Recent Advances in Data Logging for Intertidal Ecology
- Department of Marine and Environmental Sciences, Marine Science Center, Northeastern University, Nahant, MA, United States
Temperature is among the most ubiquitous determinants of organism growth, survival, and reproduction. Accurate recordings and predictions of how the temperatures of plants and animals vary in time and space are therefore critical to forecasting the likely impacts of global climate change. Intertidal zones have long served as a model ecosystem for examining the role of environmental stress on patterns of species distributions, and are emerging as models for understanding the ecological impacts of climate change. Intertidal environments are among the most physically demanding habitats on the planet, and excursions in body temperature of ectotherms can exceed 25°C over the course of a few hours. It is now well-known that the body temperatures of intertidal organisms can deviate significantly from the temperature of the surrounding air and substrate due to the influence of solar radiation, and that their size, color, morphology, and material properties markedly influence their temperatures. While many intertidal organisms are slow moving or almost entirely sessile, for others, behavior can play a significant role in driving vulnerability to temperature extremes. We explore datalogging methods used in intertidal zones and discuss the advantages and drawbacks of each. We show how measurements made in situ reveal patterns of thermal stress that otherwise would be undetectable using more remotely-sensed data. Additionally, we explore the idea that the relevant “grain size” of the physical environment, and thus the spatial scale that must be measured, is a function of (1) the size of the organism relative to local refugia; (2) an organism's ability to sense and to some degree predict near-term environmental conditions; and (3) an animal's movement speed and directionality toward refugia. Similarly, relevant temporal scales depend on the size, behavior, and physiological response of the organism. While miniaturization of dataloggers has significantly improved, several significant limitations still exist, many of which relate to difficulties in recording behavioral responses to changing environmental conditions. We discuss recent innovations in monitoring and modeling intertidal temperatures, and the important role that they have played in bridging ecological and physiological studies of ongoing impacts of climate change.
Introduction
Temperature is among the most universal determinants of a plant or animal's physiological performance and survival (Somero, 2010; Sinclair et al., 2016). Metabolism, heart rate (in animals with hearts), and enzyme functioning are all strongly temperature-dependent. At whole organism levels, temperature determines rates of growth and reproductive output. For mobile animals, body temperature can drive movement behavior, and thus ability to eat or avoid being eaten by other organisms (e.g., Adolph and Porter, 1993; Kordas et al., 2011). At extreme high or low temperatures, reproductive failure, and/or mortality occur as physiological systems shut down, enzymes cease functioning, or oxygen supply is no longer able to meet metabolic demands (Williams, 1970; Pörtner et al., 2006). Subsequently, temperature has long been recognized as a key driver of the distribution and abundance and hence biodiversity of plants and animals in nature (Hutchins, 1947; Ehrlén and Morris, 2015; Peters et al., 2016). The influence of temperature is especially evident in ectothermic organisms (which comprise the vast majority of species on Earth) that are unable to generate appreciable metabolic heat and thus have body temperatures that change with environmental conditions. While endotherms (birds and mammals) can generate heat through metabolism, they too will die or suffer stress when the maintenance of optimal body temperature becomes too challenging (Porter et al., 2000). Importantly, species display a wide range of responses to body temperature, and a temperature that may be lethal to one species may be optimal for another; while some species can tolerate only very narrow ranges of temperature, others appear to function well over wide ranges (e.g., >20°C; Dell et al., 2014).
Understanding how temperature affects organisms has taken on critical significance in the face of ongoing climate change (Porter et al., 2000; Hobday et al., 2016), as scientists attempt to understand underlying drivers of current observations of mortality events and shifts in distribution, and potentially forecast responses to an even warmer planet (Petchey et al., 2015; Sunday et al., 2015). A key component of this work has been the development of empirical and theoretical approaches that connect what we know about the physiological effects of factors such as temperature, ocean pH, and water availability—often measured under controlled experimental conditions (Somero, 2010; Williams et al., 2011; Gunderson et al., 2016)—with patterns of environmental conditions observed in the field (Denny and Helmuth, 2009). Specifically, numerous physiological studies have measured both the lethal tolerance limits of plants and animals, as well as the non-lethal cumulative effects of exposure to chronic stress (Woodin et al., 2013; Dell et al., 2014; Sinclair et al., 2016). At least in theory, these lab experiments can then be compared against measured environmental conditions (nowcasts and hindcasts) as well as model projections (forecasts) to quantify how patterns of survival, abundance, distribution, reproduction, and growth of key organisms have responded, or likely will respond, to rapid environmental change (Porter et al., 1973).
A major obstacle to such approaches has been a quantitative understanding of the environmental conditions that organisms actually experience in the field (Smale and Wernberg, 2009), and of what spatial and temporal scales we must measure these parameters in order to effectively forecast responses to environmental change (Montalto et al., 2014). The challenge is far more difficult than is often appreciated, especially given the wide availability of environmental data from ground-based weather stations and buoys (e.g., Helmuth, 1998; Kearney et al., 2012), remote sensing platforms (e.g., Geller et al., 2017), and re-analysis databases that present weather data integrated from multiple platforms (e.g., Mesinger et al., 2006; Mislan and Wethey, 2011). Below we briefly explain why such data, while necessary, may at times be insufficient for understanding the effects of climate change on organisms, and then explore various options for recording relevant environmental data at the scale of organisms using logging devices.
We focus on intertidal zones, the regions between the high and low tide lines of the world's coastlines. These habitats have long served as test beds for understanding the causal linkages between organism physiology and local and geographic patterns of distribution (e.g., Doty, 1946; Southward, 1958). Alternately exposed to the aquatic (at high tide) and terrestrial (at low tide) environments, the patterns by which species more or less predictably replace one another moving from the low to high intertidal, i.e., zonation (Figure 1), are generally assumed to be determined to a large extent by physiological stress from temperature, desiccation, and time spent feeding (Connell, 1961; Wethey, 1983, 1984; Gilman and Rognstad, 2018). Many of these organisms have been shown experimentally to live close to their stress tolerance limits during low tide (Somero, 2002; Davenport and Davenport, 2005; Harley, 2008). As a result, and because of their enormous ecological importance to coastal environments and easy accessibility to researchers, intertidal zones have contributed significantly to ecological theory (Paine, 1994). Yet, despite their importance, they are also among the environments where we have the least amount of environmental data. Here we use intertidal habitats as a case study, but the concepts that we explore have broad applicability to other environments and organisms including plant communities (e.g., Scherrer and Körner, 2011), terrestrial arthropods (Caillon et al., 2014; Woods et al., 2015), and lichens (Kershaw, 1985).
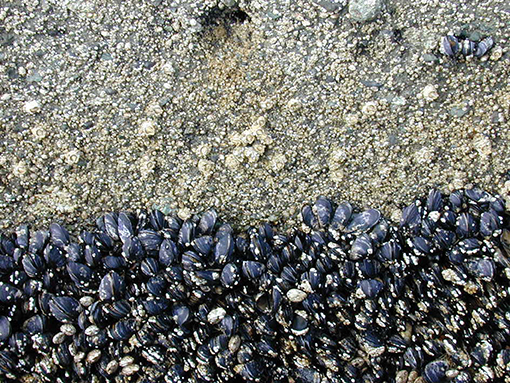
Figure 1. Zonation pattern of mussels and barnacles. The upper intertidal limit to intertidal organisms is generally assumed to be driven by physiological stress and feeding time (Connell, 1961; Wethey, 1983, 1984; Gilman and Rognstad, 2018).
The Challenge of Recording Intertidal Data at Relevant Scales
Environmental Temperature Data
The role of “temperature” in ecological and physiology studies is ubiquitous. A quick search of the Web of Science with the terms “Temperature” and “Ecolog* or Physiol*” returns almost 700,000 papers published since 1975. Yet the term “temperature” continues to be misused with subsequent carry-on effects for experimental design and interpretation of results. Critically, “temperature” is not a stand-alone variable, but rather a descriptor, and so studies that refer to “the temperature” (e.g., as a descriptor of a site) are about as meaningful as referring to “the color.” Most commonly, researchers are referring to air temperature recorded at some fixed elevation above the substratum, usually by a weather station (Tair); Sea Surface Temperature (SST); or, primarily in remote sensing literature, Land Surface Temperature (LST). Such inaccuracies are understandable given how we humans live our daily lives, using reports of near-ground air temperature as an appropriate indicator for how comfortable we are likely to be outside on any given day. Under more extreme conditions, we may also consider indices such as “wind chill” to reflect the influence of wind on heat loss through convection, or a “heat index” to reflect the role that high humidity has in limiting our ability to cool through sweating (Parsons, 2014). But, the assumption that these measurements and indices are physiologically and ecologically relevant to non-human species is often wildly violated. For example, SST can be very different from water temperature just a few meters below the surface (Smale and Wernberg, 2009; Castillo and Lima, 2010; Brewin et al., 2018). In terrestrial alpine environments, temperatures recorded at 2 m above-ground weather stations can be very different than temperatures in and around underlying plant canopies (Scherrer and Körner, 2010). In coastal systems, measurements recorded by offshore buoys (the most common data source) or satellites can differ markedly from those nearshore due to the influence of upwelling and solar heating (Pfister et al., 2007). Kearney (2006) refers to these large-scale measurements (also including those made by weather stations) as “environmental” temperature and explains that they can deviate significantly from those experienced by animals and plants—which drive their physiology and survival.
Many potential problems with the use of environmental data center on the large spatial and temporal scales over which they are, by necessity given the enormous size of the ocean, recorded, and archived. Satellite measurements of SST and LST record averages that integrate temperatures over pixel sizes ranging from 100's to 1,000's of square kilometers. In coastal systems, these pixels can overlap both terrestrial and aquatic measurements and thus can artificially reflect a hybrid of LST and SST. Because of rapid heating due to solar radiation, and cooling effects of internal waves and upwelling, temperatures at even moderate depths can vary by several degrees from SST (Smale and Wernberg, 2009; Castillo and Lima, 2010). Similarly, on land, while surface (substrate) temperature may be an accurate proxy for small organisms that live in close contact with the ground (e.g., Thomas, 1987; Bertness, 1989; Wethey, 2002; Chapperon and Seuront, 2011), LST pixels are typically many orders of magnitude larger than the size of these organisms, and thus are based on spatial averages that when parsed, can reveal extremely high within-pixel heterogeneity (Geller et al., 2017). Air temperature and wind speed change as a function of distance from the ground because of boundary layer effects (Gates, 1980), which is why weather stations typically adhere to standards where the thermometer and anemometer are placed at ~2 m and 10 m above the ground, respectively (World Meteorological Organization, 2008). Air temperature is thus always, at best, an indirect indicator of substratum temperature. Some animals are nocturnal, making measurements of environmental parameters recorded during the hottest part of the day largely irrelevant (Kearney, 2006). Similarly, in intertidal systems, the timing of low tide determines what part of the day organisms are exposed to the terrestrial, and by contrast, aquatic, environments. Environmental conditions recorded during the hottest part of the day have little relevance to an intertidal organism that is underwater during that period (Helmuth et al., 2002).
For intertidal (and terrestrial) environments, the most important reason why broad-scale weather data are often ineffective as direct proxies for plant and animal temperature is the role of the organism itself in driving heat exchange across its surface through the absorption of heat energy from solar radiation, and the transfer of heat with the surrounding air through wind-driven convection (Porter and Gates, 1969). For example, the color of an animal's skin, shell, or fur significantly affects the amount of solar heat that is either absorbed or reflected (e.g., Mitton, 1977; Erasmus and De Villiers, 1982; Etter, 1988). Likewise, the shape and surface area of an organism drives the rate of convective exchange (Mitchell, 1976). Subsequently, two ectothermic organisms exposed to precisely the same environmental conditions can have body temperatures that radically differ from one another (>10°C; Broitman et al., 2009; Gilman et al., 2015), and can be either substantially hotter (>15°C) or cooler than the temperature of the surrounding air (Helmuth, 1998). Predators and their prey, for example, can experience significant differences in their temperature, and thus in physiological stress, even if they have similar physiological tolerances (Monaco and Helmuth, 2011).
