- 1Department of Neurosurgery, Tampere University Hospital, Tampere, Finland
- 2Department of Neurosurgery, University of Helsinki, Helsinki University Hospital, Helsinki, Finland
- 3Division of Clinical Neurosciences, Department of Neurosurgery, and Turku Brain Injury Centre, Turku University Hospital, and University of Turku, Turku, Finland
- 4Priority Research Centre for Stroke and Brain Injury, School of Medicine and Public Health, University of Newcastle, Callaghan, NSW, Australia
- 5Sports Concussion Program, Hunter New England Local Health District, John Hunter Hospital, New Lambton Heights, NSW, Australia
- 6British Columbia Neuropsychiatry Program, Department of Psychiatry, University of British Columbia, Vancouver, BC, Canada
- 7Department of Physical Medicine and Rehabilitation, Harvard Medical School, Spaulding Rehabilitation Hospital, Home Base, A Red Sox Foundation and Massachusetts General Hospital Program, MassGeneral Hospital for Children™ Sports Concussion Program, Boston, MA, United States
Background: The extensive use of computed tomography (CT) after acute head injury is costly and carries potential iatrogenic risk. This systematic review examined the usefulness of blood-based glial fibrillary acidic protein (GFAP) for predicting acute trauma-related CT-positive intracranial lesions following head trauma. The main objective was to summarize the current evidence on blood-based GFAP as a potential screening test for acute CT-positive intracranial lesions following head trauma.
Methods: We screened MEDLINE, EMBASE, PsychInfo, CINAHL, Web of Science, the Cochrane Database, Scopus, Clinical Trials, OpenGrey, ResearchGate, and the reference lists of eligible publications for original contributions published between January 1980 and January 2017. Eligibility criteria included: (i) population: human head and brain injuries of all severities and ages; (ii) intervention: blood-based GFAP measurement ≤24 h post-injury; and (iii) outcome: acute traumatic lesion on non-contrast head CT ≤24 h post-injury. Three authors completed the publication screening, data extraction, and quality assessment of eligible articles.
Results: The initial search identified 4,706 articles, with 51 eligible for subsequent full-text assessment. Twenty-seven articles were ultimately included. Twenty-four (89%) studies reported a positive association between GFAP level and acute trauma-related intracranial lesions on head CT. The area under the receiver operating characteristic curve for GFAP prediction of intracranial pathology ranged from 0.74 to 0.98 indicating good to excellent discrimination. GFAP seemed to discriminate mass lesions and diffuse injury, with mass lesions having significantly higher GFAP levels. There was considerable variability between the measured GFAP averages between studies and assays. No well-designed diagnostic studies with specific GFAP cutoff values predictive of acute traumatic intracranial lesions have been published.
Conclusion: Intracranial CT-positive trauma lesions were associated with elevated GFAP levels in the majority of studies. Methodological heterogeneity in GFAP assessments and the lack of well-designed diagnostic studies with commercially validated GFAP platforms hinder the level of evidence, and variability in levels of GFAP with no clearly established cutoff for abnormality limit the clinical usefulness of the biomarker. However, blood-based GFAP holds promise as a means of screening for acute traumatic CT-positive lesion following head trauma.
Introduction
Rationale
Since the inception of computed tomography (CT) in the 1970s, its use has increased rapidly (1). Between 1980 and 2017, the number of annual CT scans in the United States has increased from 3 to 62 million (2) with the head being the most commonly imaged area. In modern medicine, non-contrast head CT is the gold standard for identifying significant intracranial injury in an emergency department setting (3). Numerous decision rules [e.g., New Orleans Criteria (4), Canadian CT Head Rule (5)] have been developed in order to focus CT imaging on patients with the greatest risk for clinically significant intracranial injury. However, despite these decision algorithms, a considerable number of trauma head CT scans are performed unnecessarily (1, 6). Almost 80% find no evidence of acute intracranial pathology (7).
