- State Key Laboratory of Veterinary Biotechnology, Harbin Veterinary Research Institute, Chinese Academy of Agricultural Sciences, Harbin, China
Probiotics exert a variety of beneficial effects, including maintaining homeostasis and the balance of intestinal microorganisms, activating the immune system, and regulating immune responses. Due to the beneficial effects of probiotics, a wide range of probiotics have been developed as probiotic agents for animal and human health. Viral diseases cause serious economic losses to the livestock every year and remain a great challenge for animals. Moreover, strategies for the prevention and control of viral diseases are limited. Viruses enter the host through the skin and mucosal surface, in which are colonized by hundreds of millions of microorganisms. The antiviral effects of probiotics have been proved, including modulation of chemical, microbial, physical, and immune barriers through various probiotics, probiotic metabolites, and host signaling pathways. It is of great significance yet far from enough to elucidate the antiviral mechanisms of probiotics. The major interest of this review is to discuss the antiviral effects and underlying mechanisms of probiotics and to provide targets for the development of novel antivirals.
Introduction
Humans and animals are colonized by hundreds of millions of microorganisms, which are far more than the number of host cells, and some argue that the number of microorganisms is comparable to host cells (Egert and Simmering, 2016). A vast majority of these microorganisms coinhabit within the gastrointestinal tract (Sender et al., 2016; Heintz-Buschart and Wilmes, 2018; Mishra et al., 2021). Probiotics are essential to maintain the balance of intestinal microorganisms. The Food and Agriculture Organization (FAO) and the World Health Organization (WHO) define probiotics as probiotics are live microorganisms, which when administered in adequate amounts confer health benefits on the host (WHO/FAO, 2002; Drago et al., 2010; Kahouli et al., 2013). Probiotics are known for their beneficial effects, mainly including maintaining the balance of intestinal microorganisms, activating the immune system, and regulating immune response (Hao et al., 2015; Gasbarrini et al., 2016; Kim et al., 2019). Due to their beneficial effects, a variety of probiotics have been widely used for promoting animal and human health in recent years (Kim et al., 2019; Teame et al., 2020; Rad et al., 2021). Several species of probiotics and their effects as probiotic agents have been evaluated. Lactobacillus, Bifidobacterium, and yeast are widely used (Al-Ghazzewi and Tester, 2016; Wieers et al., 2020; Di Pierro and Pane, 2021; Giannakou et al., 2021).
Viral diseases remain a great challenge for humans and animals (VanderWaal and Deen, 2018). Although vaccination is the most important option to prevent viral infections, differences between evolving epidemics and vaccines available make vaccination less effective. Moreover, there are no available vaccines for emerging or re-emerging viruses. Viruses enter the host through the skin and mucosal surface, where large numbers of microorganisms are colonized (Schmidt et al., 2018; Lunjani et al., 2019; Xu and Li, 2019; Peixoto et al., 2021). Probiotics are critical for the host to inhibit incoming pathogen infections (Lloyd-Price et al., 2016; Piewngam et al., 2018). A large body of literature has shown that probiotics have antiviral effects (Pradhan et al., 2021; Salaris et al., 2021). In the present review, we summarized and discussed the mechanisms of antiviral effects of probiotics, which opens up new perspectives on the use of antiviral strategies, provides new targets for the research and development of antivirals, and helps to provide a clearer background for probiotics-based antivirals.
Probiotics Contribute to the Chemical Barrier to Exert Antiviral Effects
The chemical barrier is the first barrier that pathogenic microorganisms encounter after they invade the intestine. Pathogens must break through the chemical barrier before they get access to the epithelium. Probiotics utilize carbohydrates in the host to produce various metabolites, including antimicrobial peptides (AMPs), hydrogen peroxide (H2O2), lactic acid, short-chain fatty acids (SCFAs), and extracellular vesicles (EV) (Knezevic et al., 2005; Gosmann et al., 2017; Vieira et al., 2017; Tyssen et al., 2018; Nahui et al., 2019). It has been revealed that the metabolites released by probiotics display important protective activities to inhibit viral infections. These metabolites form a micro-environment that is not conducive to viral reproduction (Figure 1A).
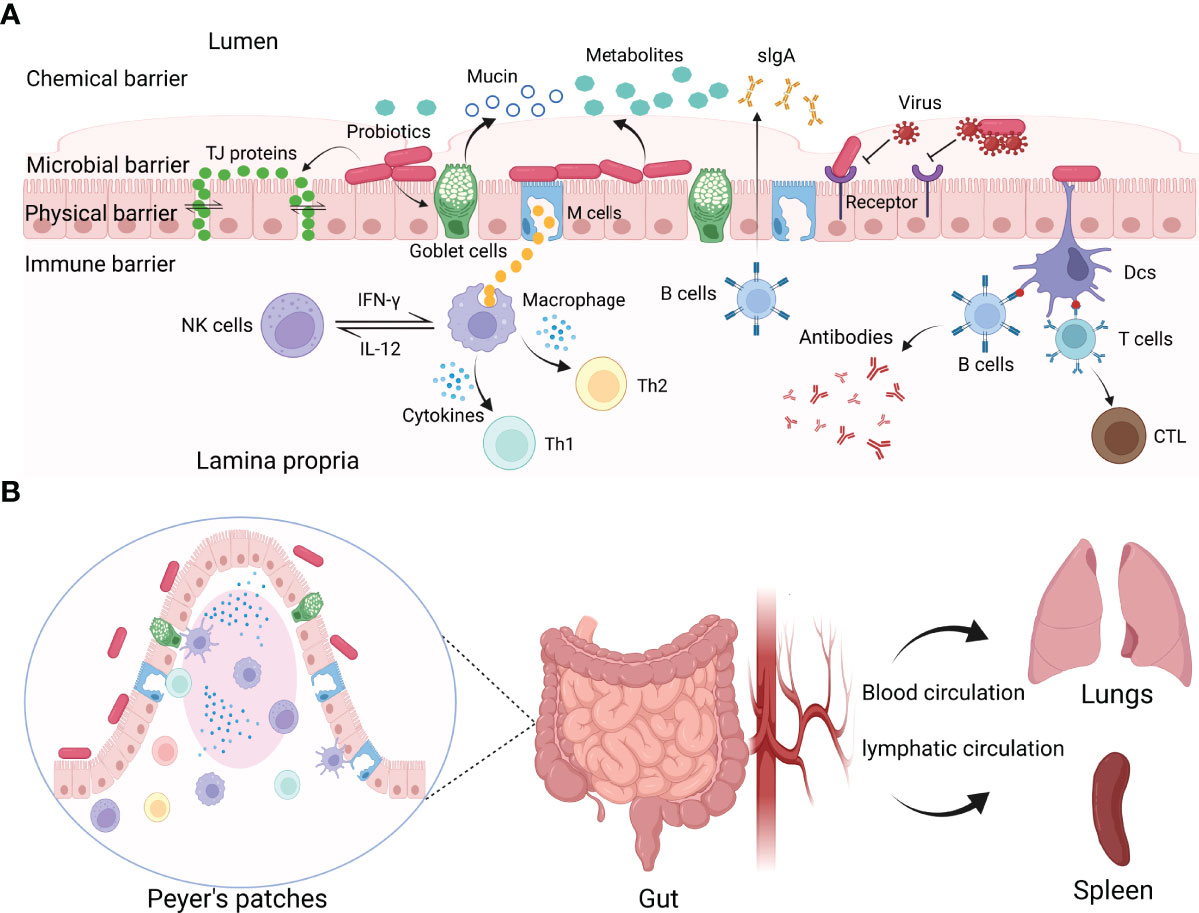
Figure 1 The interactions between probiotics and viruses. (A) Probiotics exert protective effects on viral infections by constituting or modulating chemical, microbial, physical, and immune barriers. Probiotics can produce antiviral metabolites, block virus invasion by binding with viruses or competing for the entry receptors, regulating the tight junctions of IECs, and modulating mucosal immune responses. (B) Probiotics modulate systemic immune responses.