The deviation of animal and plant body temperatures from Tair, SST, or LST has enormous implications for how we extrapolate from the lab to the field, and for how we compare physiological stress at different field sites. Recently, physiological ecologists have adopted the use of Thermal Safety Margins (TSM) to quantify the difference between an organism's lethal temperature (usually measured in the lab) and the maximum conditions that it will experience in the field (Sunday et al., 2011). A wide TSM suggests that these organisms may be less susceptible to warming than is an organism already living in a habitat where it is very close to its lethal limits (Woodin et al., 2013; Dong et al., 2017). In a scenario where body temperature is substantially hotter than air temperature, overall risk will be massively underestimated if physiological limits are compared against air temperature (Marshall et al., 2010). Even the assumption of air temperature as a relative indicator of stressful conditions can be problematic. While some models do predict that the most extreme body temperatures tend to occur on days with high air temperature and maximum solar radiation (Mislan et al., 2014), other comparisons of animal temperature against local air temperature have shown poor relationships, with root mean square errors on the order of 5–9°C (Kish et al., 2016). But, even in instances where air temperature is correlated with body temperature, a mechanism for determining “how hot is too hot” may still be difficult without some means of estimating how much hotter organism temperature is than air temperature under full sun, i.e., the offset (y-intercept) of the correlation (Kish et al., 2016).
An additional distinction, but one that is still frequently misunderstood or at least ignored by many ecologists and physiologists, is the difference between weather and climate (30+ year trends in weather conditions). Specifically, while climate change undeniably affects plants and animals, it does so indirectly through changes in local weather parameters such as air temperature, wind speed, rain, and solar radiation (Stenseth et al., 2002). The role of high frequency (hourly, daily) variability in driving physiological performance and survival remains an active area of research, but recent work shows the important role of time history (Drake et al., 2017; Koussoroplis et al., 2017), as well as that of rare, short-term extreme events (e.g., heat waves; Tsuchiya, 1983; Hobday et al., 2016) that can be masked through temporal averaging (Wethey et al., 2011; Robinet et al., 2013). Spatial variability can also be very high (Herring et al., 2016). Thus, for example, while the recent Paris Agreement made as its goal to limit the increase of the Earth's average global temperature to well below 2°C above pre-industrial levels (UNFCCC, 2015), sites in the Gulf of Maine are already displaying temperature deviations of nearly twice that magnitude over periods lasting several months (Pershing et al., 2015; see also references in chapter 3 on regional temperature trends in the recent IPCC report on 1.5°C Global Warming1 Hoegh-Guldberg et al., 2018). Subsequently, model projections based on high frequency (hourly) environmental data have been shown to yield very different projections than those based on coarser resolution (6 h: Montalto et al., 2014; monthly mean: Kearney et al., 2012) data inputs.
The end result is that if one wishes to replicate meaningful environmental conditions in a laboratory setting in order to gain insights into the impacts of global climate change, it is not sufficient to simply expose organisms to constant temperatures based on annual means or climatic norms (e.g., based on global or regional increases), as meaningful shorter-term deviations will far exceed these trends. While climatic data such as annual or decadal means made available through datasets such as the World Ocean Atlas are useful when conducting research on the climate system, they are effectively useless in physiological or ecological contexts, as they ignore not only important inter-annual variability but also variation over much shorter time periods which may have highly significant consequences for organisms such as heat waves (for an in-depth discussion of these issues, see Montalto et al., 2014; Denny, 2017).
Several mathematical (heat budget) models are now available to convert weather data (air temperature, wind speed, and solar radiation) into estimates of intertidal organism temperature (Elvin and Gonor, 1979; Bell, 1995; Helmuth, 1998, 1999; Denny and Harley, 2006; Finke et al., 2009; Szathmary et al., 2009; Helmuth et al., 2011; Iacarella and Helmuth, 2011; Sarà et al., 2011; Wethey et al., 2011; Marshall et al., 2015; Mislan and Wethey, 2015; Kish et al., 2016; Dong et al., 2017). These models range in complexity from simple regression-based approaches (Elvin and Gonor, 1979; Kish et al., 2016) to much more sophisticated land-based models (Wethey et al., 2011; Mislan and Wethey, 2015). However, and especially in intertidal ecosystems, their application has still tended to be limited to a few research groups. And, as with all models, their verification requires extensive in situ measurements of organism temperature.
Temperature Measurements in the Field
Collectively, these applications underline a critical need for field measurements of temperature at the scale of organisms, and over high temporal frequencies and small spatial scales. Several authors have recently highlighted the use of infrared thermography for recording temperature patterns in the field (Figure 2) (Meola and Carlomagno, 2004; Scherrer and Körner, 2010; Chapperon and Seuront, 2011; Lathlean and Seuront, 2014; Van Alstyne and Olson, 2014; Faye et al., 2016a; Lathlean et al., 2017). Thermography produces visible “thermal” images that are converted from infrared energy emitted and reflected on a given surface (Chapperon and Seuront, 2011). This method allows for spatial analysis of a habitat at a small organism's scale including measurements of shell and substratum which can then be used to calculate corresponding tissue temperatures of animals such as gastropods and discern habitat-specific thermoregulatory behavior (Chapperon and Seuront, 2011). There are numerous studies that use this method to examine microhabitats, behavior and physiology in response to thermal stress (e.g., Helmuth, 2002; Bulanon et al., 2009; Montanholi et al., 2010; Woods et al., 2015). Thermal infrared cameras have decreased markedly in price and now are available as part of packages that can be used on drones (Faye et al., 2016b) making their use attractive, especially for obtaining measurements of multiple organisms simultaneously (Scherrer and Körner, 2011). Their primary limitation in intertidal systems is that they typically cannot be left unattended and can only be deployed during low tide. Data measured using thermography are only discrete measurements, limiting temporal analysis to broad frequencies. Moreover, analysis of temperature patterns must be conducted by post-processing of the images obtained. A further complication involves calibration to the surface properties of the organism of interest, specifically the animal or plant's emissivity. This parameter describes the ability of an object to radiate energy in the infrared spectrum and is required in order for the camera to back-calculate temperature. Typically, biological surfaces fall in the range of 0.85–0.95, and so small errors in emissivity will make a difference of only a degree or two. However, some shelled organisms and rock can have much lower values, introducing error unless this is accounted for in image analysis.
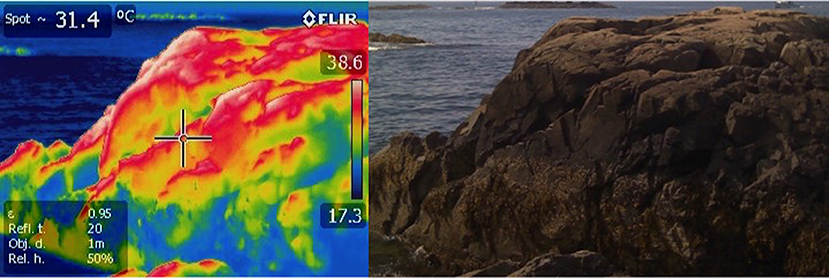
Figure 2. Infrared thermograph of a rocky intertidal site in Nahant, MA USA. Rocky intertidal temperatures range significantly based on complex topography that creates microclimates. These include both areas of high thermal risk and areas of thermal refugia.
An alternative approach that has seen more wide-scale adoption by intertidal researchers is the deployment of small sensors to directly document organism body temperatures in the field. Initially, researchers attempted to follow the lead of terrestrial studies through the use of thermocouples and thermistors connected to a central data logger (Southward, 1958; Lewis, 1963; Hardin, 1968; Vermeij, 1971; Elvin and Gonor, 1979). Early dataloggers include the Portable Chart Recorder (Omega Engineering) used in recording N. lapillus body temperatures (Etter, 1988), Stowaway XTR (Onset Computer Corporation) initially used for logging silicone-filled M. californianus shells (Helmuth and Hofmann, 2001) and live P. ochraceus (Szathmary et al., 2009), Telatemp Datalogger for logging M. californianus body temperatures (Fitzhenry et al., 2004), and Campbell CR1000 Datalogger used with M. californianus (Fitzhenry et al., 2004), G. demissa (Jost and Helmuth, 2007), and L. irroratus (Iacarella and Helmuth, 2011). While these devices were valuable for logging multiple body temperatures simultaneously during lab experiments, they often could only be deployed in the field for short periods during low tide. During long-term deployments, flooding, broken cables, and movement of the temperature probe tip made data collection extremely difficult (Helmuth and Hofmann, 2001; but see successful recent application by Gilman et al., 2015).
In the mid to late 1990's, self-contained units with on-board thermistors were introduced commercially by companies such as Dallas/Maxim (iButton), and Onset Computer Corporation (Hobo TidbiT) (Table 1). The ready availability of these rugged and relatively inexpensive loggers initiated a new wave of environmental measurements in intertidal systems, and their application continues to this day. But, as often happens with new technology, these instruments were frequently used inappropriately. Specifically, what went largely unrecognized was that just as the shape, color and mass of an organism affect its body temperature, so do those characteristics affect the temperature that a logger will record. When buried in sand or mud (Jost and Helmuth, 2007), or placed in close contact with the surface of a rock (Wethey, 2002; Harley and Helmuth, 2003), these instruments will record temperatures close to that of the substratum because of high rates of thermal conduction. They therefore can serve as effective indicators of body temperature in sessile animals such as barnacles (Wethey, 2002), small snails (Hayford et al., 2015; Marshall et al., 2015) or, on the underside of rocks, crabs (Stillman and Somero, 1996). Such measurements cannot, however be applied to all organisms at a site since all are likely to have very different body temperatures, both due to habitat heterogeneity (microhabitats) as well as a result of their thermal properties, as above. In fact, Denny et al. (2011) showed that variation in mussel temperatures within a single intertidal bench can exceed that observed over several thousand kilometers. Fitzhenry et al. (2004) compared temperature measurements by unmodified TidbiT loggers against body tissue temperature measurements of adjacent mussels in the lab and field and showed that the loggers recorded errors of up to 14°C. Claims of “site level temperature” measurements were therefore shown to be naïve.
A viable alternative which takes advantage of the rugged nature of instruments such as iButtons and TidbiTs is the use of biomimetic instruments (biomimics) designed to mimic the thermal characteristics of the target species of interest (mussels, limpets, barnacles, snails, seastars, etc.; Table 1; Helmuth et al., 2002, 2006, 2011; Seabra et al., 2011; Fly et al., 2012; Pincebourde et al., 2012; Monaco et al., 2015; Kish et al., 2016; Kroeker et al., 2016; Drake et al., 2017). These instruments record temperatures that are significantly more accurate (~1–2°C error) to the body temperatures of animals than are either air temperature or the temperature recorded by unmodified “off the shelf” instruments (Fitzhenry et al., 2004; Lima et al., 2011). Methods for building sensors have usually involved either placing the logger inside of an animal (e.g. mussel, limpet, barnacle, snail) shell and filling the shell with silicone or Scotchcast flame retardant compound both of which adequately approximate the thermal characteristics of tissue (Lima and Wethey, 2009; Denny et al., 2011) (Figure 3A) or by embedding them in materials such as epoxy resin (Figure 3B; Helmuth and Hofmann, 2001; Lima et al., 2011). A disadvantage of the latter approach is that not all sizes or species of animal can be replicated. For example, an organism's “thermal inertia,” which defines the amount of energy required to raise its temperature by one degree, can only be matched with non-biological materials such as epoxy over a narrow size range. For mussels, this was shown to occur in the size range of ~6–8 cm length (Fitzhenry et al., 2004). Biomimics of mussels at sizes smaller or larger than this range requires the use of real shells, which can break in the field after only a few weeks. Nevertheless, “robomussels” and “robolimpets” have now successfully been deployed at sites worldwide, and have provided long-term records of temperatures approximating those of the species of interest (Seabra et al., 2011; Helmuth et al., 2016). Obtaining temperature records of animals too small to contain current data logger designs remains a challenge, and most solutions still require the use of a thermocouple or thermistor (e.g., Gilman et al., 2015). However, emerging technology with smaller batteries (e.g., EnvLogger, WeePit) is poised to open new avenues of exploration for a wider range of animal sizes (Table 2).
