). Insights into transcriptional changes that accompany organelle sequestration from the stolen nucleus of Mesodinium rubrum. BMC Genomics 16:805. doi: 10.1186/s12864-015-2052-9
Lewis, C. L., and Coffroth, M. A. (2004). The acquisition of exogenous, algal symbionts by an octocorat after bleaching. Science 304, 1490–1492. doi: 10.1126/science.1097323
Li, A., Stoecker, D. K., and Adolf, J. E. (1999). Feeding, pigmentation, photosynthesis and growth of the mixotrophic dinoflagellate Gyrodinium galatheanum. Aquat. Microb. Ecol. 19, 163–176. doi: 10.3354/ame019163
Li, C. W., and Volcani, B. E. (1987). Four new apochlorotic diatoms. Br. Phycol. J. 22, 375–382. doi: 10.1080/00071618700650441
Mansour, J. S., Pollock, F. J., Díaz-Almeyda, E., Iglesias-Prieto, R., and Medina, M. (2018). Intra- and interspecific variation and phenotypic plasticity in thylakoid membrane properties across two Symbiodinium clades. Coral Reefs 37, 841–850. doi: 10.1007/s00338-018-1710-1
Marin, B., Nowack, E. C. M., and Melkonian, M. (2005). A plastid in the making: evidence for a second primary endosymbiosis. Protist 156, 425–432. doi: 10.1016/j.protis.2005.09.001
Martijn, J., and Ettena, T. J. G. (2013). From archaeon to eukaryote: the evolutionary dark ages of the eukaryotic cell. Biochem. Soc. Trans. 41, 451–457. doi: 10.1042/BST20120292
Martin, W., and Müller, M. (1998). The hydrogen hypothesis for the first eukaryote. Nature 392, 37–41. doi: 10.1038/32096
McFadden, G. I., and Yeh, E. (2017). The apicoplast: now you see it, now you don’t. Int. J. Parasitol. 47, 137–144. doi: 10.1016/j.ijpara.2016.08.005
Menge, D. N. L., and Weitz, J. S. (2009). Dangerous nutrients: evolution of phytoplankton resource uptake subject to virus attack. J. Theor. Biol. 257, 104–115. doi: 10.1016/j.jtbi.2008.10.032
Mereschkowsky, C. (1905). Über natur und ursprung der chromatophoren im pflanzenreiche. Biol. Cent. 25, 593–604. (English translation in Martin, W., Kowallik, K. V. (1999). Annotated English translation of Mereschkowsky’s 1905 paper `Uber Natur und Ursprung der Chromatophoren im Pflanzenreiche '. Eur. J. Phycol. 34, 287–295).
Millette, N. C., Pierson, J. J., Aceves, A., and Stoecker, D. K. (2017). Mixotrophy in Heterocapsa rotundata: a mechanism for dominating the winter phytoplankton. Limnol. Oceanogr. 62, 836–845. doi: 10.1002/lno.10470
Mills, D. B. (2020). The origin of phagocytosis in earth history. Interface Focus 10:20200019. doi: 10.1098/rsfs.2020.0019
Mitra, A., Flynn, K. J., Burkholder, J. M., Berge, T., Calbet, A., Raven, J. A., et al. (2014). The role of mixotrophic protists in the biological carbon pump. Biogeosciences 11, 995–1005. doi: 10.5194/bg-11-995-2014
Mitra, A., Flynn, K. J., Tillmann, U., Raven, J. A., Caron, D., Stoecker, D. K., et al. (2016). Defining planktonic protist functional groups on mechanisms for energy and nutrient acquisition: incorporation of diverse mixotrophic strategies. Protist 167, 106–120. doi: 10.1016/j.protis.2016.01.003