AMPs are known as short, positively charged, and amphipathic peptides with a broad scope of antimicrobial activity against bacteria, viruses, fungi, and protozoa (Boparai and Sharma, 2020; Bosch and Zasloff, 2021). In recent years, AMPs have attracted interests due to their therapeutic potential. Bacteriocins are the AMPs derived from bacteria (Fry, 2018). While the antibacterial effects of bacteriocins are somewhat deciphered, their antiviral effects remain to be further studied. Enterocin B produced by Enterococcus was shown to inhibit the cytopathic effects of influenza virus (H1N1 and H3N2) in Madin-Darby canine kidney cells (Ermolenko et al., 2019). Presumably, bacteriocins could lead to the aggregation of viral particles, block viral particles through binding to the host cell receptors or inhibiting key steps in the viral replication cycle (Wachsman et al., 2003). Bacteriocins are structurally diverse with similar antiviral mechanisms.
The probiotics that play protective roles in the vaginal mucosa are dominated by the Lactobacillus genus. Numerous studies have demonstrated that metabolites released by Lactobacillus have antiviral effects on human immunodeficiency virus (HIV). H2O2, one of the metabolites produced by L. acidophilus, is toxic to many viruses, including HIV. It is reported that the prevalence of H2O2-producing Lactobacilli was lower in HIV-positive compared with HIV-negative women (Knezevic et al., 2005). Another study showed that H2O2 can inhibit HIV replication in CEM (a human T-lymphoblastic cell line) cells (Klebanoff and Coombs, 1991). The H2O2-producing probiotics present in the vagina of healthy women have been suggested as some bacteria that maintain health. Disruption of HIV by the probiotics is caused by the toxicity of H2O2. Notably, there are significant differences in H2O2 production among different Lactobacilli strains, and the same strain may not even produce H2O2 by changing the culture conditions.
Previous studies on the antiviral effects of lactobacillus-based probiotics have focused on H2O2, but recent studies have shown that lactic acid is a major antiviral factor produced by Lactobacilli in the vaginal mucosa (O'Hanlon et al., 2011; Liu et al., 2021). Lactic acid, a final product of carbohydrates, is an important metabolite of Lactobacillus with anti-HIV activity (Gosmann et al., 2017; Nahui et al., 2017; Tyssen et al., 2018). The mechanism of anti-HIV effects of lactic acid-producing bacteria is likely multifactorial. First of all, lactic acid may bind to the viruses and affect the function of the virus, thereby restricting the viruses from invading host cells. Secondly, lactic acid may disrupt the viral envelope and lyse the virions of enveloped viruses (Tachedjian et al., 2017). Besides HIV, lactic acid also has a significant inhibitory effect on herpes simplex virus (HSV) (Conti et al., 2009). It has been confirmed that lactic acid successfully interferes with viral replication in cells and that both the virucidal activity and the inhibition of replication were correlated to acidic pH values.
Microorganisms in the colon produce SCFAs by using dietary carbohydrates as substrates, primarily acetate, propionate, and butyrate, as final products (Wong et al., 2006). The ability to produce SCFAs by both Lactobacilli and Bifidobacteria is highlighted (LeBlanc et al., 2017). Probiotics have been reported to regulate butyrate metabolism resulting in an enhancement of host resistance to influenza virus infection. The correlation analysis revealed that the butyrate was negatively related to viral loading (Lu et al., 2021). SCFAs produced by probiotics are also potential regulatory effectors of epithelial proliferation in the gut (Wang et al., 2018). In addition, SCFAs can not only act on the gut but also transport to distant sites through blood circulation as a chemical signal for communication. For example, the abundance of SCFAs-producing bacteria and serum SCFA levels increased in respiratory syncytial virus (RSV)-infected mice after administration of a probiotic mixture (LeBlanc et al., 2017). Likewise, similar findings have been reported on antiviral responses induced by commensal microbiota (Antunes et al., 2019).
Lactobacillus can produce extracellular vehicles (EVs), along with other bacterial and mammalian cells, which are essential for the communication between bacteria and cells. It has been proved that L. crispatus BC3 and L. gasseri BC12 protected human cervicovaginal and tonsillar tissues from HIV-1 infection in vitro by producing EVs (Nahui et al., 2019). EVs inhibit HIV-1 infection by reducing HIV-1 entry/attachment to target cells. In addition, probiotics can refine the barrier function by promoting goblet cells to secrete mucin to form a mucin layer above the intestinal epithelium, protecting the mucosa from virus attachment (Engevik et al., 2019; Lorella et al., 2020). For instance, probiotics mixture VSL#3 induced mucin expression in colonic epithelial cells and prompted goblet cells to secrete mucin against pathogen attachment (Caballero-Franco et al., 2007). Relevant studies have confirmed that probiotics can secrete extracellular proteins, weaken the adhesion of pathogens, and protect the intestinal cells (Liu et al., 2020). Viruses are intracellular pathogens that require the host machinery to replicate. These metabolites exert antiviral effects by preventing the viruses from attaching to host cells or directly killing the viruses, which is a broad-spectrum antiviral mechanism. In summary, probiotics enhance the chemical barrier to maintain host health by producing antiviral metabolites or stimulating goblet cells to produce mucin.
Probiotics Constitute the Microbial Barrier to Exert Antiviral Effects
Besides producing some antiviral metabolites, probiotics can also inhibit viruses directly by interacting with viruses or competing for the cellular receptors to inhibit virus entry into host cells (Figure 1A) (Lievin-Le and Servin, 2014).
Several studies have revealed that probiotics can inhibit the infection of vesicular stomatitis virus (VSV) in vitro. It was suggested that pre-incubation of cell monolayers with probiotics, or probiotics with VSV can decrease VSV titer (Botic et al., 2007). A possible mechanism of inhibiting VSV replication is that probiotics compete with the virus for cell binding and interference with virus attachment or entry. Another possible mechanism is that probiotics can trap VSV specifically or nonspecifically. The above are two possible mechanisms by which probiotics inhibit VSV infection, and more mechanisms remain to be elucidated. B. subtilisis is one of the probiotics with excellent antiviral and immune regulation properties. The current study proved that B. subtilisis OKB105 inhibited the entry of transmissible gastroenteritis virus (TGEV) into the intestinal porcine epithelial cell line (IPEC-J2) by competing for the entry receptors (Wang et al., 2013). Another study revealed that Enterococcus faecium NCIMB 10415 was efficient to inhibit swine influenza virus (SIV) infection by the direct interaction between the SIV and probiotics (Wang et al., 2013). Published literature analysis indicated that the antiviral effects of probiotics are strain-dependent. Besides, the probiotics also limit pathogen access to nutrient resources. Furthermore, studies have revealed that the mucosal immune system of germ-free mice is immature due to the lack of microorganism colonization of their mucosal surfaces (Shen et al., 2014). The colonization of microorganisms also promotes the maturation of the host mucosal immune system. The microbial barrier constituted by probiotics is very important to inhibit viral infections and provides an insight into the development of novel antiviral strategies in the future.
Probiotics Strengthen the Physical Barrier to Exert Antiviral Effects
The physical barrier is composed of epidermides and mucosae (Karst, 2016). Probiotics form a defensive barrier against the invasion of viruses by enhancing the tight junctions (TJs) between intestinal epithelial cells (IECs) (Figure 1A).