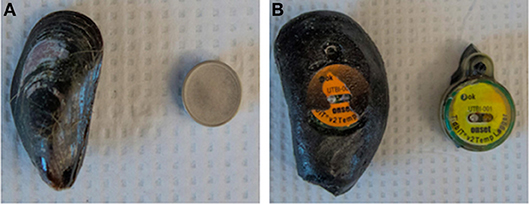
Figure 3. (A) An iButton temperature logger (Maxim Integrated) next to a real Mytilus edulis mussel shell. This biomimic is made by filling the shell with silicone and embedding the logger within. (B) An unmodified HOBO TidbiT v2 temperature logger (Onset Computer Corporation) next to a biomimic. The biomimic is a custom cast made of Evercoat Premium Marine Resin modeled after a larger (74 mm) mussel (Fitzhenry et al., 2004), more suitable for use approximating body temperatures of the larger M. californianus species from the Pacific Ocean.
Long-term records of non-shelled organisms have proven much more elusive, especially for those with a wet surface that cannot be mimicked with epoxy. Loggers constructed of foam have been used to approximate temperatures of intertidal seastars for periods of several months (Figure 4; Table 1; Fly et al., 2012; Pincebourde et al., 2012; Monaco et al., 2015). But, the lack of a design which effectively mimics water loss (and thus cooling through evaporation) and yet is sufficiently rugged to withstand wave stresses, continues to be a major impediment to understanding the thermal ecology of soft-bodied intertidal organisms.
Despite these challenges, the use of biomimetics in intertidal ecology has yielded major insights that otherwise would likely have gone undetected. First and foremost, these field measurements have shown that in many cases intertidal organisms are living far closer to the limits of their thermal tolerance than would be predicted based on air temperature (e.g., Stillman and Somero, 1996; Helmuth and Hofmann, 2001; Mislan et al., 2014). Second, they have shown that patterns of temperature on local and geographic scales are far more complex in space and time than previously appreciated. For example, Helmuth et al. (2002, 2006) showed that intertidal mussels along the west coast of North America do not display a geographic gradient in temperatures, but rather conform to a mosaic pattern where sites can be much hotter or colder than predicted based on latitude. These complex patterns have been shown to occur in Europe (Pearson et al., 2009; Seabra et al., 2011) and SE Asia (Dong et al., 2017), although not on the west coast of South America (Finke et al., 2007). Importantly, these observations suggest that climate change may be having significant impacts even well within species' geographic ranges and not just at range boundaries (Place et al., 2008; Pearson et al., 2009; Torossian et al., 2016). Kroeker et al. (2016) expanded on this idea to explore the impacts of multiple stressors acting on mussel populations, and showed mosaic patterns of not just temperature but food availability and ocean pH explained observed geographic patterns in mussel growth. Results from biomimic deployments also have pointed to the likely importance of stepping stones and climate refugia (“rescue sites”) in coastal ecosystems, which may enhance the recovery of species following extreme events (Potter et al., 2013; Hannah et al., 2014).
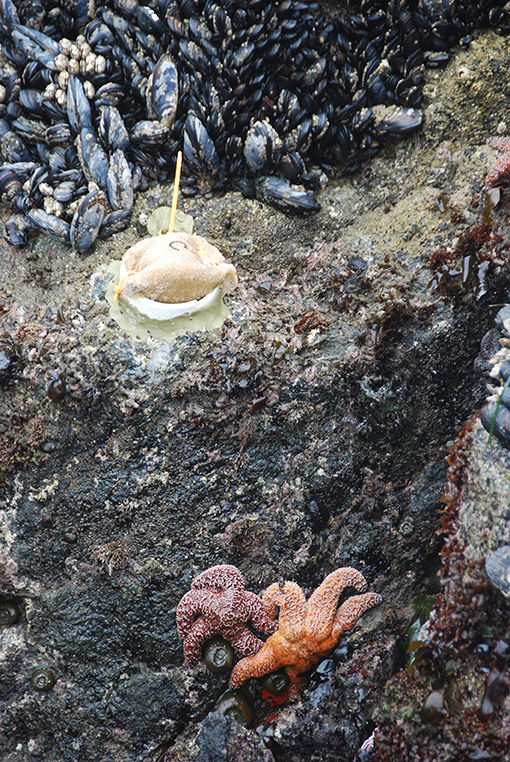
Figure 4. A “roboseastar” biomimic deployed in the intertidal next to live seastars (Pisaster ochraceus) and their mussel prey. An iButton temperature logger is embedded within the foam and the unit is affixed to the rock using marine epoxy.
Biomimetic sensors have also opened new avenues for exploring the differential responses of interacting species to environmental stress. Broitman et al. (2009) used biomimics to track the temperatures of predatory seastars and their mussel prey at multiple sites and showed that relative stress levels were significantly affected by differences in the thermal dynamics of the two species. Zardi et al. (2010) found that competition among different lineages of the mussel Perna perna were maintained in part by physiological stress that occurred during aerial exposure at low tide.
Current Limitations and Recent Advances in Logger Design
Despite their demonstrated importance in informing ecological theory, intertidal dataloggers still have several limitations. We next examine some of these in detail, along with recent progress being made in overcoming these barriers.
Durability in Wave-Swept Environments
The intertidal environment is unique due to the binary nature of environmental stressors resulting from the tidal cycle. Crashing intertidal waves can produce water velocities exceeding 8 m/s and accelerations of up to 400 m/s2 (Denny, 1985). Thus, loggers need to resist dislodgement, physical impact and seawater ingress. Current loggers still face some of these durability limitations, both in their construction and deployment. Two-part marine epoxies are still commonly used to attach intertidal biomimetics to the underlying rock surface. The amount of epoxy must be sufficient to resist removal during storms, yet an excess will affect the thermal characteristics of the logger. While superficially this issue appears trivial, and is not typically considered in terrestrial environments, it represents a major limitation in exposed rocky intertidal systems.
The materials used to construct biomimetic loggers also represent a design limitation, as the thermal conductivity as well as the specific heat capacity will affect their relevance to organisms. The growing availability of plastic and rubber mold-making materials, traditionally used by hobbyists and performing arts professionals, will expand the range of options for future biomimics, particularly for soft-bodied organisms like seastars and macroalgae. One such company called Smooth-On2 manufactures several user-friendly silicone and urethane-based rubbers, foams and polyester hard epoxies that are traditionally used for sculpting and performing arts. The company has products available in stores on 6 continents and has a global shipping distribution network. These products vary in tear strength resistance, hardness, and conductivity. Those with the highest tear strengths are less likely to break apart due to wave stress, but their other thermal properties must meet the requirements of the organism being considered. In preliminary observations, some of these non-epoxy materials, particularly the ones meant to be vacuum degassed (i.e., the removal of entrapped air bubbles upon pouring), may harden into objects with a textured, semi-permeable surface when poured without vacuum degassing. This technique may facilitate the development of more accurate biomimics that can partially simulate a live organism's ability to retain seawater upon emersion, then release it via evaporation. The materials may be colored to mimic albedo and casted with a UV resistant agent which reduces breakdown in the field due to solar radiation. In addition, some of these materials are food-safe and non-toxic, making them more attractive options for use over traditional materials like polyester epoxy.
Lack of Real-Time Data Capabilities
Scientists have increasingly turned to the acquisition of real-time data from their instruments (Zhou et al., 2018). Utilizing real time data capabilities for sensing in the intertidal environment, however, is extremely challenging due to the tides. While real-time equipped data loggers have been used extensively in terrestrial research (Porter et al., 2005), they are not used commonly in marine research. This is due to the difficulty inherent in transmitting data wirelessly through water. Continuous, real time intertidal sensors are challenging to implement because wireless signals are transmitted very differently in water and air. Real time data transmission also requires considerable battery power, in direct conflict with the need to miniaturize sensors. Zhou et al. (2018) developed an intertidal sensor prototype for real-time data that tackles both the challenges of transmitting in water and battery life (Zhou et al., 2018). Specifically, they created a mesh intertidal wireless sensors network (IT-WSN) whereby sensors communicate and transfer data to each other. Transferring data between sister sensors is a method to strengthen connection. With each logger acting as a stepping stone, data are eventually pushed via a series of nodes to the land-based “base” or “sink” node that is connected to the cloud (Figure 5). To enhance battery longevity, a primary limitation to miniaturization, the sensors are designed to log data continuously but transmit data only when sensors are exposed to air at low tide and a strong network link can be made. This is done by incorporating a new complex metric in the sensor (PIDO: Predictive Delay Optimization) which controls for dormancy based on (1) node conditions (dryness of the sensor), (2) link quality (connectivity to sister nodes), and (3) predictability classifier (uploaded tide cycles) to control for dormancy for each sensor. With multiple factors confirming optimal data transmission, real-time PIDO sensors can be deployed for longer periods of time (Zhou et al., 2018).
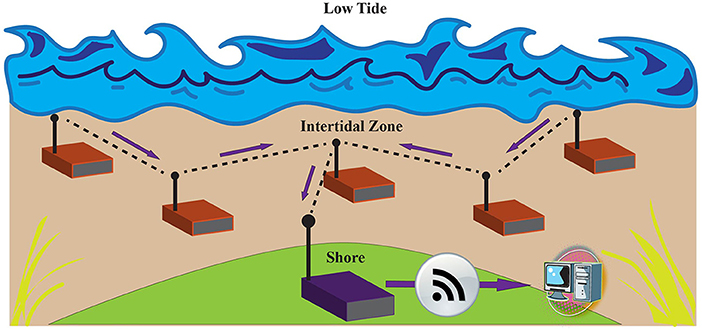
Figure 5. Diagram of a mesh Intertidal Wireless Sensor Network (IT-WSN) that uses PIDO (Predictive Delay Optimization) for more frequent and effective intertidal data transmission as described in Zhou et al. (2018). Environmental data are collected by the sensor nodes (red) where they is then transmitted via links (dashed lines) to other nodes (Freq. = 915 MHz) using the most efficient routing path during low tide. Data are then transmitted to a land-based sink node (purple) before they are offloaded to a computer.
In other cases, even when data cannot be recorded in real time, methods for facilitating the rapid transfer of data manually can speed data collection. Data from iButton loggers and older, LED-based versions of Onset TidbiT data loggers can only be uploaded to a computer or data shuttle directly using a cabled reader. Data collected by the newest wireless Onset TidbiT Bluetooth loggers (MX2204) are a significant improvement as data can be downloaded via Bluetooth on to a cell phone or other mobile device during low tide. A new Portuguese company, Electric Blue, has developed a small, ruggedized temperature logger (EnvLogger)3 that can offload data and be programmed using a mobile Android device and has near real-time data capabilities (Table 2). This new technology avoids the need to remove the logger from its shell casing or make time-costly modifications to offload data through protruding wires (e.g., Robert and Thompson, 2003; Lima and Wethey, 2009; Lima et al., 2011; Chan et al., 2016). WeePit temperature loggers have not been widely used in intertidal studies, however their large memory and high resolution are useful for long-term deployments. Additionally, it remains unclear whether the logger's data can be transmitted to the PitStop Radio-Frequency Identification (RFID) reader through shell, epoxy, or other biomimic components.
Miniaturization
Rapid advancements in printed circuit board and battery miniaturization have increased the availability of new datalogging technology. Existing sensors and loggers (Table 1) are small enough to be used to study many, although not all, intertidal organisms. The size of a self-contained logger is mostly determined by battery size. Some of the first uses of self-contained temperature loggers for intertidal biomimetic applications occurred in 2000-2001 where the first commercially available temperature loggers from Onset were slightly larger in circular diameter than 24 mm, and several cm thick. Some newer models of loggers are significantly smaller.