Morozov, A. A., and Galachyants, Y. P. (2019). Diatom genes originating from red and green algae: implications for the secondary endosymbiosis models. Mar. Genomics 45, 72–78. doi: 10.1016/j.margen.2019.02.003
Nakayama, T., and Ishida, K. I. (2009). Another acquisition of a primary photosynthetic organelle is underway in Paulinella chromatophora. Curr. Biol. 19, R284–R285. doi: 10.1016/j.cub.2009.02.043
Nowack, E. C. M. (2014). Paulinella chromatophora—rethinking the transition from endosymbiont to organelle. Acta Soc. Bot. Pol. 83, 387–397. doi: 10.5586/asbp.2014.049
Nowack, E. C. M., and Grossman, A. R. (2012). Trafficking of protein into the recently established photosynthetic organelles of Paulinella chromatophora. Proc. Natl. Acad. Sci. U. S. A. 109, 5340–5345. doi: 10.1073/pnas.1118800109
Nowack, E. C. M., Melkonian, M., and Glöckner, G. (2008). Chromatophore genome sequence of Paulinella sheds light on acquisition of photosynthesis by eukaryotes. Curr. Biol. 18, 410–418. doi: 10.1016/j.cub.2008.02.051
O’Connor, M. I., Piehler, M. F., Leech, D. M., Anton, A., and Bruno, J. F. (2009). Warming and resource availability shift food web structure and metabolism. PLoS Biol. 7:e1000178. doi: 10.1371/journal.pbio.1000178
Ok, J. H., Jeong, H. J., Lim, A. S., You, J. H., Kang, H. C., Kim, S. J., et al. (2019). Effects of light and temperature on the growth of Takayama helix (Dinophyceae): mixotrophy as a survival strategy against photoinhibition. J. Phycol. 55, 1181–1195. doi: 10.1111/jpy.12907
Onuma, R., Hirooka, S., Kanesaki, Y., Fujiwara, T., Yoshikawa, H., and Miyagishima, S. (2020). Changes in the transcriptome, ploidy, and optimal light intensity of a cryptomonad upon integration into a kleptoplastic dinoflagellate. ISME J. 14, 2407–2423. doi: 10.1038/s41396-020-0693-4
Onuma, R., and Horiguchi, T. (2015). Kleptochloroplast enlargement, karyoklepty and the distribution of the cryptomonad nucleus in Nusuttodinium (= Gymnodinium) aeruginosum (Dinophyceae). Protist 166, 177–195. doi: 10.1016/j.protis.2015.01.004
Park, M. G., Kim, M., and Kim, S. (2014). The acquisition of plastids/phototrophy in heterotrophic dinoflagellates. Acta Protozool. 53, 39–50. doi: 10.4467/16890027AP.14.005.1442
Patron, N. J., Waller, R. F., and Keeling, P. J. (2006). A tertiary plastid uses genes from two endosymbionts. J. Mol. Biol. 357, 1373–1382. doi: 10.1016/j.jmb.2006.01.084
Pfannschmidt, T., Nilsson, A., and Allen, J. F. (1999). Photosynthetic control of chloroplast gene expression. Nature 397, 625–628. doi: 10.1038/17624
Pierce, S. K., Massey, S. E., Hanten, J. J., and Curtis, N. E. (2003). Horizontal transfer of functional nuclear genes between multicellular organisms. Biol. Bull. 204, 237–240. doi: 10.2307/1543594
Pillet, L., and Pawlowski, J. (2013). Transcriptome analysis of foraminiferan Elphidium margaritaceum questions the role of gene transfer in kleptoplastidy. Mol. Biol. Evol. 30, 66–69. doi: 10.1093/molbev/mss226