TJs are an important form of intercellular connection and the most important structure of the mucosal barrier (Otani and Furuse, 2020). IECs are interconnected by the TJ proteins, which are the membrane protein complex with defensive functions formed between epithelial cells and endothelial cells (Gunzel and Fromm, 2012). Different species of probiotics have been reported to protect the host from viral infections by regulating the production of the TJ proteins (Kanmani and Kim, 2019; Lorella et al., 2020). Lactiplantibacillus (Lp.) plantarum, Weissella cibaria, or Latilactobacillus (Ll.) sakei could protect IECs from viral infections by maintaining the activity of TJ proteins. The TJ proteins between epidermal cells are necessary to maintain the integrity of the mucosal barrier. An intact mucosal barrier can prevent pathogens or antigenic elements of pathogens from entering the lamina propria of the gut. Disruption of the epithelial barrier caused by the TJ proteins deficiency will lead to microorganism translocation to the mucosal surface, disrupting microbial homeostasis. Furthermore, it has been confirmed that Bifidobacterium (Bf.) breve treatment promoted the proliferation of small intestine epithelial in mice (Ishizuka et al., 2009). The proliferation of small intestinal epithelial cells promotes the production of new cells and the shedding of necrotic cells. The physical barrier created by the epithelial cells constantly renews itself and enhances the ability of the mucosa physical barrier to resist pathogens. Moreover, probiotics modulated the trans-epithelial electrical resistance and epithelial permeability (Krishnan et al., 2016). The balance of the intestinal microbial environment is necessary for humans and animals to maintain homeostasis. In summary, probiotics can inhibit viral infections by maintaining intestinal permeability and mucosal barrier integrity.
Probiotics Modulate the Immune Barrier to Exert Antiviral Effects
One of the most beneficial effects of probiotics is to modulate the immune response (Shida and Nanno, 2008; Azad et al., 2018). The immune barrier is the last line of the host to inhibit viral infections, consisting of mucosal and systemic immune responses (Figures 1A, B) (Ashraf and Shah, 2014; La Fata et al., 2018).
Mucosal Immune Responses
Generally, there are large numbers of lymphoid tissues and immune cells in the gastrointestinal tract than in the rest of the body combined. The mucosal immune system (MIS), also known as mucosa-associated lymphoid tissues (MALTs), is mainly composed of immune tissues, cells, and molecules. The mucosa is directly connected to the external environment and involved in complex immune responses (Pelaseyed et al., 2014). Various immune cells are engaged in innate immune response. Natural killer (NK) cells are pivotal members of innate immunity that play key roles in recognizing and killing target cells and regulating immune responses. Activation and proliferation of NK cells can limit viral replication effectively. It has been reported that Lp. plantarum 06CC2 reduces HSV-1 virus yields in the brain of mice (Matsusaki et al., 2016). Lp. plantarum 06CC2 enhanced immunomodulatory activity by increasing the mRNA expression of IL-12 and IFN-γ in Peyer’s patches (PPs). IL-12 and IFN-γ can activate NK cells and macrophages effectively. Macrophages and NK cells cooperate with each other for virus clearance.
Pattern recognition receptors (PRRs) have extensive interactions between microorganisms and hosts. PRRs are specialized molecules that host cells recognize pathogens. At present, PRRs mainly include Toll-like receptors (TLRs), nucleotide-binding oligomerization domain (NOD)-like receptors (NLRs), retinoic acid-inducible-like receptors (RLRs), C-type lectin receptors (CLRs), absent in melanoma 2 (AIM2)-like receptors (ALRs) and cyclic GMP-AMP synthase (CGAs). Members of the TLRs can identify a wide range of pathogens, such as bacteria, viruses, and fungi. It has been demonstrated that Lacticaseibacillus rhamnosus GG (LGG) is therapeutically effective on diarrhea induced by rotavirus (RV) infection by the TLR3 signaling pathway (Aoki-Yoshida et al., 2016). Ligilactobacillus (Lg.) salivarius FFIG35 and FFIG58 also displayed antiviral effects by activating the TLR3 signaling pathway (Indo et al., 2021). TLR3 mainly recognizes nucleic acids in endosomes. Activation of TLR3 increases the expression of type I IFNs. In addition to activating TLR3, probiotics can also activate other PRRs. For example, L. acidophilus could enhance the antiviral effects by inducing the expression of virus immune defense genes in dendritic cells (DCs) in mice (Weiss et al., 2010). The expression of immune defense genes induced by viruses was dependent on the activation of the TLR2 pathway. In addition, Lp. plantarum could inhibit pneumovirus (PMV) infection in mice via the NLR (NOD2) and TLR (TLR2) pathways (Rice et al., 2016). Both NOD2 and TLR2 mediate the important innate immune response to inhibit viral infections. Several studies have shown that the probiotic mixtures also have immunomodulatory activity. The probiotics mixture (L. helveticus R0052, Bifidobacterium R0033, and Bifidobacterium R0071) had a major impact on downregulating the expression of proinflammatory cytokines, such as IL-6, IL-8, and IL-1β (Macpherson et al., 2014). The major effects include the upregulated expression of TLR3, mitogen-activated protein kinase, and factor-kappa B (NF-κB) expression. Furthermore, Lp. plantarum, W. cibaria, and Ll. sakei could modulate innate antiviral immune responses induced by poly(I:C) in IECs by activating the TLRs and NF-κB pathways (Kanmani and Kim, 2019). The NF-κB signaling pathway is associated with the production of proinflammatory cytokines. During viral infections, activating the NF-κB signaling pathway can increase the production of proinflammatory cytokines and regulate the function of immune cells.
IFNs are important mediators of antiviral immunity and regulation of immune system homeostasis (Stefan et al., 2020). Lc. rhamnosus CRL1505 has been reported to inhibit viral infections by inducing type I IFNs in intestinal antigen-presenting cells (APCs) (Villena et al., 2014). Lc. paracasei DG can also significantly induce the expression of type I IFNs, which can limit the viral replication and assembly (Ishizuka et al., 2016). Besides, Lp. plantarum Lp-1 has been shown to exert an anti-TGEV effect on IPEC-J2 cells by inducing large amounts of IFN-β in the early stage and activating the JAK-STAT1 pathway in the late stage, and the activated JAK-STAT1 pathway increases the transcription and expression of some antiviral proteins (Wang et al., 2019).
Efficient clearance of viruses depends on the orchestration of innate and adaptive immune responses. Accumulating evidence showed that probiotics can protect the host from viral infections by stimulating an adaptive immune response. Limosilactobacillus (Lm.) reuteri significantly reduced the viral loads of PCV2 in feces, ileum, and mesenteric lymph nodes (MLNs) and increased the immunoglobulin A (IgA) in the ileum (Karaffova et al., 2017). IgA, the main component of the mucosal immune system, is widely distributed in the mucosal secretions of host mucosal tissues, which can inhibit viral attachment to epithelial cells, slow down viral replication, and play important roles in the immune barrier. Increasing evidence has shown that not only live probiotics but also heat-killed probiotics have immunomodulatory effects. For example, heat-killed Lc. casei DK128 has broad protection against IFV infection by intranasal treatment by inducing virus-specific antibodies (Jung et al., 2017). Secretory IgA can bind to the virus specifically and the antibody-captured virus can be destroyed by phagocytes.
Systemic Immune Responses
Probiotics colonized on mucosal surfaces also have distal protective activity against viral infections (Figure 1B). It has been confirmed that Lm. reuteri has antiviral effects by regulating local mucosal immunity (Karaffova et al., 2017). A recent study has shown that Lm. reuteri can also protect the mice from viral infections by regulating the systemic immune responses (Mudronova et al., 2018). Furthermore, orally administrated Lc. rhamnosus LA68 induces the expression of some cytokines in the spleen or blood and activates Th1-type immune response (Dimitrijevic et al., 2014). Probiotics or their metabolites can activate immune cells to move distal locations to mediate antiviral responses, or by stimulating immune cells to release cytokines that act distally through blood and lymphatic circulation.