Mimicking Aggregation Effects
Most intertidal bivalves such as mussels and oysters commonly grow in aggregations that range in size from small (< 25 cm2) (Hunt and Scheibling, 2001) to large populations of hundreds of thousands of individuals (Okamura, 1986). Biomimics of shelled molluscs have been shown to record markedly different temperatures when deployed in growth position, in intact beds, as compared to when they are deployed as solitary individuals (Fitzhenry et al., 2004). Generally, loggers such as “robomussels” need to be deployed in intact beds, surrounded by living animals that provide shading (Figure 6). This limitation is increasingly important to address due to the extensive die-off of mussels in locations such as the Gulf of Maine (Sorte et al., 2016). The causes of these declines remain uncertain (Sorte et al., 2016), and studies attempting to explore whether the underlying causal factor is related to temperature face the problem that in many locations there are no intact mussel beds in which to deploy sensors. This severely limits our ability to record relevant temperatures in sites where animals have disappeared. An individual that is shaded by conspecifics within a bed can have 40% less surface area exposed to direct sunlight than mussels living as solitary individuals, and the bed as a whole creates a higher thermal inertia (Helmuth, 1998). Therefore, not only does the aggregation buffer any one individual from thermal stress, it also produces a matrix of individuals that can experience significantly different body temperatures based on their spatial position (Denny et al., 2011; Nicastro et al., 2012; Lathlean et al., 2016b). Similarly, oysters that orient their shells vertically to decrease surface area exposed to solar radiation can reduce thermal stress for themselves and smaller invertebrates that live amongst them within an oyster reef (McAfee et al., 2018). As we explore in more detail in a case study below, the development of low-cost open source hardware and software has allowed researchers to begin testing new biomimetic mussel bed devices to provide greater insight into how mussels and other invertebrates that live within beds experience differing environmental conditions, and how these may be mimicked using new sensor designs.
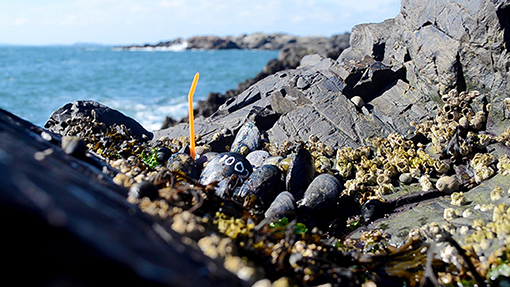
Figure 6. A single robomussel deployed in growth position within a live mussel bed. The presence of surrounding organisms helps improve biomimetic logger accuracy.
Accounting for Behavioral Thermoregulation
One of the biggest challenges facing logging of intertidal temperatures is understanding the role of animal behavior in determining body temperatures (Williams and Morritt, 1995; Williams et al., 2005). Under thermal stress, many organisms are adept at seeking or creating shaded microhabitats such as crevices or the shade of larger organisms (Potter et al., 2013; Scheffers et al., 2013; Sunday et al., 2014) or creating microclimates through aggregation behavior (Nicastro et al., 2012; Olabarria et al., 2016). Others burrow, living in conditions much cooler than those on the surface (Kearney et al., 2010). Leaf-mining insects create microenvironments by burrowing into plant tissue (Pincebourde and Casas, 2006), and crop pests benefit significantly from shade provided by the plants on which they feed (Faye et al., 2017).
Intertidal animals display a wide repertoire of behavioral strategies for thermoregulation. The most common form of behavioral thermoregulation involves pre-emptive movement in to crevices or other shaded microhabitats where heating from direct solar radiation is minimized, i.e., taking advantage of the immediate thermal landscape (Sears et al., 2016). Like terrestrial organisms, porcelain crabs may compete for cool, shaded areas under large rocks to reduce their body temperatures (Stillman and Somero, 1996). Many snail species are also adept at moving to crevices and other shaded areas to avoid extreme temperatures (Marshall et al., 2010; Cartwright and Williams, 2012; Ng et al., 2017). Monaco et al. (2016) measured potential and realized microhabitats in the more slowly-moving seastar Pisaster and found that these animals exhibited a “bet-hedging” strategy that allowed them to avoid extreme conditions, but at the expense of maximized physiological performance.
Slowly-moving or sessile species that are unable to avoid full sun can modify their body temperatures via several mechanisms. Hunt (1997) and Hunt and Scheibling (2002) suggested that individuals of the mussel Mytilus trossulus usually moved 1–2 cm in a month-long period, however some may move up to 50 cm over that same stretch. In contrast, Schneider et al. (2005) showed much more rapid movement, but only by small animals. Miller and Dowd (2017) noted that mussels living within a colder tidepool did not reposition themselves as much as those living on hotter bare rock in the high or low intertidal. Gastropods can reorient their shell and remove their foot from the geologic substrate to lower their body temperature (Miller and Denny, 2016).
Intertidal animals also display methods for thermoregulation that do not involve movement to shaded microhabitats. The seastar Pisaster has been shown to increase its thermal inertia by taking up cold water into its body during high tides preceding stressful low tide conditions (Pincebourde et al., 2009). The temperatures of intertidal algae likewise remain low as long as sufficient water is present to cool the surface through evaporation, but once significant desiccation occurs, thallus temperatures can skyrocket (Bell, 1995). This combination of desiccation and high temperature has significant effects on photosynthetic rates (Dring and Brown, 1982; Brown, 1987) and is thought to be the main determinant of algal species upper zonation limits in the intertidal zone (Lubchenco, 1980; Brown, 1987). Some species of gastropods can “mushroom” (Williams et al., 2005), cooling their bodies by exposing moist tissue, facilitating the evaporation of water. Yet other species climb atop one another to form chains, minimizing contact with the hot rock surface by all but the bottom-most animal (Ng et al., 2017).
Several studies have suggested the potential importance of shell gaping in bivalves as a means of thermoregulation, although to date results suggest high variability among species. During exposure at low tide, several intertidal bivalve species will remain tightly closed, but during extreme events will open their valves (gape), either to obtain oxygen or, potentially, to cool through evaporation of tissue water. Whether or not this behavior cools animals remains a matter of debate. Excessive levels of cooling through evaporation can lead to desiccation, suggesting significant trade-offs between temperature control and desiccation. Helmuth (1998) presented biophysical models for mussels suggesting that even slight evaporation can lead to notable decreases in body temperature. In contrast, Fitzhenry et al. (2004) forced mussel shells open inside a wind tunnel and compared temperatures to mussels that were forced shut. Their results suggested no significant difference in animal temperature. Miller and Dowd (2017) also found that live M. californianus mussels in the intertidal that experienced high body temperatures during low tide (>25°C) generally kept their shells closed more often than cooler mussels meaning this species likely does not utilize cooling through evaporation to control body temperature during hot conditions. Nicastro et al. (2012) showed that individual mussels did not apparently cool as a result of gaping, but suggested that entire beds of mussels, acting in concert, could lead to overall cooling.
Biomimetic sensors, unless attached to or imbedded in a living animal, can thus only provide information on potential body temperatures in the absence of behavioral thermoregulation (Adolph, 1990; Buckley et al., 2013; Díaz et al., 2015). Thus, there is a pressing need to better understand the cues and behavioral “rules” that allow intertidal organisms to proactively move to appropriate microhabitats. Typically this has been accomplished by placing biomimetic sensors in a number of potential microhabitats, and then determining the factors that cause organisms to move among these options, usually in response to trade-offs such as food availability, thermal stress and avoidance of predators (e.g., Monaco et al., 2015). Understanding the relationships between body temperature, shell gaping/orientation behaviors, and desiccation, and to link these trade-offs to physiological and molecular responses (e.g., Williams et al., 2011; Gleason et al., 2017) remains a significant information gap. Examining these factors simultaneously, in both the field and under controlled laboratory settings would provide an understanding of thermal physiological thresholds and how the organisms balance trade-offs. Progress is being made in this arena (Table 3). Researchers in the U.K. and the Netherlands have developed a biomonitoring device called the Musselmonitor that measures the amount and timing of mussel shell gaping using hall effect sensors (Allen et al., 2010). This device has been used on the freshwater zebra mussel (Driessena polymorpha) and the blue mussel (Mytilus edulis). A repeated pattern of shell gaping and closing is a signal of poor water quality. Mussels that spend the vast majority (70–80%) of their time with their shell open indicate good water quality. This device may be used to record early warning indicators of water quality in local areas of saltwater or freshwater, and can even be used to test drinking water quality. Hall effect sensors have also been used with oysters to investigate seasonal shell gaping patterns (Comeau et al., 2012) and gaping in response to the presence of toxic dinoflagellates (Nagai et al., 2006).
A device recently developed by Miller and Dowd (2017) used low cost custom-built printed circuit boards (PCBs) with type K thermocouples, waterproofed hall effect sensors, and an accelerometer/magnetometer to track mussel body temperature, shell gape, and orientation continuously in the field for a period of 21 days. The hall effect sensor, also used in the Musselmonitor, produces a magnetic field across the mussel's two half-shells where the output voltage gradient is proportional to the amount of shell gape. This type of system in the future should be used in controlled laboratory experiments along with other sensors to examine how mussel body temperature, respiration, heart rate, orientation, and shell gape all influence each other and determine the physiological performance of an individual under different conditions. Although these new datalogging capabilities provide researchers with valuable data, they are wired sensors which presents problems for trying to obtain long-term (>1 month) data sets from the field.
We also know surprisingly little about the role of predictability in the environment (temporal autocorrelation) in driving any of these behavioral responses (Helmuth et al., 2006; Dong et al., 2017). Some organisms may be able to behaviorally respond to immediate environmental conditions, for example by quickly moving during low tide to reach shaded microhabitat before critical levels occur. However, many species can only move during high tide, and “hunker in place” at low tide (Ng and Williams, 2006). In order for these organisms to successfully use appropriate microhabitats or to otherwise modify their behavior they must predict, at some level, the likelihood of near-term extreme events based on current conditions. Specifically, environmental conditions need to be temporally autocorrelated, i.e., when extreme conditions on Day 1 presage extreme conditions during the next low tide (Szathmary et al., 2009). However, at the few sites where this idea has been tested (Helmuth et al., 2006; Dong et al., 2017) the most extreme sites are often the least temporally autocorrelated.
Future Developments in Intertidal Datalogging
The development of newer, more complex biomimetic devices has been enhanced by open-source hardware (microcontroller circuit boards) and software programs such as Arduino and Raspberry Pi. These programs are revolutionizing the way marine scientists can collect oceanic data and have already been used in novel devices such as a microplastics sensor (Edson and Patterson, 2015), a CTD data logger (Lockridge et al., 2016), and a chlorophyll sensor (Leeuw et al., 2013). Novice engineers and programmers can learn to use microcontrollers to build electronic devices (e.g., dataloggers, robots, lights) for customized projects. These microcontrollers can be plugged into a computer via a USB cord and programmed using their corresponding computer applications (e.g., Arduino: Integrated Development Environment). Once programmed, a microcontroller will carry out the user-defined functions within the electrical circuit. First prototypes are typically designed by manually connecting the microcontroller to a solderless breadboard with integrated circuits (usually sensors and actuators) that are connected by jumper wires to form the full circuit. For projects that require smaller, more compact devices, these prototype designs can be documented (i.e., mapped) and submitted for development into custom printed circuit boards (e.g., Miller and Dowd, 2017).
Case Study: Biomimetic Mussel Bed
A project currently underway seeks to develop a biomimetic mussel bed that circumvents some of the issues associated with traditional robomussels. The biomimetic mussel bed consists of 23 mussel-shaped biomimics of sizes ranging from 25 to 60 mm in length—about the size of adolescent-adult M. edulis mussels found in muddy embayments and rocky shores on the East Coast of North America. These robomussels are synthesized using a colored UV resistant polyurethane rubber (Econ-80 from Smooth-On) a hard rubber material that closely matches the specific heat of live mussel tissue. The 2-part liquid polyurethane mixture can be poured into a silicone mold of several mussel shaped cavities all of which are fitted with nylon bolts, and two of which are fitted with type K thermocouple sensors. These thermocouples are then connected to an Arduino circuit board encased in a waterproof housing. Each mussel [including the experimental unit(s)] is then secured to an acrylic platform using nylon-insert locknuts, eliminating the need for marine epoxy. It can also be bolted to rock (Figure 7) or affixed to muddy bottom using thin rebar.