There is a non-trivial iatrogenic risk associated with CT scanning, mainly for radiation-induced neoplasia. One head CT significantly increases the risk of subsequent cancer with subsequent CTs conferring additive vulnerability (6, 8). Further, considering the economic cost associated with CT proliferation, judicious use of this imaging modality is important. A growing body of evidence has shown that some blood-based brain trauma biomarkers could aid in predicting which patients will have acute intracranial abnormalities, thus possibly reducing unnecessary head CT scanning (9, 10). Glial fibrillary acidic protein (GFAP) is one of these biomarkers (9, 10).
For several decades, S100B has been investigated as a blood-based marker of brain damage (11). Since the publication of the most recent Scandinavian guidelines for head injury management (12), S100B has been adopted into clinical use mainly in some Nordic countries. According to the guidelines, S100B can be used to substitute head CT scanning in isolated mild head injury patients, who have a low risk for intracranial hemorrhage and are seen within 6 h of injury. Two recent publications have shown that S100B in the context of the Scandinavian guidelines is a safe and cost-effective means of reducing the number of unnecessary CTs in head trauma (13, 14). The clear caveat and applicability-weakening factor of S100B is the sensitivity to extracranial injuries and a short metabolic half-life (11). Theoretically, GFAP is superior to S100B with a more brain injury-specific profile and a longer half-life (15–17).
A substantial body of literature suggests that serum GFAP elevations are associated with acute brain pathology, as evidenced by head CT, across the spectrum of brain injury severity (17–22). Increases in serum levels are detectable within hours of injury and stay elevated for days, a temporal profile that makes GFAP detection potentially very practical and useful in the emergency setting (22, 23).
Objectives
We conducted a systematic review of the usefulness of blood-based GFAP for predicting acute trauma-related CT-positive intracranial lesions. The main objective was to summarize the current evidence on blood-based GFAP as a potential screening test for acute CT-positive intracranial lesions following head trauma. Our secondary objective was to examine whether or not GFAP was clearly associated with intracranial lesions in patients with mild traumatic brain injury (TBI).
Research Question
Is increased blood-based GFAP consistently associated with acute (within 24 h post-injury) CT-detectible intracranial trauma lesions following head injury?
Methods
Participants
Eligibility criteria included: (i) population: human head and brain injuries of all severities and age groups; (ii) intervention: blood-based GFAP measurement ≤24 h post-injury; and (iii) outcome: acute traumatic lesion on non-contrast head CT ≤24 h post-injury. We focused on emergency management and, therefore, applied the time cutoff of 24 h and examined only blood-based GFAP.
Systematic Review Protocol
The review was registered with PROSPERO (registration number: CRD42016049452) and adhered to the PRISMA guidelines (24).
Search Strategy
We screened MEDLINE, EMBASE, PsychInfo, CINAHL, Web of Science, the Cochrane Database, Scopus, Clinical Trials, OpenGrey, ResearchGate, and the reference lists of eligible publications for original contributions published between January 1, 1980, and October 12, 2016. In order to make the review more comprehensive, we updated the literature search on the 10th of February 2017, and thus included original publications published between January 1, 1980 and January 31, 2017. A senior research librarian performed the literature search. The key search terms included: head injur*; head trauma; brain injur*; brain trauma; brain damage; brain contusion*; brain laceration*; brain hemorrhage*; concussion*; MTBI*; TBI*; craniocerebral injur*; craniocerebral trauma; craniocerebral damage; intracranial hemorrhage*; intracranial hematoma; intracranial lesion*; intracranial abnormalit*; intracerebral hemorrhage, traumatic; hematoma, subdural, acute; subdural hematoma; hematoma, epidural, cranial; epidural hematoma; cerebral hemorrhage, traumatic; cerebral hematoma, traumatic; subarachnoid hemorrhage; diffuse axonal injur*; glial fibrillary acidic protein*; astroprotein; glial fibrillary acid protein; glial intermediate filament protein; GFA-protein; GFAP; and GFAP-BDP. The detailed search strategy is presented in an online supplement (Appendix S1 in Supplementary Material). We included only studies published in English.