Bifidobacterium, one of the main members of intestinal microorganisms, has immunomodulatory activity. Bf. longum improved clinical symptoms and reduced mortality in mice after being inoculated intranasally with IFV. The Bf. longum MM-2 enhanced NK cell activity in the lungs and spleen and increased the expression of cytokines (IFN-γ, IL-2, IL-12, and IL-18) (Kawahara et al., 2015). E. faecalis CECT7121 induced strong activation of DCs and secretion of high levels of inflammatory cytokines (IL-12, IL-6, TNF-α, and IL-10) (Molina et al., 2015). These cytokines are essential for antiviral innate immune response. IL-10 is a well-established inflammatory and immunosuppressive factor, which can regulate immune responses. Due to viral infections, the increase of IL-10 expression can reduce the damage to the host caused by inflammation. DCs are the sentinel cells in the immune system, acting as APCs. They are also the bridge between innate immunity and adaptive immunity and can activate T and B cells directly or migrate to the mesenteric lymph nodes. Besides, orally administrated Lp. plantarum conferred protective activities to IFV by producing high levels of IL-12 and IFN-γ in the lungs (Takeda et al., 2011; Park et al., 2013). IL-12 is a key regulatory molecule of innate immunity and adaptive immunity.
IFNs are mediators of innate immunity and play key roles in resisting viral infections. It is reported that double-stranded RNAs produced by lactic acid bacteria (LABs) triggered DCs to produce IFN-β (Kawashima et al., 2018). Lc. lactis JCM5805 regulated immune response to IFV in humans by increasing the expression of IFN-α and ISGs (Sugimura et al., 2015). In addition, oral administration of heat-killed Lp. plantarum had a protective activity on H1N1 influenza virus infection in mice (Maeda et al., 2009). The beneficial effects of heat-killed Lp. plantarum was mediated by inducing host cells to produce type I IFNs. The probiotic mixture can also protect the host from RSV infection by stimulating AM to produce IFNs (Ji et al., 2021). Activation of the IFN signaling pathway increases the expression of ISGs, the major antiviral effectors of IFNs. A growing number of studies have shown that ISGs target different stages of viral replication to inhibit viral infections. It has been reported that B. velezensis can reduce the pigeon circovirus (PiCV) viral load significantly in the feces and spleen of pigeons by upregulating Mx1 and signal transducers and activators of transcription 1 (STAT1) genes (Tsai et al., 2021). Myxovirus resistance 1 (Mx1), one of ISGs, can block the early transcription of its nucleic acid after the virus invades the cell (Zurcher et al., 1992). Orally administrated Lc. rhamnosus can improve the resistance to RSV infection by producing type I IFNs and ISGs (including IFNAR1, Mx2, OAS1, OAS2, RNase L, and IFITM3) (Garcia-Castillo et al., 2020). These molecules enhanced the ability to inhibit RSV infection in mice. L. gasseri SBT2055 also has prophylactic potential to prevent RSV infection by upregulating the expression of IFNs and ISGs (Eguchi et al., 2019). Moreover, a candidate protein SRCAP of L. gasseri SBT2055 with RSV antiviral activity was identified. But the exact function of SRCAP protein to inhibit RSV replication needs to be further determined. Furthermore, L. gasseri SBT2055 can also protect mice from IFV infection by increasing the expression of the Mx1 and Oas1a genes, which are critical for reducing virus titer in the lungs (Nakayama et al., 2014). Upregulated ISGs can inhibit IFV infection effectively.
Moreover, heat-killed E. faecalis protected mice suppress influenza virus and enterovirus infections (Chen et al., 2017). The protective activity of E. faecalis is associated with the activation of the MCP-1/CCR2 pathway, which might act as a key mediator in the improved antiviral immune response. The expression level of MCP-1 was negatively correlated with virus load.
It is well known that the efficient elimination of viruses relies on adaptive immunity. The mice treated with Bf. bifidum produced antibodies, IL-4, IL-12, and IFN-γ, and protected from the challenge with H1N1 influenza virus (Mahooti et al., 2019). IL-4 can induce Th2 immune responses, while IFN-γ modulates Th1 immune responses. The balance between Th1 and Th2 is important for the homeostasis of the host immune system. Oral administration of Lp. plantarum (YU) induced sIgA and neutralizing antibodies in bronchoalveolar lavage fluids and suppressed viral proliferation in the lungs (Kawashima et al., 2011). Moreover, oral administration of Lc. rhamnosus M21 increased the survival of mice after IFV challenge and sIgA and Th1 cytokines (IL-2 and IFN-γ) were significantly increased (Song et al., 2016). Therefore, the resistance of mice to IFV infection is attributed to the cellular immune responses activated by Lc. rhamnosus M21.
Conclusions and Perspectives
The world is now facing a multitude of novel infectious diseases. Among them, viral diseases are particularly serious. Currently, WHO has stated that severe coronavirus disease 2019 (COVID-19) is a pandemic challenge to humanity. Vaccination is an important tool to inhibit viral infections. But there is a lag in vaccine development for novel viruses and some antiviral drugs also have some adverse reactions. The development of novel antiviral strategies is imminent. The role of probiotics in human and animal health has been an interesting topic in recent years. Probiotics have been proved to exert antiviral effects, representing safe alternative prophylactics for viral diseases in the future (Stavropoulou and Bezirtzoglou, 2020; Sundararaman et al., 2020). However, only a few probiotics have been investigated for their antiviral effects, and the clinical data are still insufficient. At present, the antiviral effects of probiotics were mainly carried out in vitro or in mouse models, which needs to be validated in the natural host of the virus. More animal models remain to be established. Accumulating evidence has indicated that the antiviral effects of probiotics are correlated with the routes of administration. The usual delivery route for probiotics is oral administration. But recent studies have shown that probiotics administered intranasally or sublingually can also reduce viral loads and improve the survival of animals. Intranasal and sublingual routes may be alternatives to oral administration. The dosage of probiotics will also affect the antiviral effects. The same probiotics strain in different doses can induce different immune responses. An excessive dose of probiotics may increase the risk of immunosuppression. Thus, we should pay more attention to the species, dosages, and routes of administration of probiotics when using probiotics-based antiviral agents. In addition, more clinical studies should be conducted to reveal which probiotics or their combinations would be the most effective ones for specific viruses.
Homeostasis of the immune system helps the host against viral infections. It has been proved that probiotics can activate not only local immune response but also systemic immune response to viral infections. The exact mechanisms remain unverified. Though the antiviral mechanism of probiotics has been partially uncovered, including probiotic components or metabolites and corresponding host PRRs contributing to the antiviral effects as shown in Table 1, it is necessary to understand the antiviral effects of probiotics in more details and more extensive and accurate investigations are demanded to clarify the mechanisms underlying the antiviral effects of probiotics using novel molecular tools and technologies. Understanding the antiviral immunity of humans and animals in the context of probiotics is conducive to the development of new antiviral strategies. The antiviral effects of various probiotics will become an interesting area of future research.
Author Contributions
YW wrote the manuscript. AM, JH, YS and H-JQ revised this manuscript. All authors contributed to the article and approved the submitted version.
Funding
This study was supported by the National Key R&D Program of China (Grant no. 2021YFD1801403).
Conflict of Interest
The authors declare that the research was conducted in the absence of any commercial or financial relationships that could be construed as a potential conflict of interest.
Publisher’s Note
All claims expressed in this article are solely those of the authors and do not necessarily represent those of their affiliated organizations, or those of the publisher, the editors and the reviewers. Any product that may be evaluated in this article, or claim that may be made by its manufacturer, is not guaranteed or endorsed by the publisher.