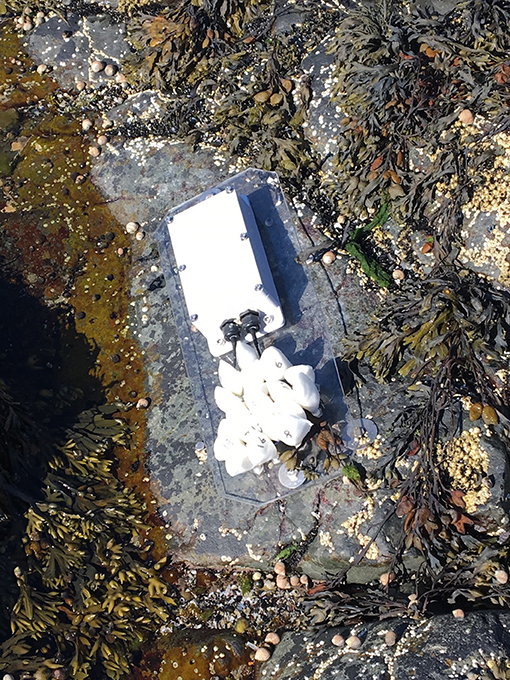
Figure 7. A robomussel bed prototype deployed in the rocky intertidal zone in Nahant, MA USA. The robomussels in this prototype are colored white in order to collect preliminary data on how albedo may affect the accuracy of biomimetic devices.
The Arduino is a microcontroller that can be programmed in the Arduino Integrated Development Environment (IDE) using the C/C++ programming languages. The board can be programmed to collect temperature measurements at any customized interval and stores them on a micro SD card. In addition, it uses software interrupts to go into a power-off mode between readings to further conserve power and can extend battery life for over a year (battery life depends on sampling interval). The logger is configured with two thermocouples, allowing for the simultaneous data collection of two different mussels within the bed. However, it uses the One-Wire library so more sensors may be added to the same data line with ease4.
The project also offers a customized solution for simultaneously logging body temperatures of Nucella lapillus gastropod predators foraging on mussels using biomimics. Casts of Nucella shells have been constructed to log body temperature of individuals at different positions on the mussel bed. The bed contains screw-in attachment points both on the top and bottom of the bed. Therefore, it is useful for gaining insight into the temperatures experienced by an intertidal predator and its prey based on spatial position. Nucella biomimics can be colored which helps account for the effects of albedo of the different color variants found in the wild. Therefore, the logger allows researchers to better understand the role of albedo and spatial position in shaping an organism's thermal experience.
In situ Movement Tracking
Radio-Frequency Identification (RFID) technology has been used in prior intertidal research for tracking organismal behavior. Nucella ostrina gastropods have been shown to advantageously venture into risky areas (i.e., areas closer to thermal maxima) for increased access to food by using the timing of the tide to their advantage (Hayford et al., 2015, 2018). These same investigations found that RFID can be used to replace traditional tagging or marking methods in the intertidal. Their results showed that RFID tagging methods are 10-fold more efficient as a mechanism for relocating specific individuals than were traditional means.
Conclusions
Significant advances in temperature logging in intertidal systems have provided significant insights in to how this model system will continue to respond to the ongoing threat of climate change. Advances in logger design, both in terms of materials used to construct loggers as well as the size, battery life, and live transmission of data make biomimetics ever more powerful for a wider range of species. A major limitation still lies in understanding the role of thermoregulatory behavior by intertidal animals, both through movement to microhabitats but also through non-movement thermoregulatory behavior. New tools for tracking movement through RFID and for monitoring behaviors such as gaping are opening avenues of research, but they must be coupled with a better understanding of the “grain size” of the environment that the organism perceives. A small crab that is able to scuttle to safety in a crevice when its body temperature exceeds a critical threshold will experience very different temporal and spatial patterns of small-scale refugia than will a large seastar that is limited both by availability of sufficiently large hiding places as well as by movement speed. In contrast, by virtue of a significantly greater thermal inertia, a larger animal may have less need of a hiding place except during extreme events (Monaco et al., 2015). All of these organism-environment interactions are occurring simultaneously at a single site, yet without insights in to the way that they experience their environments and one another our expectations of the impacts of environmental change will likely fail (Broitman et al., 2009; Gilman et al., 2015; Hayford et al., 2015).
The development of data sensing and logging tools that are accurate and most importantly relevant to the species of experimental interest is now more important than ever. This instrumentation must be combined with laboratory and field experiments that holistically evaluate the state of the species from an assemblage-based perspective. Once these approaches are taken, they may potentially be scaled up to regional assessments of species performance and distribution (e.g., Woodin et al., 2013). Advanced biologging techniques will allow for more accurate ecological forecasting that enhances our understanding of what to expect in the future. There are many unique challenges to monitoring intertidal organisms, however the rapid pace of technological improvement is making these challenges easier to overcome, and thus promises new insights from this model system.
Author Contributions
All authors contributed equally to the development and writing of the manuscript. RJ led the writing team and created the experimental mussel bed system described in this paper.
Conflict of Interest Statement
The authors declare that the research was conducted in the absence of any commercial or financial relationships that could be construed as a potential conflict of interest.
Acknowledgments
This research was supported by NSF grant OCE 1635989 to BH. This is contribution number 385 of the Northeastern University Marine Science Center.
Footnotes
References
Adolph, S. C. (1990). Influence of behavioral thermoregulation on microhabitat use by two Sceloporus lizards. Ecology 71, 315–327. doi: 10.2307/1940271
Adolph, S. C., and Porter, W. P. (1993). Temperature, activity, and lizard life histories. Am. Nat. 142, 273–295. doi: 10.1086/285538
Allen, H. J., Waller, W. T., Acevedo, M. F., Morgan, E. L., Dickson, K. L., and Kennedy, J. H. (2010). A minimally invasive technique to monitor valve-movement behavior in bivalves. Environ. Technol. 17, 501–507. doi: 10.1080/09593331708616411
Bell, E. C. (1995). Environmental and morphological influences on thallus temperature and desiccation of the intertidal alga Mastocarpus papillatus Kützing. J. Exp. Mar. Biol. Ecol. 191, 29–55.
Bertness, M. D. (1989). Intraspecific competition and facilitation in a northern acorn barnacle population. Ecology 70, 257–268. doi: 10.2307/1938431
Brewin, R. J. W., Smale, D. A., Moore, P. J., Dall'Olmo, G., Miller, P. I., Taylor, B. H., et al. (2018). Evaluating operational AVHRR sea surface temperature data at the coastline using benthic temperature loggers. Remote Sens. 10:925. doi: 10.3390/rs10060925
Broitman, B. R., Szathmary, P. L., Mislan, K. A. S., Blanchette, C. A., and Helmuth, B. (2009). Predator–prey interactions under climate change: the importance of habitat vs body temperature. Oikos 118, 219–224. doi: 10.1111/j.1600-0706.2008.17075.x
Brown, M. T. (1987). Effects of desiccation on photosynthesis of intertidal algae from a Southern New Zealand shore. Bot. Mar. 30, 121–127. doi: 10.1515/botm.1987.30.2.121
Buckley, L. B., Miller, E. F., and Kingsolver, J. G. (2013). Ectotherm thermal stress and specialization across altitude and latitude. Integr. Comp. Biol. 53, 571–581. doi: 10.1093/icb/ict026
Bulanon, M., Burks, T. F., and Alchanatis, V. (2009). Image fusion and thermal images for fruitdetection. Biosyst. Eng. 103, 12–22. doi: 10.1016/j.biosystemseng.2009.02.009
Caillon, R., Suppo, C., Casas, J., Woods, H. A., and Pincebourde, S. (2014). Warming decreases thermal heterogeneity of leaf surfaces: implications for behavioural thermoregulation by arthropods. Funct. Ecol. 28, 1449–1458. doi: 10.1111/1365-2435.12288
Cartwright, S. R., and Williams, G. A. (2012). Seasonal variation in utilization of biogenic microhabitats by littorinid snails on tropical rocky shores. Mar. Biol. 159, 2323–2332. doi: 10.1007/s00227-012-2017-3
Castillo, K. D., and Lima, F. P. (2010). Comparison of in situ and satellite-derived (MODIS-Aqua/Terra) methods for assessing temperatures on coral reefs. Limnol. Oceanogr. Methods 8, 107–117. doi: 10.4319/lom.2010.8.0107
Chan, B. K. K., Lima, F. P., Williams, G. A., Seabra, R., and Wang, H.-Y. (2016). A simplified biomimetic temperature logger for recording intertidal barnacle body temperatures. Limnol. Oceanogr. Methods 14, 448–455. doi: 10.1002/lom3.10103
Chapperon, C., and Seuront, L. (2011). Space-time variability in environmental thermal properties and snail thermoregulatory behaviour. Funct. Ecol. 25, 1040–1050. doi: 10.1111/j.1365-2435.2011.01859.x
Comeau, L., Mayrand, E., and Mallet, A. (2012). Winter quiescence and spring awakening of the Eastern oyster Crassostrea virginica at its northernmost distribution limit. Mar. Biol. 159, 2269–2279. doi: 10.1007/s00227-012-2012-8
Connell, J. H. (1961). The influence of interspecific competition and other factors on the distribution of the barnacle Chthamalus stellatus. Ecology 42, 710–723.
Davenport, J., and Davenport, J. L. (2005). Effects of shore height, wave exposure and geographical distance on thermal niche with of intertidal fauna. Mar. Ecol. Prog. Ser. 292, 41–50. doi: 10.2307/24868068
Dell, A. I., Pawar, S., and Savage, V. M. (2014). Temperature dependence of trophic interactions are driven by asymmetry of species responses and foraging strategy. J. Anim. Ecol. 84, 70–84. doi: 10.1111/1365-2656.12081
Denny, M. (2017). The fallacy of the average: on the ubiquity, utility and continuing novelty of Jensen's inequality. J. Exp. Biol. 220, 139–146. doi: 10.1242/jeb.140368
Denny, M. W. (1985). Wave forces on intertidal organisms: a case study. Limnol. Oceanogr. 30, 1171–1187. doi: 10.4319/lo.1985.30.6.1171
Denny, M. W., Dowd, W. W., Bilir, L., and Mach, K. J. (2011). Spreading the risk: small-scale body temperature variation among intertidal organisms and its implications for species persistence. J. Exp. Mar. Biol. Ecol. 400, 175–190. doi: 10.1016/j.jembe.2011.02.006
Denny, M. W., and Harley, C. D. G. (2006). Hot limpets: predicting body temperature in a conductance-mediated thermal system. J. Exp. Biol. 209, 2409–2419. doi: 10.1242/jeb.02257
Denny, M. W., and Helmuth, B. (2009). Confronting the physiological bottleneck: a challenge from ecomechanics. Integr. Comp. Biol. 49, 197–201. doi: 10.1093/icb/icp070
Díaz, F., Denisse, R. A., Salas, A., Galindo-Sanchez, C. E., Gonzalez, M. A., Sanchez, A., et al. (2015). Behavioral thermoregulation and critical thermal limits of giant keyhole limpet Megathura crenulate (Sowerby 1825) (Mollusca; Vetigastropoda). J. Therm. Biol. 54, 133–138. doi: 10.1016/j.jtherbio.2013.05.007
Diederich, C. M., and Pechenik, J. A. (2013). Thermal tolerance of Crepidula fornicata (Gastropoda) life history stages from intertidal and subtidal subpopulations. Mar. Ecol. Prog. Ser. 486, 173–187. doi: 10.3354/meps10355
Dong, Y.-W., Li, X.-X., Choi, F. M. P., Williams, G. A., Somero, G. N., and Helmuth, B. (2017). Untangling the roles of microclimate, behaviour and physiological polymorphism in governing vulnerability of intertidal snails to heat stress. Proc. R. Soc. B 284:20162367. doi: 10.1098/rspb.2016.2367
Doty, M. S. (1946). Critical tide factors that are correlated with the vertical distribution of marine algae and other organisms along the Pacific coast. Ecology 27, 315–328. doi: 10.2307/1933542
Drake, M. J., Miller, N. A., and Todgham, A. E. (2017). The role of stochastic thermal environments in modulating the thermal physiology of an intertidal limpet, Lottia digitalis. J. Exp. Biol. 220, 3072–3083. doi: 10.1242/jeb.159020
Dring, M. J., and Brown, F. A. (1982). Photosynthesis of intertidal brown algae during and after periods of emersion: a renewed search for physiological causes of zonation. Mar. Ecol. Prog. Ser. 8, 301–308. doi: 10.2307/24815108
Edson, E. C., and Patterson, M. R. (2015). “MantaRay: a novel autonomous sampling instrument for in situ measurements of environmental microplastic particle concentrations,”in OCEANS 2015 - MTS/IEEE Washington. Abstract retrieved from IEEE Xplore (Accession No. 15798895) (Washington, DC).