Princiotta, S. D. V., Smith, B. T., and Sanders, R. W. (2016). Temperature-dependent phagotrophy and phototrophy in a mixotrophic chrysophyte. J. Phycol. 52, 432–440. doi: 10.1111/jpy.12405
Probert, I., Siano, R., Poirier, C., Decelle, J., Biard, T., Tuji, A., et al. (2014). Brandtodinium gen. nov. and B.nutricula comb. Nov. (Dinophyceae), a dinoflagellate commonly found in symbiosis with polycystine radiolarians. J. Phycol. 50, 388–399. doi: 10.1111/jpy.12174
Qiu, H., Lee, J. M., Yoon, H. S., and Bhattacharya, D. (2017). Hypothesis: gene-rich plastid genomes in red algae may be an outcome of nuclear genome reduction. J. Phycol. 53, 715–719. doi: 10.1111/jpy.12514
Raven, J. A. (1984). A cost-benefit analysis of photon absorption by photosynthetic unicells. New Phytol. 98, 593–625. doi: 10.1111/j.1469-8137.1984.tb04152.x
Raven, J. A. (1997). Phagotrophy in phototrophs. Limnol. Oceanogr. 42, 198–205. doi: 10.4319/lo.1997.42.1.0198
Raven, J. A., Beardall, J., Flynn, K. J., and Maberly, S. C. (2009). Phagotrophy in the origins of photosynthesis in eukaryotes and as a complementary mode of nutrition in phototrophs: relation to darwin’s insectivorous plants. J. Exp. Bot. 60, 3975–3987. doi: 10.1093/jxb/erp282
Reich, H. G., Rodriguez, I. B., LaJeunesse, T. C., and Ho, T. Y. (2020). Endosymbiotic dinoflagellates pump iron: differences in iron and other trace metal needs among the Symbiodiniaceae. Coral Reefs 39, 915–927. doi: 10.1007/s00338-020-01911-z
Reumann, S., Inoue, K., and Keegstra, K. (2005). Evolution of the general protein import pathway of plastids (review). Mol. Membr. Biol. 22, 73–86. doi: 10.1080/09687860500041916
Reyes-Prieto, A., Moustafa, A., and Bhattacharya, D. (2008). Multiple genes of apparent algal origin suggest ciliates may once have been photosynthetic. Curr. Biol. 18, 956–962. doi: 10.1016/j.cub.2008.05.042
Rodríguez-Ezpeleta, N., Brinkmann, H., Burey, S. C., Roure, B., Burger, G., Löffelhardt, W., et al. (2005). Monophyly of primary photosynthetic eukaryotes: green plants, red algae, and glaucophytes. Curr. Biol. 15, 1325–1330. doi: 10.1016/j.cub.2005.06.040
Rose, J. M., and Caron, D. A. (2007). Does low temperature constrain the growth rates of heterotrophic protists? Evidence and implications for algal blooms in cold waters. Limnol. Oceanogr. 52, 886–895. doi: 10.4319/lo.2007.52.2.0886
Rusterholz, P. M., Hansen, P. J., and Daugbjerg, N. (2017). Evolutionary transition towards permanent chloroplasts?—division of kleptochloroplasts in starved cells of two species of Dinophysis (Dinophyceae). PLoS One 12:e0177512. doi: 10.1371/journal.pone.0177512
Sagan, L. (1967). On the origin of mitosing cells. J. Theor. Biol. 14, 225–IN6. doi: 10.1016/0022-5193(67)90079-3
Saldarriaga, J. F., Taylor, F. J. R., Keeling, P. J., and Cavalier-Smith, T. (2001). Dinoflagellate nuclear SSU rRNA phylogeny suggests multiple plastid losses and replacements. J. Mol. Evol. 53, 204–213. doi: 10.1007/s002390010210
Schimper, A. F. W. (1883). Uber die entwicklung der chlorophyllkorner und farbkorper. Bot. Zeit. 41, 105–114.