References
Al-Ghazzewi, F. H., Tester, R. F. (2016). Biotherapeutic Agents and Vaginal Health. J. Appl. Microbiol. 121, 18–27. doi: 10.1111/jam.13054
Antunes, K. H., Fachi, J. L., de Paula, R., Da, S. E., Pral, L. P., Dos, S. A., et al. (2019). Microbiota-Derived Acetate Protects Against Respiratory Syncytial Virus Infection Through a GPR43-Type 1 Interferon Response. Nat. Commun. 10, 3273. doi: 10.1038/s41467-019-11152-6
Aoki-Yoshida, A., Saito, S., Fukiya, S., Aoki, R., Takayama, Y., Suzuki, C., et al. (2016). Lactobacillus rhamnosus GG Increases Toll-Like Receptor 3 Gene Expression in Murine Small Intestine Ex Vivo and In Vivo. Benef. Microbes 7, 421–429. doi: 10.3920/BM2015.0169
Ashraf, R., Shah, N. P. (2014). Immune System Stimulation by Probiotic Microorganisms. Crit. Rev. Food Sci. 54, 938–956. doi: 10.1080/10408398.2011.619671
Azad, M., Sarker, M., Wan, D. (2018). Immunomodulatory Effects of Probiotics on Cytokine Profiles. Biomed. Res. Int. 2018, 8063647. doi: 10.1155/2018/8063647
Boparai, J. K., Sharma, P. K. (2020). Mini Review on Antimicrobial Peptides, Sources, Mechanism and Recent Applications. Protein Pept. Lett. 27, 4–16. doi: 10.2174/0929866526666190822165812
Bosch, T., Zasloff, M. (2021). Antimicrobial Peptides or How Our Ancestors Learned to Control the Microbiome. mBio 12, e0184721. doi: 10.1128/mBio.01847-21
Botic, T., Klingberg, T. D., Weingartl, H., Cencic, A. (2007). A Novel Eukaryotic Cell Culture Model to Study Antiviral Activity of Potential Probiotic Bacteria. Int. J. Food Microbiol. 115, 227–234. doi: 10.1016/j.ijfoodmicro.2006.10.044
Caballero-Franco, C., Keller, K., De Simone, C., Chadee, K. (2007). The VSL3 Probiotic Formula Induces Mucin Gene Expression and Secretion in Colonic Epithelial Cells. Am. J. Physiol. Gastrointest. Liver Physiol. 292, G315–G322. doi: 10.1152/ajpgi.00265.2006
Chen, M. F., Weng, K. F., Huang, S. Y., Liu, Y. C., Tseng, S. N., Ojcius, D. M., et al. (2017). Pretreatment with a Heat-Killed Probiotic Modulates Monocyte Chemoattractant Protein-1 and Reduces the Pathogenicity of Influenza and Enterovirus 71 Infections. Mucosal Immunol. 10, 215–227. doi: 10.1038/mi.2016.31
Conti, C., Malacrino, C., Mastromarino, P. (2009). Inhibition of Herpes Simplex Virus Type 2 by Vaginal Lactobacilli. J. Physiol. Pharmacol. 6, 19–26. doi: 10.1016/j.jcma.2017.07.010
Dimitrijevic, R., Ivanovic, N., Mathiesen, G., Petrusic, V., Zivkovic, I., Djordjevic, B., et al. (2014). Effects of Lactobacillus rhamnosus LA68 on the Immune System of C57BL/6 Mice upon Oral Administration. J. Dairy Res. 81, 202–207. doi: 10.1017/S0022029914000028
Di Pierro, F., Pane, M. (2021). Bifidobacterium Longum W11: Uniqueness and Individual or Combined Clinical Use in Association with Rifaximin. Clin. Nutr. Espen. 42, 15–21. doi: 10.1016/j.clnesp.2020.12.025
Drago, L., Rodighiero, V., Celeste, T., Rovetto, L., De Vecchi, E. (2010). Microbiological Evaluation of Commercial Probiotic Products Available in the USA in 2009. J. Chemother. 22, 373–377. doi: 10.1179/joc.2010.22.6.373
Egert, M., Simmering, R. (2016). The Microbiota of the Human Skin. Adv. Exp. Med. Biol. 902, 61–81. doi: 10.1007/978-3-319-31248-4_5
Eguchi, K., Fujitani, N., Nakagawa, H., Miyazaki, T. (2019). Prevention of Respiratory Syncytial Virus Infection With Probiotic Lactic Acid Bacterium Lactobacillus gasseri SBT2055. Sci. Rep. 9, 4812. doi: 10.1038/s41598-019-39602-7
Engevik, M. A., Luk, B., Chang-Graham, A. L., Hall, A., Herrmann, B., Ruan, W., et al. (2019). Bifidobacterium dentium Fortifies the Intestinal Mucus Layer via Autophagy and Calcium Signaling Pathways. mBio 10. doi: 10.1128/mBio.01087-19
Ermolenko, E. I., Desheva, Y. A., Kolobov, A. A., Kotyleva, M. P., Sychev, I. A., Suvorov, A. N. (2019). Anti-Influenza Activity of Enterocin B in vitro and Protective Effect of Bacteriocinogenic Enterococcal Probiotic Strain on Influenza Infection in Mouse Model. Probiotics Antimicrob. Proteins 11, 705–712. doi: 10.1007/s12602-018-9457-0
Food and Agriculture Organization of the United Nations/World Health Organization (FAO/WHO) (2002). Guidelines for the Evaluation of Probiotics in Food (London, Ontario, Canada: Joint FAO/WHO Working Group on Drafting Guidelines for the Evaluation of Probiotics in Food).
Garcia-Castillo, V., Tomokiyo, M., Raya, T. F., Islam, M. A., Takahashi, H., Kitazawa, H., et al. (2020). Alveolar Macrophages are Key Players in the Modulation of the Respiratory Antiviral Immunity Induced by Orally Administered Lacticaseibacillus Rhamnosus CRL1505. Front. Immunol. 11. doi: 10.3389/fimmu.2020.568636
Gasbarrini, G., Bonvicini, F., Gramenzi, A. (2016). Probiotics History. J. Clin. Gastroenterol. 2, 13–15. doi: 10.1097/MCG.0000000000000697
Giannakou, K., Visinoni, F., Zhang, P., Nathoo, N., Jones, P., Cotterrell, M., et al. (2021). Biotechnological Exploitation of Saccharomyces Jurei and its Hybrids in Craft Beer Fermentation Uncovers New Aroma Combinations. Food Microbiol. 100, 103838. doi: 10.1016/j.fm.2021.103838
Gosmann, C., Anahtar, M. N., Handley, S. A., Farcasanu, M., Abu-Ali, G., Bowman, B. A., et al. (2017). Lactobacillus-Deficient Cervicovaginal Bacterial Communities are Associated with Increased HIV Acquisition in Young South African Women. Immunity 46, 29–37. doi: 10.1016/j.immuni.2016.12.013
Gunzel, D., Fromm, M. (2012). Claudins and Other Tight Junction Proteins. Compr. Physiol. 2, 1819–1852. doi: 10.1002/cphy.c110045
Hao, Q., Dong, B. R., Wu, T. (2015). Probiotics for Preventing Acute Upper Respiratory Tract Infections. Cochrane Database Syst. Rev. 2, 6895. doi: 10.1002/14651858
Heintz-Buschart, A., Wilmes, P. (2018). Human Gut Microbiome: Function Matters. Trends Microbiol. 26, 563–574. doi: 10.1016/j.tim.2017.11.002
Indo, Y., Kitahara, S., Tomokiyo, M., Araki, S., Islam, M. A., Zhou, B., et al. (2021). Ligilactobacillus salivarius Strains Isolated from the Porcine Gut Modulate Innate Immune Responses in Epithelial Cells and Improve Protection against Intestinal Viral-Bacterial Superinfection. Front. Immunol. 12. doi: 10.3389/fimmu.2021.652923