Ehrlén, J., and Morris, W. F. (2015). Predicting changes in the distribution and abundance of species under environmental change. Ecol. Lett. 18, 303–314. doi: 10.1111/ele.12410
Elvin, D. W., and Gonor, J. J. (1979). The thermal regime of an intertidal Mytilus californianus Conrad population on the Central Oregon Coast. J. Exp. Mar. Biol. Ecol. 39, 265–279.
Erasmus, T., and De Villiers, A. F. (1982). Ore dust pollution and body temperatures of intertidal animals. Mar. Pollut. Bull. 13, 30–32.
Etter, R. J. (1988). Physiological stress and color polymorphism in the intertidal snail Nucella lapillus. Evolution 4, 660–680.
Faye, E., Dangles, O., and Pincebourde, S. (2016a). Distance makes the difference in thermography for ecological studies. J. Therm. Biol. 56, 1–9. doi: 10.1016/j.jtherbio.2015.11.011
Faye, E., Rebaudo, F., Cajo, D. Y., Fraunié, S. C., and Dangles, O. (2016b). A toolbox for studying thermal heterogeneity across spatial scales: from unmanned aerial vehicle imagery to landscape metrics. Methods Ecol. Evol. 7, 437–446. doi: 10.1111/2041-210X.12488
Faye, E., Rebaudo, F., Carpio, C., Herrera, M., and Dangles, O. (2017). Does heterogeneity in crop canopy microclimates matter for pests? Evidence from aerial high-resolution thermography. Agric. Ecosyst. Environ. 246, 124–133. doi: 10.1016/j.agee.2017.05.027
Finke, G. R., Bozinovic, F., and Navarrete, S. A. (2009). A mechanistic model to study the thermal ecology of a southeastern Pacific dominant intertidal mussel and implications for climate change. Physiol. Biochem. Zool. 82, 303–313. doi: 10.1086/599321
Finke, G. R., Navarrete, S. A., and Bozinovic, F. (2007). Tidal regimes of temperate coasts and their influences on aerial exposure for intertidal organisms. Mar. Ecol. Prog. Ser. 343, 57–62. doi: 10.3354/meps06918
Fitzhenry, T., Halpin, P. M., and Helmuth, B. (2004). Testing the effects of wave exposure, site, and behavior on intertidal mussel body temperatures: applications and limits of temperature logger design. Mar. Biol. 145, 339–349. doi: 10.1007/s00227-004-1318-6
Fly, E. K., Monaco, C. J., Pincebourde, S., and Tullis, A. (2012). The influence of intertidal location and temperature on the metabolic cost of emersion in Pisaster ochraceus. J. Exp. Mar. Biol. Ecol. 422–423, 20–28. doi: 10.1016/j.jembe.2012.04.007
Geller, G. N., Halpin, P. N., Helmuth, B., Hestir, E. L., Skidmore, A., Abrams, M. J., et al. (2017). “Remote Sensing for Biodiversity,” in The GEO Handbook on Biodiversity Observation Networks, eds M. Walters and R. J. Scholes (Cham: Springer), 187–210. doi: 10.1007/978-3-319-27288-7_8
Gilman, S., Hayford, H., Craig, C., and Carrington, E. (2015). Body temperatures of an intertidal barnacle and two whelk predators in relation to shore height, solar aspect, and microhabitat. Mar. Ecol. Prog. Ser. 536, 77–88. doi: 10.3354/meps11418
Gilman, S. E., and Rognstad, R. L. (2018). Influence of food supply and shore height on the survival and growth of the barnacle Balanus glandula (Darwin). J. Exp. Mar. Biol. Ecol. 498, 32–38. doi: 10.1016/j.jembe.2017.10.006
Gleason, L. U., and Burton, R. S. (2016). Regional patterns of thermal stress and constitutive gene expression in the marine snail Chlorostoma funebralis in northern and southern California. Mar. Ecol. Prog. Ser. 556, 143–159. doi: 10.3354/meps11850
Gleason, L. U., Miller, L. P., Winnikoff, J. R., Somero, G. N., Yancey, P. H., Bratz, D., et al. (2017). Thermal history and gape of individual Mytilus californianus correlate with oxidative damage and thermoprotective osmolytes. J. Exp. Biol. 220, 4292–4304. doi: 10.1242/jeb.168450
Gunderson, A. R., Armstrong, E. J., and Stillman, J. H. (2016). Multiple stressors in a changing world: the need for an improved perspective on physiological responses to the dynamic marine environment. Annu. Rev. Mar. Sci. 8, 357–378. doi: 10.1146/annurev-marine-122414-033953
Hannah, L., Flint, L., Syphard, A. D., Moritz, M. A., Buckley, L. B., and McCullough, I. M. (2014). Fine-grain modeling of species' response to climate change: holdouts, stepping-stones, and microrefugia. Trends Ecol. Evol. 29, 390–397. doi: 10.1016/j.tree.2014.04.006
Hardin, D. D. (1968). A comparative study of lethal temperature in limpets Acmaea digitalis and Acmaea scabra. Veliger 11, 78–83.
Harley, C. D. G. (2008). Tidal dynamics, topographic orientation, and temperature-mediated mass mortalities on rocky shores. Mar. Ecol. Prog. Ser. 371, 37–46. doi: 10.3354/meps07711
Harley, C. D. G., and Helmuth, B. S. T. (2003). Local and regional scale effects of wave exposure, thermal stress, and absolute vs. effective shore level on patterns of intertidal zonation. Limnol. Oceanogr. 48, 1498–1508. doi: 10.4319/lo.2003.48.4.1498
Harley, C. D. G., and Lopez, J. P. (2003). The natural history, thermal physiology, and ecological impacts of intertidal mesopredators, Oedoparena spp. (Diptera: Dryomyzidae). Invertebr. Biol. 122, 61–73. doi: 10.1111/j.1744-7410.2003.tb00073.x
Hayford, H. A., Gilman, S. E., and Carrington, E. (2015). Foraging behavior minimizes heat exposure in a complex thermal landscape. Mar. Ecol. Prog. Ser. 518, 165–175. doi: 10.3354/meps11053
Hayford, H. A., O'Donnell, M., and Carrington, E. (2018). Radio tracking detects thermoregulation at a snail's pace. J. Exp. Mar. Biol. Ecol. 499, 17–25. doi: 10.1016/j.jembe.2017.12.005
Helmuth, B. (1999). Thermal biology of rocky intertidal mussels: quantifying body temperatures using climatological data. Ecology 80, 15–34.
Helmuth, B. (2002). How do we measure the environment? Linking intertidal thermal physiology and ecology through biophysics. Integr. Comp. Biol. 42, 837–845. doi: 10.1093/icb/42.4.837
Helmuth, B., Broitman, B. R., Blanchette, C. A., Gilman, S., Halpin, P., Harley, C. D. G., et al. (2006). Mosaic patterns of thermal stress in the rocky intertidal zone: implications for climate change. Ecol. Monogr. 76, 461–479. doi: 10.1890/0012-9615(2006)076[0461:MPOTSI]2.0.CO;2
Helmuth, B., Choi, F., Matzelle, A., Torossian, J. L., Morello, S. L., Mislan, K. A. S., et al. (2016). Long-term, high frequency in situ measurements of intertidal mussel bed temperatures using biomimetic sensors. Sci. Data 3:160087. doi: 10.1038/sdata.2016.87
Helmuth, B., Harley, C. D. G., Halpin, P. M., O'Donnell, M., Hofmann, G. E., and Blanchette, C. A. (2002). Climate change and latitudinal patterns of intertidal thermal stress. Science 298, 1015–1017. doi: 10.1126/science.1076814
Helmuth, B., Yamane, L., Lalwani, S., Matzelle, A., Tockstein, A., and Gao, N. (2011). Hidden signals of climate change in intertidal ecosystems: what (not) to expect when you are expecting. J. Exp. Mar. Biol. Ecol. 400, 191–199. doi: 10.1016/j.jembe.2011.02.004
Helmuth, B. S., and Hofmann, G. E. (2001). Microhabitats, thermal heterogeneity, and patterns of physiological stress in the rocky intertidal zone. Biol. Bull. 201, 374–384. doi: 10.1126/science.1076814
Helmuth, B. S. T. (1998). Intertidal mussel microclimates: predicting the body temperature of a sessile invertebrate. Ecol. Monogr. 68, 51–74.
Herring, S. C., Hoell, A., Hoerling, M. P., Kossin, J. P., Schreck III, C. J. and Stott, P. A. (2016). Explaining extreme events of 2015 from a climate perspective. Bull. Amer. Meteor. Soc. 97, S1–S145.
Hobday, A. J., Alexander, L. V., Perkins, S. E., Smale, D. A., Straub, S. C., Oliver, E. C. J., et al. (2016). A hierarchical approach to defining marine heatwaves. Prog. Oceanogr. 141, 227–238. doi: 10.1016/j.pocean.2015.12.014
Hoegh-Guldberg, O., Jacob, D., Taylor, M., Bindi, M., Brown, S., Camilloni, I., et al. (2018). “Impacts of 1.5°C global warming on natural and human systems,” in Global Warming of 1.5°C. An IPCC Special Report on the Impacts of Global Warming of 1.5°C Above Pre-industrial Levels and Related Global Greenhouse Gas Emission Pathways, in the Context of Strengthening the Global Response to the Threat of Climate Change, Sustainable Development, and Efforts to Eradicate Poverty, eds V. Masson-Delmotte, P. Zhai, H. O. Pörtner, D. Roberts, J. Skea, P. R. Shukla, A. Pirani, W. Moufouma-Okia, C. Péan, R. Pidcock, S. Connors, J. B. R. Matthews, Y. Chen, X. Zhou, M. I. Gomis, E. Lonnoy, T. Maycock, M. Tignor, and T. Waterfield. In Press.
Hunt, H. L. (1997). Structure and Dynamics of Intertidal Mussel (Mytilus trossulus, Mytilus edulis) Assemblages. PhD Thesis, Dalhousie University, Halifax, NS.
Hunt, H. L., and Scheibling, R. E. (2001). Patch dynamics of mussels on rocky shores: integrating process to understand pattern. Ecology 82, 3213–3231. doi: 10.2307/2679845
Hunt, H. L., and Scheibling, R. E. (2002). Movement and wave dislodgement of mussels on a wave-exposed rocky shore. Veliger 45, 273–277.
Hutchins, L. W. (1947). The bases for temperature zonation in geographical distribution. Ecol. Monogr. 17, 325–335.