Schneider, L., Anestis, K., Mansour, J., Anschütz, A., Gypens, N., Hansen, P., et al. (2020). A dataset on trophic modes of aquatic protists. Biodivers. Data J. 8:e56648. doi: 10.3897/bdj.8.e56648
Sellers, C. G., Gast, R. J., and Sanders, R. W. (2014). Selective feeding and foreign plastid retention in an Antarctic dinoflagellate. J. Phycol. 50, 1081–1088. doi: 10.1111/jpy.12240
Selosse, M. A., Charpin, M., and Not, F. (2017). Mixotrophy everywhere on land and in water: the grand écart hypothesis. Ecol. Lett. 20, 246–263. doi: 10.1111/ele.12714
Sforza, E., Cipriani, R., Morosinotto, T., Bertucco, A., and Giacometti, G. M. (2012). Excess CO2 supply inhibits mixotrophic growth of Chlorella protothecoides and Nannochloropsis salina. Bioresour. Technol. 104, 523–529. doi: 10.1016/j.biortech.2011.10.025
Sibbald, S. J., and Archibald, J. M. (2020). Genomic insights into plastid evolution. Genome Biol. Evol. 12, 978–990. doi: 10.1093/gbe/evaa096
Skovgaard, A. (1996). Mixotrophy in Fragilidium subglobosum (Dinophyceae): growth and grazing responses as functions of light intensity. Mar. Ecol. Prog. Ser. 143, 247–253. doi: 10.3354/meps143247
Skovgaard, A. (2000). A phagotrophically derivable growth factor in the plastidic dinoflagellate Gyrodinium resplendens (Dinophyceae). J. Phycol. 36, 1069–1078. doi: 10.1046/j.1529-8817.2000.00009.x
Smalley, G. W., Coats, D. W., and Stoecker, D. K. (2003). Feeding in the mixotrophic dinoflagellate Ceratium furca is influenced by intracellular nutrient concentrations. Mar. Ecol. Prog. Ser. 262, 137–151. doi: 10.3354/meps262137
Smith, M., and Hansen, P. J. (2007). Interaction between Mesodinium rubrum and its prey: importance of prey concentration, irradiance and pH. Mar. Ecol. Prog. Ser. 338, 61–70. doi: 10.3354/meps338061
Stoecker, D. K., Hansen, P. J., Caron, D. A., and Mitra, A. (2017). Mixotrophy in the marine plankton. Annu. Rev. Mar. Sci. 9, 311–335. doi: 10.1146/annurev-marine-010816-060617
Stoecker, D. K., Johnson, M. D., De Vargas, C., and Not, F. (2009). Acquired phototrophy in aquatic protists. Aquat. Microb. Ecol. 57, 279–310. doi: 10.3354/ame01340
Stoecker, D. K., and Lavrentyev, P. J. (2018). Mixotrophic plankton in the polar seas: a pan-Arctic review. Front. Mar. Sci. 5:292. doi: 10.3389/fmars.2018.00292
Theissen, U., and Martin, W. (2006). The difference between organelles and endosymbionts. Curr. Biol. 16, R1016–R1017. doi: 10.1016/j.cub.2006.11.020
Timmis, J. N., Ayliff, M. A., Huang, C. Y., and Martin, W. (2004). Endosymbiotic gene transfer: organelle genomes forge eukaryotic chromosomes. Nat. Rev. Genet. 5, 123–135. doi: 10.1038/nrg1271
Tippit, D. H., and Heaps, J. D. P. (1976). Apparent amitosis in the binucleate dinoflagellate Peridinium balticum. J. Cell Sci. 21, 273–289. doi: 10.1242/jcs.21.2.273
Tomas, R. N., Cox, E. R., and Steidinger, K. A. (1973). Peridinium balticum (Levander) Lemmermann, and unusual dinoflagelate with a mesocaryotic and an eucaryotic nucleus. J. Phycol. 9, 91–98. doi: 10.1111/j.0022-3646.1973.00091.x
Trench, R. K., and Thinh, L. V. (1995). Gymnodinium linucheae sp. Nov.: the dinoflagellate symbiont of the jellyfish Linuche unguiculata. Eur. J. Phycol. 30, 149–154. doi: 10.1080/09670269500650911
Uwizeye, C., Brisbin, M. M., Gallet, B., Chevalier, F., Lekieffre, C., Schieber, N., et al. (2020). Cytoklepty in the plankton: a host strategy to optimize the bioenergetic machinery of endosymbiotic algae. bioRxiv. [Preprint]. doi: 10.1101/2020.12.08.416644