Ishizuka, S., Iwama, A., Dinoto, A., Suksomcheep, A., Maeta, K., Kasai, T., et al. (2009). Synbiotic Promotion of Epithelial Proliferation by Orally Ingested Encapsulated Bifidobacterium breve and Raffinose in the Small Intestine of Rats. Mol. Nutr. Food Res. 53, S62–S67. doi: 10.1002/mnfr.200800041
Ishizuka, T., Kanmani, P., Kobayashi, H., Miyazaki, A., Soma, J., Suda, Y., et al. (2016). Immunobiotic Bifidobacteria Strains Modulate Rotavirus Immune Response in Porcine Intestinal Epitheliocytes via Pattern Recognition Receptor Signaling. PLoS One 11, e0152416. doi: 10.1371/journal.pone.0152416
Ji, J. J., Sun, Q. M., Nie, D. Y., Wang, Q., Zhang, H., Qin, F. F., et al. (2021). Probiotics Protect Against RSV Infection by Modulating the Microbiota-Alveolar-Macrophage Axis. Acta Pharmacol. Sin. 42, 1630–1641. doi: 10.1038/s41401-020-00573-5
Jung, Y. J., Lee, Y. T., Ngo, V. L., Cho, Y. H., Ko, E. J., Hong, S. M., et al. (2017). Heat-Killed Lactobacillus casei Confers Broad Protection against Influenza A Virus Primary Infection and Develops Heterosubtypic Immunity Against Future Secondary Infection. Sci. Rep. 7, 17360. doi: 10.1038/s41598-017-17487-8
Kahouli, I., Tomaro-Duchesneau, C., Prakash, S. (2013). Probiotics in Colorectal Cancer (CRC) with Emphasis on Mechanisms of Action and Current Perspectives. J. Med. Microbiol. 62, 1107–1123. doi: 10.1099/jmm.0.048975-0
Kanmani, P., Kim, H. (2019). Immunobiotic Strains Modulate Toll-Like Receptor 3 Agonist Induced Innate Antiviral Immune Response in Human Intestinal Epithelial Cells by Modulating IFN Regulatory Factor 3 and NF-κB Signaling. Front. Immunol. 101536. doi: 10.3389/fimmu.2019.01536
Karaffova, V., Csank, T., Mudronova, D., Kiraly, J., Revajova, V., Gancarcikova, S., et al. (2017). Influence of Lactobacillus reuteri L26 BiocenolTM on Immune Response Against Porcine Circovirus Type 2 Infection in Germ-Free Mice. Benef. Microbes 8, 367–378. doi: 10.3920/BM2016.0114
Karst, S. M. (2016). The Influence of Commensal Bacteria on Infection with Enteric Viruses. Nat. Rev. Microbiol. 14, 197–204. doi: 10.1038/nrmicro.2015.25
Kawahara, T., Takahashi, T., Oishi, K., Tanaka, H., Masuda, M., Takahashi, S., et al. (2015). Consecutive Oral Administration of Bifidobacterium longum MM-2 Improves the Defense System against Influenza Virus Infection by Enhancing Natural Killer Cell Activity in a Murine Model. Microbiol. Immunol. 59, 1–12. doi: 10.1111/1348-0421.12210
Kawashima, T., Hayashi, K., Kosaka, A., Kawashima, M., Igarashi, T., Tsutsui, H., et al. (2011). Lactobacillus plantarum Strain YU from Fermented Foods Activates Th1 and Protective Immune Responses. Int. Immunopharmacol. 11, 2017–2024. doi: 10.1016/j.intimp.2011.08.013
Kawashima, T., Ikari, N., Watanabe, Y., Kubota, Y., Yoshio, S., Kanto, T., et al. (2018). Double-Stranded RNA Derived from Lactic Acid Bacteria Augments Th1 Immunity via Interferon-Beta from Human Dendritic Cells. Front. Immunol. 9. doi: 10.3389/fimmu.2018.00027
Kim, S. K., Guevarra, R. B., Kim, Y. T., Kwon, J., Kim, H., Cho, J. H., et al. (2019). Role of Probiotics in Human Gut Microbiome-Associated Diseases. J. Microbiol. Biotechnol. 29, 1335–1340. doi: 10.4014/jmb.1906.06064
Klebanoff, S. J., Coombs, R. W. (1991). Viricidal Effect of Lactobacillus Acidophilus on Human Immunodeficiency Virus Type 1: Possible Role in Heterosexual Transmission. J. Exp. Med. 174, 289–292. doi: 10.1084/jem.174.1.289
Knezevic, A., Stepanovic, S., Cupic, M., Jevtovic, D., Ranin, J., Jovanovic, T. (2005). Reduced Quantity and Hydrogen-Peroxide Production of Vaginal Lactobacilli in HIV Positive Women. Biomed. Pharmacother. 59, 521–533. doi: 10.1016/j.biopha.2005.06.010
Krishnan, M., Penrose, H. M., Shah, N. N., Marchelletta, R. R., McCole, D. F. (2016). VSL3 Probiotic Stimulates T-Cell Protein Tyrosine Phosphatase-Mediated Recovery of IFN-Gamma-Induced Intestinal Epithelial Barrier Defects. Inflamm. Bowel Dis. 22, 2811–2823. doi: 10.1097/MIB.0000000000000954
La Fata, G., Weber, P., Mohajeri, M. H. (2018). Probiotics and the Gut Immune System: Indirect Regulation. Probiotics Antimicrob. Proteins 10, 11–21. doi: 10.1007/s12602-017-9322-6
LeBlanc, J. G., Chain, F., Martin, R., Bermudez-Humaran, L. G., Courau, S., Langella, P. (2017). Beneficial Effects on Host Energy Metabolism of Short-Chain Fatty Acids and Vitamins Produced by Commensal and Probiotic Bacteria. Microb. Cell Fact. 16, 79. doi: 10.1186/s12934-017-0691-z
Lievin-Le, M. V., Servin, A. L. (2014). Anti-Infective Activities of Lactobacillus Strains in the Human Intestinal Microbiota: From Probiotics to Gastrointestinal Anti-Infectious Biotherapeutic Agents. Clin. Microbiol. Rev. 27, 167–199. doi: 10.1128/CMR.00080-13
Liu, Z., Xu, C., Tian, R., Wang, W., Ma, J., Gu, L., et al. (2021). Screening Beneficial Bacteriostatic Lactic Acid Bacteria in the Intestine and Studies of Bacteriostatic Substances. J. Zhejiang Univ. Sci. B 22, 533–547. doi: 10.1631/jzus.B2000602
Liu, Q., Yu, Z., Tian, F., Zhao, J., Zhang, H., Zhai, Q., et al. (2020). Surface Components and Metabolites of Probiotics for Regulation of Intestinal Epithelial Barrier. Microb. Cell Fact. 19, 23. doi: 10.1186/s12934-020-1289-4
Lloyd-Price, J., Abu-Ali, G., Huttenhower, C. (2016). The Healthy Human Microbiome. Genome Med. 8, 51. doi: 10.1186/s13073-016-0307-y
Lorella, P., Lorella, T., Cristina, B., Laura, P., Carla, D., Lucio, P., et al. (2020). Protective Action of Bacillus Clausii Probiotic Strains in an In Vitro Model of Rotavirus Infection. Sci. Rep. 10, 12636. doi: 10.1038/s41598-020-69533-7
Lu, W., Fang, Z., Liu, X., Li, L., Zhang, P., Zhao, J., et al. (2021). The Potential Role of Probiotics in Protection against Influenza a Virus Infection in Mice. Foods 10, 902. doi: 10.3390/foods10040902
Lunjani, N., Hlela, C., O'Mahony, L. (2019). Microbiome and Skin Biology. Curr. Opin. Allergy Clin. Immunol. 19, 328–333. doi: 10.1097/ACI.0000000000000542
Macpherson, C., Audy, J., Mathieu, O., Tompkins, T. A. (2014). Multistrain Probiotic Modulation of Intestinal Epithelial Cells' Immune Response to a Double-Stranded RNA Ligand, Poly(I.C). Appl. Environ. Microbiol. 80, 1692–1700. doi: 10.1128/AEM.03411-13