Iacarella, J. C., and Helmuth, B. (2011). Experiencing the salt marsh environment through the foot of Littoraria irrorata: behavioral responses to thermal and desiccation stresses. J. Exp. Mar. Biol. Ecol. 409, 143–153. doi: 10.1016/j.jembe.2011.08.011
Jost, J., and Helmuth, B. (2007). Morphological and ecological determinants of body temperature of Geukensia demissa, the Atlantic Ribbed Mussel, and their effects on mussel mortality. Biol. Bull. 213, 141–151. doi: 10.2307/25066630
Kearney, M. (2006). Habitat, environment and niche: what are we modelling? Oikos 115, 186–191. doi: 10.1111/j.2006.0030-1299.14908.x
Kearney, M., Simpson, S. J., Raubenheimer, D., and Helmuth, B. (2010). Modelling the ecological niche from functional traits. Philos. Trans. R. Soc. B 365, 3469–3483. doi: 10.1098/rstb.2010.0034
Kearney, M. R., Matzelle, A., and Helmuth, B. (2012). Biomechanics meets the ecological niche: the importance of temporal data resolution. J. Exp. Biol. 215, 922–933. doi: 10.1242/jeb.059634
Kish, N. E., Helmuth, B., and Wethey, D. S. (2016). Physiologically grounded metrics of model skill: a case study estimating heat stress in intertidal populations. Conserv. Physiol. 4:cow038. doi: 10.1093/conphys/cow038
Kordas, R. L., Harley, C. D. G., and O'Connor, M. I. (2011). Community ecology in a warming world: the influence of temperature on interspecific interactions in marine systems. J. Exp. Mar. Biol. Ecol. 400, 218–226. doi: 10.1016/j.jembe.2011.02.029
Koussoroplis, A. M., Pincebourde, S., and Wacker, A. (2017). Understanding and predicting physiological performance of organisms in fluctuating and multifactorial environments. Ecol. Monogr. 87, 178–197. doi: 10.1002/ecm.1247
Kroeker, K. J., Sanford, E., Rose, J. M., Blanchette, C. A., Chan, F., Chavez, F. P., et al. (2016). Interacting environmental mosaics drive geographic variation in mussel performance and predation vulnerability. Ecol. Lett. 19, 771–779. doi: 10.1111/ele.12613
Lathlean, J. A., Ayre, D. J., Coleman, R. A., and Minchinton, T. E. (2014). Using biomimetic loggers to measure interspecific and microhabitat variation in body temperatures of rocky intertidal invertebrates. Mar. Freshw. Res. 66, 86–94. doi: 10.1071/MF13287
Lathlean, J. A., and Seuront, L. (2014). Infrared thermography in marine ecology: methods, previous applications, and future challenges. Mar. Ecol. Prog. Ser. 514, 263–277. doi: 10.3354/meps10995
Lathlean, J. A., Seuront, L., McQuaid, C. D., Terence, P. T. N., Zardi, G. I., and Nicastro, K. R. (2016a). Cheating the locals: invasive mussels steal and benefit from the cooling effect of indigenous mussels. PLoS ONE 11:e0152556. doi: 10.1371/journal.pone.0152556
Lathlean, J. A., Seuront, L., McQuaid, C. D., Terence, P. T. N., Zardi, G. I., and Nicastro, K. R. (2016b). Size and position (sometimes) matter: small-scale patterns of heat stress associated with two co-occurring mussels with different thermoregulatory behaviour. Mar. Biol. 163:189. doi: 10.1007/s00227-016-2966-z
Lathlean, J. A., Seuront, L., and Ng, T. P. T. (2017). On the edge: The use of infrared thermography in monitoring responses of intertidal organisms to heat stress. Ecol. Indic. 81, 567–577. doi: 10.1016/j.ecolind.2017.04.057
Leeuw, T., Boss, E., and Wright, D. (2013). In situ measurements of phytoplankton fluorescence using low cost electronics. Sensors 13, 7872–7883. doi: 10.3390/s130607872
Lewis, J. B. (1963). Environmental and tissue temperatures of some tropical intertidal marine animals. Biol. Bull. 124, 277–284.
Lima, F., Burnett, N., Helmuth, B., Kish, N., and Aveni-Deforge, K. (2011). “Monitoring the intertidal environment with biomimetic devices,” in Biomimetic Based Applications, ed A. George (InTech). doi: 10.5772/14153
Lima, F. P., and Wethey, D. S. (2009). Robolimpets: measuring intertidal body temperatures using biomimetic loggers. Limnol. Oceanogr. Methods 7, 347–353. doi: 10.4319/lom.2009.7.347
Lockridge, G., Dzwonkowski, B., Nelson, R., and Powers, S. (2016). Development of a low-cost Arduino-based sonde for coastal applications. Sensors 16:528. doi: 10.3390/s16040528
Lubchenco, J. (1980). Algal zonation in the New England rocky intertidal community: an experimental analysis. Ecology 61, 333–344.
Marshall, D. J., McQuaid, C. D., and Williams, G. A. (2010). Non-climatic thermal adaptation: implications for species' responses to climate warming. Biol. Lett. 6, 669–673. doi: 10.1098/rsbl.2010.0233
Marshall, D. J., Rezende, E. L., Baharuddin, N., Choi, F., and Helmuth, B. (2015). Thermal tolerance and climate warming sensitivity in tropical snails. Ecol. Evol. 5, 5905–5919. doi: 10.1002/ece3.1785
McAfee, D., Bishop, M. J., Yu, T. N., and Williams, G. A. (2018). Structural traits dictate abiotic stress amelioration by intertidal oysters. Funct. Ecol. 27, 249. doi: 10.1111/1365-2435.13210
Meola, C., and Carlomagno, G. M. (2004). Recent advances in the use of infrared thermography. Meas. Sci. Technol. 15, 27–58. doi: 10.1088/0957-0233/15/9/R01
Mesinger, F., DiMego, G., Kalnay, E., Mitchell, K., Shafran, P. C., Ebisuzaki, W., et al. (2006). North American regional reanalysis. Bull. Am. Meteorol. Soc. 87:3. doi: 10.1175/BAMS-87-3-343
Miller, L. P., and Denny, M. W. (2016). Importance of behavior and morphological traits for controlling body temperature in Littorinid snails. Biol. Bull. 220, 209–223. doi: 10.1086/BBLv220n3p209
Miller, L. P., and Dowd, W. W. (2017). Multimodal in situ datalogging quantifies inter-individual variation in thermal experience and persistent origin effects on gaping behavior among intertidal mussels (Mytilus californianus). J. Exp. Biol. 220, 4305–4319. doi: 10.1242/jeb.164020
Mislan, K. A., and Wethey, D. S. (2011). Gridded meteorological data as a resource for mechanistic macroecology in coastal environments. Ecol. Appl. 21, 2678–2690. doi: 10.1890/10-2049.1
Mislan, K. A., and Wethey, D. S. (2015). A biophysical basis for patchy mortality during heat waves. Ecology 96, 902–907. doi: 10.1890/14-1219.1
Mislan, K. A. S., Helmuth, B., and Wethey, D. S. (2014). Geographical variation in climatic sensitivity of intertidal mussel zonation. Glob. Ecol. Biogeogr. 23, 744–756. doi: 10.1111/geb.12160
Mitton, J. B. (1977). Shell color and pattern variation in Mytilus edulis and its adaptive significance. Chesapeake Sci. 18, 387–390. doi: 10.2307/1350595
Monaco, C. J., and Helmuth, B. (2011). Tipping points, thresholds and the keystone role of physiology in marine climate change research. Adv. Mar. Biol. 60, 123–160. doi: 10.1016/B978-0-12-385529-9.00003-2
Monaco, C. J., Wethey, D. S., Gulledge, S., and Helmuth, B. (2015). Shore-level size gradients and thermal refuge use in the predatory sea star Pisaster ochraceus: the role of environmental stressors. Mar. Ecol. Prog. Ser. 539, 191–205. doi: 10.3354/meps11475
Monaco, C. J., Wethey, D. S., and Helmuth, B. (2016). Thermal sensitivity and the role of behavior in driving an intertidal predator–prey interaction. Ecol. Monogr. 86, 429–447. doi: 10.1002/ecm.1230
Montalto, V., Sarà, G., Ruti, P. M., Dell'Aquila, A., and Helmuth, B. (2014). Testing the effects of temporal data resolution on predictions of the effects of climate change on bivalves. Ecol. Model. 278, 1–8. doi: 10.1016/j.ecolmodel.2014.01.019
Montanholi, Y. R., Swanson, K. C., Palme, R., Schenkel, F. S., McBride, B. W., Lu, D., et al. (2010). Assessing feed efficiency in beef steers through feeding behaviour, infrared thermography and glucocorticoids. Animal 4, 692–701. doi: 10.1017/S1751731109991522
Nagai, K., Honjo, T., Go, J., Yamashita, H., and Seok Jin, O. (2006). Detecting the shellfish killer Heterocapsa circularisquama (Dynophyceae) by measuring bivalve valve activity with a hall element sensor. Aquaculture 255, 395–401. doi: 10.1016/j.aquaculture.2005.12.018
Ng, J. S. S., and Williams, G. A. (2006). Intraspecific variation in foraging behaviour: influence of shore height on temporal organization of activity in the chiton Acanthopleura japonica. Mar. Ecol. Prog. Ser. 321, 183–192. doi: 10.3354/meps321183
Ng, T. P. T., Lau, S. L. Y., Seuront, L., Davies, M. S., Stafford, R., Marshall, D. J., et al. (2017). Linking behaviour and climate change in intertidal ectotherms: insights from littorinid snails. J. Exp. Mar. Biol. Ecol. 492, 121–131. doi: 10.1016/j.jembe.2017.01.023
Nicastro, K. R., Zardi, G. I., McQuaid, C. D., Pearson, G. A., and Serrão, E. A. (2012). Love thy neighbour: group properties of gaping behaviour in mussel aggregations. PLoS ONE 7:e47382. doi: 10.1371/journal.pone.0047382
Okamura, B. (1986). Group living and the effects of spatial position in aggregations of Mytilus edulis. Oecologia 69, 341–347. doi: 10.1007/BF00377054
Olabarria, C., Gestoso, I., Lima, F. P., Vázquez, E., Comeau, L. A., Gomes, F., et al. (2016). Response of two Mytilids to a heatwave: the complex interplay of physiology, behaviour and ecological interactions. PLoS ONE 11:e0164330. doi: 10.1371/journal.pone.0164330.3
Paine, R. T. (1994). Marine Rocky Shores and Community Ecology: An Experimentalist's Perspective. Oldendorf; Luhe: Ecology Institute.
Parsons, K. (2014). Human Thermal Environments: The Effects of Hot, Moderate, and Cold Environments on Human Health, Comfort, and Performance. Boca Raton, FL: CRC Press.
Pearson, G. A., Leston, A. L., and Mota, C. (2009). Frayed at the edges: selective pressure and adaptive response to abiotic stressors are mismatched in low diversity edge populations. J. Ecol. 97, 450–462. doi: 10.1111/j.1365-2745.2009.01481.x
Pershing, A. J., Alexander, M. A., Hernandez, C. M., Kerr, L. A., Le Bris, A., Mills, K. E., et al. (2015). Slow adaptation in the face of rapid warming leads to collapse of the Gulf of Maine cod fishery. Science 350:6262, 809–812. doi: 10.1126/science.aac9819
Petchey, O. L., Pontarp, M., Massie, T. M., Kéfi, S., Ozgul, A., Weilenmann, M., et al. (2015). The ecological forecast horizon, and examples of its uses and determinants. Ecol. Lett. 18, 597–611. doi: 10.1111/ele.12443
Peters, M. K., Hemp, A., Appelhans, T., Behler, C., Classen, A., Detsch, F., et al. (2016). Predictors of elevational biodiversity gradients change from single taxa to the multi-taxa community level. Nat. Commun. 7:13736. doi: 10.1038/ncomms13736
Pfister, C. A., Wootton, J. T., and Neufeld, C. J. (2007). The relative roles of coastal and oceanic processes in determining physical and chemical characteristics of an intensively sampled nearshore system. Limnol. Oceanogr. 52, 1767–1775. doi: 10.4319/lo.2007.52.5.1767
Pincebourde, S., and Casas, J. (2006). Leaf miner-induced changes in leaf transmittance cause variations in insect respiration rates. J. Insect Physiol. 52, 194–201. doi: 10.1016/j.jinsphys.2005.10.004
Pincebourde, S., Sanford, E., Casas, J., and Helmuth, B. (2012). Temporal coincidence of environmental stress events modulates predation rates. Ecol. Lett. 15, 680–688. doi: 10.1111/j.1461-0248.2012.01785.x
Pincebourde, S., Sanford, E., and Helmuth, B. (2008). Interaction between underwater and aerial body temperatures in influencing a top predator feeding rate in the intertidal. Comp. Biochem. Physiol. Part A Mol. Integr. Physiol. 150:3. doi: 10.1016/j.cbpa.2008.04.184
Pincebourde, S., Sanford, E., and Helmuth, B. (2009). An intertidal sea star adjusts thermal inertia to avoid extreme body temperatures. Am. Nat. 174, 890–897. doi: 10.1086/648065
Place, S. P., O'Donnell, M. J., and Hofmann, G. E. (2008). Gene expression in the intertidal mussel Mytilus californianus: physiological response to environmental factors on a biogeographic scale. Mar. Ecol. Prog. Ser. 356, 1–14. doi: 10.3354/meps07354
Porter, J., Arzberger, P., Braun, H., Bryant, P., Gage, S., Hansen, T., et al. (2005). Wireless sensor networks for ecology. BioScience 55, 561–572. doi: 10.1641/0006-3568(2005)055[0561:WSNFE]2.0.CO;2
Porter, W. P., Budaraju, S., Stewart, W. E., and Ramankutty, N. (2000). Calculating climate effects on birds and mammals: impacts on biodiversity, conservation, population parameters, and global community structure. Integr. Comp. Biol. 40, 597–630. doi: 10.1093/icb/40.4.597
Porter, W. P., and Gates, D. M. (1969). Thermodynamic Equilibria of Animals with Environment. Ecol. Monogr. 39, 227–244. doi: 10.2307/1948545
Porter, W. P., Mitchell, J. W., Beckman, W. A., and DeWitt, C. B. (1973). Behavioral implications of mechanistic ecology. Oecologia 13, 1–54.