Uzuka, A., Kobayashi, Y., Onuma, R., Hirooka, S., Kanesaki, Y., Yoshikawa, H., et al. (2019). Responses of unicellular predators to cope with the phototoxicity of photosynthetic prey. Nat. Commun. 10:5606. doi: 10.1038/s41467-019-13568-6
Van Steenkiste, N. W. L., Stephenson, I., Herranz, M., Husnik, F., Keeling, P. J., and Leander, B. S. (2019). A new case of kleptoplasty in animals: marine flatworms steal functional plastids from diatoms. Sci. Adv. 5:eaaw4337. doi: 10.1126/sciadv.aaw4337
von Heijne, G. (1986). Why mitochondria need a genome. FEBS Lett. 198, 1–4. doi: 10.1016/0014-5793(86)81172-3
Ward, B. A., Dutkiewicz, S., Barton, A. D., and Follows, M. J. (2011). Biophysical aspects of resource acquisition and competition in algal mixotrophs. Am. Nat. 178, 98–112. doi: 10.1086/660284
Wilken, S., Choi, C. J., and Worden, A. Z. (2020). Contrasting mixotrophic lifestyles reveal different ecological niches in two closely related marine protists. J. Phycol. 56, 52–67. doi: 10.1111/jpy.12920
Wilken, S., Huisman, J., Naus-Wiezer, S., and Van Donk, E. (2013). Mixotrophic organisms become more heterotrophic with rising temperature. Ecol. Lett. 16, 225–233. doi: 10.1111/ele.12033
Wilken, S., Yung, C. C. M., Hamilton, M., Hoadley, K., Nzongo, J., Eckmann, C., et al. (2019). The need to account for cell biology in characterizing predatory mixotrophs in aquatic environments. Philos. Trans. R. Soc. Lond. Ser. B Biol. Sci. 374:20190090. doi: 10.1098/rstb.2019.0090
Williams, B. A. P., and Keeling, P. J. (2003). Cryptic organelles in parasitic protists and fungi. Adv. Parasitol. 54, 9–68. doi: 10.1016/S0065-308X(03)54001-5
Yamada, N., Bolton, J. J., Trobajo, R., Mann, D. G., Dąbek, P., Witkowski, A., et al. (2019). Discovery of a kleptoplastic ‘dinotom’ dinoflagellate and the unique nuclear dynamics of converting kleptoplastids to permanent plastids. Sci. Rep. 9:10474. doi: 10.1038/s41598-019-46852-y
Yoon, H. S., Reyes-Prieto, A., Melkonian, M., and Bhattacharya, D. (2006). Minimal plastid genome evolution in the Paulinella endosymbiont. Curr. Biol. 16, 670–672. doi: 10.1016/j.cub.2006.08.018
Yutin, N., Wolf, M. Y., Wolf, Y. I., and Koonin, E. V. (2009). The origins of phagocytosis and eukaryogenesis. Biol. Direct 4, 1–26. doi: 10.1186/1745-6150-4-9
Keywords: evolution, mixotrophy, endosymbiosis, plankton, kleptoplasty, plastids
Citation: Mansour JS and Anestis K (2021) Eco-Evolutionary Perspectives on Mixoplankton. Front. Mar. Sci. 8:666160. doi: 10.3389/fmars.2021.666160
Edited by:
Viola Liebich, Bremen Society for Natural Sciences, GermanyReviewed by:
Elisabeth Hehenberger, GEOMAR Helmholtz Center for Ocean Research Kiel, GermanyPatricia M. Glibert, University of Maryland Center for Environmental Science (UMCES), United States
Copyright © 2021 Mansour and Anestis. This is an open-access article distributed under the terms of the Creative Commons Attribution License (CC BY). The use, distribution or reproduction in other forums is permitted, provided the original author(s) and the copyright owner(s) are credited and that the original publication in this journal is cited, in accordance with accepted academic practice. No use, distribution or reproduction is permitted which does not comply with these terms.
*Correspondence: Konstantinos Anestis, a2FuZXN0aXNAYXdpLmRl
†These authors have contributed equally to this work
‡ORCID: Joost Samir Mansour orcid.org/0000-0002-9505-1673
Konstantinos Anestis orcid.org/0000-0002-8208-6789