Maeda, N., Nakamura, R., Hirose, Y., Murosaki, S., Yamamoto, Y., Kase, T., et al. (2009). Oral Administration of Heat-Killed Lactobacillus plantarum L-137 Enhances Protection against Influenza Virus Infection by Stimulation of Type I Interferon Production in Mice. Int. Immunopharmacol. 9, 1122–1125. doi: 10.1016/j.intimp.2009.04.015
Mahooti, M., Abdolalipour, E., Salehzadeh, A., Mohebbi, S. R., Gorji, A., Ghaemi, A. (2019). Immunomodulatory and Prophylactic Effects of Bifidobacterium bifidum Probiotic Strain on Influenza Infection in Mice. World J. Microbiol. Biotechnol. 35, 91. doi: 10.1007/s11274-019-2667-0
Matsusaki, T., Takeda, S., Takeshita, M., Arima, Y., Tsend-Ayush, C., Oyunsuren, T., et al. (2016). Augmentation of T Helper Type 1 Immune Response through Intestinal Immunity in Murine Cutaneous Herpes Simplex Virus Type 1 Infection by Probiotic Lactobacillus plantarum Strain 06CC2. Int. Immunopharmacol. 39, 320–327. doi: 10.1016/j.intimp.2016.08.001
Mishra, A., Lai, G. C., Yao, L. J., Aung, T. T., Shental, N., Rotter-Maskowitz, A., et al. (2021). Microbial Exposure during Early Human Development Primes Fetal Immune Cells. Cell 184, 3394–3409. doi: 10.1016/j.cell.2021.04.039
Molina, M. A., Diaz, A. M., Hesse, C., Ginter, W., Gentilini, M. V., Nunez, G. G., et al. (2015). Immunostimulatory Effects Triggered by Enterococcus faecalis CECT7121 Probiotic Strain Involve Activation of Dendritic Cells and Interferon-Gamma Production. PLoS One 10, e0127262. doi: 10.1371/journal.pone.0127262
Mudronova, D., Karaffova, V., Csank, T., Kiraly, J., Revajova, V., Gancarcikova, S., et al. (2018). Systemic Immune Response of Gnotobiotic Mice Infected with Porcine Circovirus Type 2 After Administration of Lactobacillus reuteri L26 Biocenol. Benef. Microbes 9, 951–961. doi: 10.3920/BM2017.0147
Nahui, P. R., Vanpouille, C., Laghi, L., Parolin, C., Melikov, K., Backlund, P., et al. (2019). Extracellular Vesicles from Symbiotic Vaginal Lactobacilli Inhibit HIV-1 Infection of Human Tissues. Nat. Commun. 10, 5656. doi: 10.1038/s41467-019-13468-9
Nahui, P. R., Zicari, S., Vanpouille, C., Vitali, B., Margolis, L. (2017). Vaginal Lactobacillus Inhibits HIV-1 Replication in Human Tissues Ex Vivo. Front. Microbiol. 8. doi: 10.3389/fmicb.2017.00906
Nakayama, Y., Moriya, T., Sakai, F., Ikeda, N., Shiozaki, T., Hosoya, T., et al. (2014). Oral Administration of Lactobacillus gasseri SBT2055 is Effective for Preventing Influenza in Mice. Sci. Rep. 4, 4638. doi: 10.1038/srep04638
O'Hanlon, D. E., Moench, T. R., Cone, R. A. (2011). In Vaginal Fluid, Bacteria Associated with Bacterial Vaginosis can be Suppressed with Lactic Acid But Not Hydrogen Peroxide. BMC Infect. Dis. 11, 200. doi: 10.1186/1471-2334-11-200
Otani, T., Furuse, M. (2020). Tight Junction Structure and Function Revisited. Trends Cell Biol. 30, 805–817. doi: 10.1016/j.tcb.2020.08.004
Park, M. K., Ngo, V., Kwon, Y. M., Lee, Y. T., Yoo, S., Cho, Y. H., et al. (2013). Lactobacillus plantarum DK119 as a Probiotic Confers Protection against Influenza Virus by Modulating Innate Immunity. PLoS One 8, e75368. doi: 10.1371/journal.pone.0075368
Peixoto, R. S., Harkins, D. M., Nelson, K. E. (2021). Advances in Microbiome Research for Animal Health. Annu. Rev. Anim. Biosci. 9, 289–311. doi: 10.1146/annurev-animal-091020-075907
Pelaseyed, T., Bergstrom, J. H., Gustafsson, J. K., Ermund, A., Birchenough, G. M., Schutte, A., et al. (2014). The Mucus and Mucins of the Goblet Cells and Enterocytes Provide the First Defense Line of the Gastrointestinal Tract and Interact with the Immune System. Immunol. Rev. 260, 8–20. doi: 10.1111/imr.12182
Piewngam, P., Zheng, Y., Nguyen, T. H., Dickey, S. W., Joo, H. S., Villaruz, A. E., et al. (2018). Pathogen Elimination by Probiotic Bacillus via Signalling Interference. Nature 562, 532–537. doi: 10.1038/s41586-018-0616-y
Pradhan, D., Biswasroy, P., Kar, B., Bhuyan, S. K., Ghosh, G., Rath, G. (2021). Clinical Interventions and Budding Applications of Probiotics in the Treatment and Prevention of Viral Infections. Arch. Med. Res. 8, 122–130. doi: 10.1016/j.arcmed.2021.09.008
Rad, A. H., Aghebati-Maleki, L., Kafil, H. S., Abbasi, A. (2021). Molecular Mechanisms of Postbiotics in Colorectal Cancer Prevention and Treatment. Crit. Rev. Food Sci. Nutr. 61, 1787–1803. doi: 10.1080/10408398.2020.1765310
Rice, T. A., Brenner, T. A., Percopo, C. M., Ma, M., Keicher, J. D., Domachowske, J. B., et al. (2016). Signaling via Pattern Recognition Receptors NOD2 and TLR2 Contributes to Immunomodulatory Control of Lethal Pneumovirus Infection. Antiviral Res. 132, 131–140. doi: 10.1016/j.antiviral.2016.06.002
Salaris, C., Scarpa, M., Elli, M., Bertolini, A., Guglielmetti, S., Pregliasco, F., et al. (2021). Lacticaseibacillus paracasei DG Enhances the Lactoferrin Anti-SARS-CoV-2 Response in Caco-2 Cells. Gut Microbes 13, 1961970. doi: 10.1080/19490976.2021.1961970
Schmidt, T., Raes, J., Bork, P. (2018). The Human Gut Microbiome: From Association to Modulation. Cell 172, 1198–1215. doi: 10.1016/j.cell.2018.02.044
Sender, R., Fuchs, S., Milo, R. (2016). Are We Really Vastly Outnumbered? Revisiting the Ratio of Bacterial to Host Cells in Humans. Cell 164, 337–340. doi: 10.1016/j.cell.2016.01.013
Shen, J., Zuo, Z. X., Mao, A. P. (2014). Effect of Probiotics on Inducing Remission and Maintaining Therapy in Ulcerative Colitis, Crohn's Disease, and Pouchitis: Meta-Analysis of Randomized Controlled Trials. Inflamm. Bowel Dis. 20, 21–35. doi: 10.1097/01.MIB.0000437495.30052.be
Shida, K., Nanno, M. (2008). Probiotics and Immunology: Separating the Wheat from the Chaff. Trends Immunol. 29, 565–573. doi: 10.1016/j.it.2008.07.011
Song, J. A., Kim, H. J., Hong, S. K., Lee, D. H., Lee, S. W., Song, C. S., et al. (2016). Oral Intake of Lactobacillus rhamnosus M21 Enhances the Survival Rate of Mice Lethally Infected with Influenza Virus. J. Microbiol. Immunol. Infect. 49, 16–23. doi: 10.1016/j.jmii.2014.07.011
Stavropoulou, E., Bezirtzoglou, E. (2020). Probiotics in Medicine: A Long Debate. Front. Immunol. 11. doi: 10.3389/fimmu.2020.02192