Pörtner, H. O., Peck, L. S., and Hirse, T. (2006). Hyperoxia alleviates thermal stress in the Antarctic bivalve, Laternula elliptica: evidence for oxygen limited thermal tolerance. Polar Biol. 29, 688–693. doi: 10.1007/s00300-005-0106-1
Potter, K. A., Arthur Woods, H., and Pincebourde, S. (2013). Microclimatic challenges in global change biology. Glob. Change Biol. 19, 2932–2939. doi: 10.1111/gcb.12257
Robert, K. A., and Thompson, M. B. (2003). Reconstructing Thermochron iButtons to reduce size and weight as a new technique in the study of small animal thermal biology. Herpetol. Rev. 34, 130–132.
Robinet, C., Rousselet, J., Pineau, P., Miard, F., and Roques, A. (2013). Are heat waves susceptible to mitigate the expansion of a species progressing with global warming?. Ecol. Evol. 3, 2947–2957. doi: 10.1002/ece3.690
Sarà, G., Kearney, M., and Helmuth, B. (2011). Combining heat-transfer and energy budget models to predict thermal stress in Mediterranean intertidal mussels. Chem. Ecol. 27, 135–145. doi: 10.1080/02757540.2011.552227
Scheffers, B. R., Edwards, D. P., Diesmos, A., Williams, S. E., and Evans, T. A. (2013). Microhabitats reduce animal's exposure to climate extremes. Glob. Change Biol. 20, 495–503. doi: 10.1111/gcb.12439
Scherrer, D., and Körner, C. (2010). Infra-red thermometry of alpine landscapes challenges climatic warming projections. Glob. Change Biol. 16, 2602–2613. doi: 10.1111/j.1365-2486.2009.02122.x
Scherrer, D., and Körner, C. (2011). Topographically controlled thermal-habitat differentiation buffers alpine plant diversity against climate warming. J. Biogeogr. 38, 406–416. doi: 10.1111/j.1365-2699.2010.02407.x
Schneider, K. R., and Helmuth, B. (2007). Spatial variability in habitat temperature may drive patterns of selection between an invasive and native mussel species. Mar. Ecol. Prog. Ser. 339, 157–167. doi: 10.3354/meps339157
Schneider, K. R., Wethey, D. S., Helmuth, B. S. T., and Hilbish, T. J. (2005). Implications of movement behavior on mussel dislodgement: exogenous selection in a Mytilus spp. hybrid zone. Mar. Biol. 146, 333–343. doi: 10.1007/s00227-004-1446-z
Seabra, R., Wethey, D. S., Santos, A. M., and Lima, F. P. (2011). Side matters: microhabitat influence on intertidal heat stress over a large geographical scale. J. Exp. Mar. Biol. Ecol. 400, 200–208. doi: 10.1016/j.jembe.2011.02.010
Sears, M. W., Angilletta, M. J., Schuler, M. S., Borchert, J., Dilliplane, K. F., Stegman, M., et al. (2016). Configuration of the thermal landscape determines thermoregulatory performance of ectotherms. Proc. Natl. Acad. Sci. U.S.A. 113, 10595–10600. doi: 10.1073/pnas.1604824113
Sinclair, B. J., Marshall, K. E., Sewell, M. A., Levesque, D. L., Willett, C. S., and Slotsbo, S. (2016). Can we predict ectotherm responses to climate change using thermal performance curves and body temperatures? Ecol. Lett. 19, 1372–1385. doi: 10.1111/ele.12686
Smale, D. A., and Wernberg, T. (2009). Satellite-derived SST data as a proxy for water temperature in nearshore benthic ecology. Mar. Ecol. Prog. Ser. 387, 27–37. doi: 10.3354/meps08132
Somero, G. N. (2002). Thermal physiology and vertical zonation of intertidal animals: optima, limits, and costs of living. Integr. Comp. Biol. 42, 780–789. doi: 10.1093/icb/42.4.780
Somero, G. N. (2010). The physiology of climate change: how potentials for acclimatization and genetic adaptation will determine ‘winners' and ‘losers'. J. Exp. Biol. 213, 912–920. doi: 10.1242/jeb.037473
Sorte, C. J., Davidson, V. E., Franklin, M. C., Benes, K. M., Doellman, M. M., Etter, R. J., et al. (2016). Long-term declines in an intertidal foundation species parallel shifts in community composition. Glob. Change Biol. 23, 341–352. doi: 10.1111/gcb.13425
Southward, A. J. (1958). Note on the temperature tolerances of some intertidal animals in relation to environmental temperatures and geographical distribution. J. Mar. Biol. Assoc. U.K. 37, 49–66. doi: 10.1017/S0025315400014818
Stenseth, N. C., Mysterud, A., Ottersen, G., Hurrell, J. W., Chan, K. S., and Lima, M. (2002). Ecological effects of climate fluctuations. Science 297, 1292–1296. doi: 10.1126/science.1071281
Stillman, J., and Somero, G. (1996). Adaptation to temperature stress and aerial exposure in congeneric species of intertidal porcelain crabs (genus Petrolisthes): correlation of physiology, biochemistry and morphology with vertical distribution. J. Exp. Biol. 199, 1845–1855.
Sunday, J. M., Bates, A. E., and Dulvy, N. K. (2011). Global analysis of thermal tolerance and latitude in ectotherms. Proc. R. Soc. Lond. Ser. B 278, 1823–1830. doi: 10.1098/rspb.2010.1295
Sunday, J. M., Bates, A. E., Kearney, M. R., Colwell, R. K., Dulvy, N. K., Longino, J. T., et al. (2014). Thermal-safety margins and the necessity of thermoregulatory behavior across latitude and elevation. Proc. Natl. Acad. Sci. U.S.A. 111, 5610–5615. doi: 10.1073/pnas.1316145111
Sunday, J. M., Pecl, G. T., Frusher, S., Hobday, A. J., Hill, N., Holbrook, N. J., et al. (2015). Species traits and climate velocity explain geographic range shifts in an ocean-warming hotspot. Ecol. Lett. 18, 944–953. doi: 10.1111/ele.12474
Szathmary, P. L., Helmuth, B., and Wethey, D. S. (2009). Climate change in the rocky intertidal zone: predicting and measuring the body temperature of a keystone predator. Mar. Ecol. Prog. Ser. 374, 43–56. doi: 10.3354/meps07682
Thomas, F. I. M. (1987). The Hot and Cold of Life on Rocks: Determinants of Body Temperature in the Northern Rock Barnacle, Semibalanus Balanoides. Master's Thesis, Brown University, Providence, RI.
Torossian, J. L., Kordas, R. L., and Helmuth, B. (2016). Cross-Scale approaches to forecasting biogeographic responses to climate change. Adv. Ecol. Res. 55, 371–433. doi: 10.1016/bs.aecr.2016.08.003
Tsuchiya, M. (1983). Mass mortality in a population of the mussel Mytilus edulis L. caused by high temperature on rocky shores. J. Exp. Mar. Biol. Ecol. 66, 101–111. doi: 10.1016/0022-0981(83)90032-1
Van Alstyne, K. L., and Olson, T. K. (2014). Estimating variation in surface emissivities of intertidal macroalgae using an infrared thermometer and the effects on temperature measurements. Mar. Biol. 161, 1409–1418. doi: 10.1007/s00227-014-2429-3
Vermeij, G. J. (1971). Temperature relationships of some tropical Pacific intertidal gastropods. Mar. Biol. 10, 308–314.
Wethey, D. S. (1983). Geographic limits and local zonation: the barnacles Semibalanus (Balanus) and Chthamalus in New England. Biol. Bull. 165, 330–341.
Wethey, D. S. (1984). Sun and shade mediate competition in the barnacles Chthamalus and Semibalanus: a field experiment. Biol. Bull. 167, 176–185.
Wethey, D. S. (2002). Biogeography, competition, and microclimate: the barnacle Chthamalus fragilis in New England. Integr. Comp. Biol. 42, 872–880. doi: 10.1093/icb/42.4.872
Wethey, D. S., Brin, L. D., Helmuth, B., and Mislan, K. A. S. (2011). Predicting intertidal organism temperatures with modified land surface models. Ecol. Modell. 222, 3568–3576. doi: 10.1016/j.ecolmodel.2011.08.019
Williams, G. A., De Pirro, M., Cartwright, S., Khangura, K., Ng, W. C., Leung, P. T. Y., et al. (2011). Come rain or shine: the combined effects of physical stresses on physiological and protein-level responses of an intertidal limpet in the monsoonal tropics. Funct. Ecol. 25, 101–110. doi: 10.1111/j.1365-2435.2010.01760.x
Williams, G. A., De Pirro, M., Leung, K. M. Y., and Morritt, D. (2005). Physiological responses to heat stress on a tropical shore: the benefits of mushrooming behaviour in the limpet Cellana grata. Mar. Ecol. Prog. Ser. 292, 213–224. doi: 10.3354/meps292213
Williams, G. A., and Morritt, D. (1995). Habitat partitioning and thermal tolerance in a tropical limpet, Cellana grata. Mar. Ecol. Prog. Ser. 124, 89–103. doi: 10.3354/meps124089
Woodin, S. A., Hilbish, T. J., Helmuth, B., Jones, S. J., and Wethey, D. S. (2013). Climate change, species distribution models, and physiological performance metrics: predicting when biogeographic models are likely to fail. Ecol. Evol. 3, 3334–3346. doi: 10.1002/ece3.680
Woods, H. A., Dillon, M. E., and Pincebourde, S. (2015). The roles of microclimatic diversity and of behavior in mediating the responses of ectotherms to climate change. J. Therm. Biol. 54, 86–97. doi: 10.1016/j.jtherbio.2014.10.002
World Meteorological Organization (2008). Guide to Meteorological Instruments and Methods of Observation, 7th Edn. WMO Report No. 8, I.2–3.
Zardi, G. I., Nicastro, K. R., McQuaid, C. D., Hancke, L., and Helmuth, B. (2010). The combination of selection and dispersal helps explain genetic structure in intertidal mussels. Oecologia 165, 947–958. doi: 10.1007/s00442-010-1788-9
Keywords: behavior, climate change, biomimetic sensor, intertidal, logging, thermal physiology
Citation: Judge R, Choi F and Helmuth B (2018) Recent Advances in Data Logging for Intertidal Ecology. Front. Ecol. Evol. 6:213. doi: 10.3389/fevo.2018.00213
Received: 31 July 2018; Accepted: 28 November 2018;
Published: 18 December 2018.
Edited by:
Thomas Wassmer, Siena Heights University, United StatesReviewed by:
Bernardo R. Broitman, Centro de Estudios Avanzados en Zonas Áridas (CEAZA), ChileElvira S. Poloczanska, Helmholtz-Gemeinschaft Deutscher Forschungszentren (HZ), Germany
Copyright © 2018 Judge, Choi and Helmuth. This is an open-access article distributed under the terms of the Creative Commons Attribution License (CC BY). The use, distribution or reproduction in other forums is permitted, provided the original author(s) and the copyright owner(s) are credited and that the original publication in this journal is cited, in accordance with accepted academic practice. No use, distribution or reproduction is permitted which does not comply with these terms.
*Correspondence: Brian Helmuth, b.helmuth@northeastern.edu