Stefan, K. L., Kim, M. V., Iwasaki, A., Kasper, D. L. (2020). Commensal Microbiota Modulation of Natural Resistance to Virus Infection. Cell 183, 1312–1324.e10. doi: 10.1016/j.cell.2020.10.047
Sugimura, T., Takahashi, H., Jounai, K., Ohshio, K., Kanayama, M., Tazumi, K., et al. (2015). Effects of Oral Intake of Plasmacytoid Dendritic Cells-Stimulative Lactic Acid Bacterial Strain on Pathogenesis of Influenza-Like Illness and Immunological Response to Influenza Virus. Br. J. Nutr. 114, 727–733. doi: 10.1017/S0007114515002408
Sundararaman, A., Ray, M., Ravindra, P. V., Halami, P. M. (2020). Role of Probiotics to Combat Viral Infections with Emphasis on COVID-19. Appl. Microbiol. Biotechnol. 104, 8089–8104. doi: 10.1007/s00253-020-10832-4
Tachedjian, G., Aldunate, M., Bradshaw, C. S., Cone, R. A. (2017). The Role of Lactic Acid Production by Probiotic Lactobacillus Species in Vaginal Health. Res. Microbiol. 168, 782–792. doi: 10.1016/j.resmic.2017.04.001
Takeda, S., Takeshita, M., Kikuchi, Y., Dashnyam, B., Kawahara, S., Yoshida, H., et al. (2011). Efficacy of Oral Administration of Heat-Killed Probiotics from Mongolian Dairy Products Against Influenza Infection in Mice: Alleviation of Influenza Infection by Its Immunomodulatory Activity through Intestinal Immunity. Int. Immunopharmacol. 11, 1976–1983. doi: 10.1016/j.intimp.2011.08.007
Teame, T., Wang, A., Xie, M., Zhang, Z., Yang, Y., Ding, Q., et al. (2020). Paraprobiotics and Postbiotics of Probiotic Lactobacilli, Their Positive Effects on the Host and Action Mechanisms: A Review. Front. Nutr. 7. doi: 10.3389/fnut.2020.570344
Tsai, C. Y., Hu, S. Y., Santos, H. M., Catulin, G., Tayo, L. L., Chuang, K. P. (2021). Probiotic Supplementation Containing Bacillus velezensis Enhances Expression of Immune Regulatory Genes against Pigeon Circovirus in Pigeons (Columba Livia). J. Appl. Microbiol. 130, 1695–1704. doi: 10.1111/jam.14893
Tyssen, D., Wang, Y. Y., Hayward, J. A., Agius, P. A., DeLong, K., Aldunate, M., et al. (2018). Anti-HIV-1 Activity of Lactic Acid in Human Cervicovaginal Fluid. mSphere 3, e00055–e00018. doi: 10.1128/mSphere.00055-18
VanderWaal, K., Deen, J. (2018). Global Trends in Infectious Diseases of Swine. Proc. Natl. Acad. Sci. U. S. A. 115, 11495–11500. doi: 10.1073/pnas.1806068115
Vieira, A. T., Galvão, I., Macia, L. M., Sernaglia, E. M., Vinolo, M. A. R., Garcia, C. C., et al. (2017). Dietary Fiber and the Short-Chain Fatty Acid Acetate Promote Resolution of Neutrophilic Inflammation in a Model of Gout in Mice. J. Leukocyte Biol. 101, 275–284. doi: 10.1189/jlb.3A1015-453RRR
Villena, J., Chiba, E., Vizoso-Pinto, M. G., Tomosada, Y., Takahashi, T., Ishizuka, T., et al. (2014). Immunobiotic Lactobacillus rhamnosus Strains Differentially Modulate Antiviral Immune Response in Porcine Intestinal Epithelial and Antigen Presenting Cells. BMC Microbiol. 14, 126. doi: 10.1186/1471-2180-14-126
Wachsman, M. B., Castilla, V., de Ruiz, H. A., de Torres, R. A., Sesma, F., Coto, C. E. (2003). Enterocin CRL35 Inhibits Late Stages of HSV-1 and HSV-2 Replication In Vitro. Antiviral Res. 58, 17–24. doi: 10.1016/s0166-3542(02)00099-2
Wang, Z., Chai, W., Burwinkel, M., Twardziok, S., Wrede, P., Palissa, C., et al. (2013). Inhibitory Influence of Enterococcus faecium on the Propagation of Swine Influenza A Virus In Vitro. PLoS One 8, 053043. doi: 10.1371/journal.pone.0053043
Wang, J., Ji, H., Wang, S., Liu, H., Zhang, W., Zhang, D., et al. (2018). Probiotic Lactobacillus plantarum Promotes Intestinal Barrier Function by Strengthening the Epithelium and Modulating Gut Microbiota. Front. Microbiol. 9. doi: 10.3389/fmicb.2018.01953
Wang, K., Ran, L., Yan, T., Niu, Z., Kan, Z., Zhang, Y., et al. (2019). Anti-TGEV Miller Strain Infection Effect of Lactobacillus plantarum Supernatant Based on the JAK-STAT1 Signaling Pathway. Front. Microbiol. 10. doi: 10.3389/fmicb.2019.02540
Weiss, G., Rasmussen, S., Zeuthen, L. H., Nielsen, B. N., Jarmer, H., Jespersen, L., et al. (2010). Lactobacillus acidophilus Induces Virus Immune Defence Genes in Murine Dendritic Cells by a Toll-Like Receptor-2-Dependent Mechanism. Immunology 131, 268–281. doi: 10.1111/j.1365-2567.2010.03301.x
Wieërs, G., Verbelen, V., Van Den Driessche, M., Melnik, E., Vanheule, G., Marot, J. C., et al. (2020). Do Probiotics during in-Hospital Antibiotic Treatment Prevent Colonization of Gut Microbiota with Multi-Drug-Resistant Bacteria? A Randomized Placebo-Controlled Trial Comparing Saccharomyces to a Mixture of Lactobacillus, Bifidobacterium, and Saccharomyces. Front. Public Health 8. doi: 10.3389/fpubh.2020.578089
Wong, J. M., de Souza, R., Kendall, C. W., Emam, A., Jenkins, D. J. (2006). Colonic Health: Fermentation and Short Chain Fatty Acids. J. Clin. Gastroenterol. 40, 235–243. doi: 10.1097/00004836-200603000-00015
Xu, H., Li, H. (2019). Acne, the Skin Microbiome, and Antibiotic Treatment. Am. J. Clin. Dermatol. 20, 335–344. doi: 10.1007/s40257-018-00417-3
Keywords: probiotics, viral infections, antiviral effects, antiviral mechanisms, novel antivirals
Citation: Wang Y, Moon A, Huang J, Sun Y and Qiu H-J (2022) Antiviral Effects and Underlying Mechanisms of Probiotics as Promising Antivirals. Front. Cell. Infect. Microbiol. 12:928050. doi: 10.3389/fcimb.2022.928050
Received: 25 April 2022; Accepted: 10 May 2022;
Published: 06 June 2022.
Edited by:
Zhanbo Zhu, Heilongjiang Bayi Agricultural University, ChinaReviewed by:
Kangcheng Pan, Sichuan Agricultural University, ChinaYonggang Qu, Shihezi University, China
Copyright © 2022 Wang, Moon, Huang, Sun and Qiu. This is an open-access article distributed under the terms of the Creative Commons Attribution License (CC BY). The use, distribution or reproduction in other forums is permitted, provided the original author(s) and the copyright owner(s) are credited and that the original publication in this journal is cited, in accordance with accepted academic practice. No use, distribution or reproduction is permitted which does not comply with these terms.
*Correspondence: Hua-Ji Qiu, cWl1aHVhamlAY2Fhcy5jbg==; Yuan Sun, c3VueXVhbkBjYWFzLmNu