Screening and Eligibility
The web-based reference management program Mendeley© (Mendeley Ltd., London, UK) was used for publication screening. Before uploading the references to Mendeley©, duplicate publications (duplicate exclusion: n = 2,232; included for screening: n = 2,474) were excluded by our librarian. Three authors (Teemu M. Luoto, Rahul Raj, and Jussi P. Posti; hereafter assessing authors) completed the publication screening, data extraction, and quality assessment of eligible articles. Each of the included publications (n = 2,474) was initially screened for eligibility based on the title and abstract by two of these assessing authors. After initial screening, 2,423 publications were excluded because they did not fulfill the aforementioned eligibility criteria. Two assessing authors also independently reviewed the full-text versions of a subset of primarily eligible articles (n = 51). Conflicts over inclusion were resolved by involving the third assessor.
Data Extraction and Analysis
Teemu M. Luoto, Rahul Raj, and Jussi P. Posti completed the publication screening, data extraction, and quality assessment of eligible articles. The data extraction form included the following variables: study design and setting, study country and number of sites used, method of GFAP analytics, head CT findings (gradings and percentage of abnormal findings), time intervals between injury and CT/GFAP assessments, extracranial injuries, samples sizes (TBI patient and/or controls), gender and age distributions, GFAP concentrations, and results of relevant statistical tests. All the extracted data were collected on a group level, no individual case level data were available. On an individual article level, two of the assessing authors extracted data independently, and the third reviewed and verified these extraction results. Conflicts over results were resolved by consensus. The scientific quality (including potential sources of bias) of each article was evaluated with the Newcastle–Ottawa Scale (25). The level of evidence was rated according to the Oxford Center of Evidence-based Medicine (26) and GRADE rankings (27). To obtain and confirm missing data (e.g., on study methodology), the investigators of the included publications were contacted by email. Some publications were comprised of overlapping samples, which was acknowledged in the qualitative synthesis. We defined head injury of any severity as the labeling criteria for a case. For example, some studies classified head trauma patients with negative CT scans as “controls.” For this systematic review, these patients were assigned as head trauma cases instead of controls. Results from adult and pediatric studies are reported separately.
Results
Study Selection and Characteristics
The PRISMA flow chart is presented in Figure 1. A total of 27 articles [adult studies: 22 (81%), and pediatric studies: 5 (19%)] were included. Table 1 summarizes the main characteristics of the included studies.
For this review, we re-classified seven (19%) studies as cohort studies although the original authors named those study designs case–control (46). The investigators in 17 (63%) publications did not explicitly report the study design. Of the included studies, 13 (48%) were case–control and 14 (52%) cohort studies. All of the included studies were observational; none of the studies had a diagnostic test design (the accuracy of exact GFAP levels in distinguishing CT-positives from CT-negatives). The majority of the studies were conducted in trauma centers in the United States.
Patient Demographics and Acute Traumatic Lesions
A total of 3,549 participants (68% males) with mild to severe TBI were enrolled in the included studies with individual sample sizes varying between 27 and 325. Control sample sizes varied between 13 and 259 participants, for a sum total of 1,522, of which 54% were males. Orthopedic trauma patients and healthy volunteers were the most commonly enrolled controls; other controls included blood donors and also paid volunteers. The age distribution of the participants was as follows: adult TBI = 15–91 years, pediatric TBI = 0–21 years, adult controls = 18–83 years, and pediatric controls = 0–21 years. Depending on the study, 9–100% of the patients with TBI had acute traumatic lesions on head CT. The Marshall classification (47) was the most commonly used head CT grading system (12 studies, 44%). Many studies reported only gross categories of the traumatic intracranial lesion (i.e., subdural hematoma, contusion, subarachnoid hemorrhage) or only binary CT outcomes (i.e., “positive” or “negative”). A considerable number of studies did not explicitly specify the subtypes of abnormalities that were considered as acute traumatic CT lesions (16 studies, 59%).
Analytical Platforms
The analytical platforms used to measure GFAP were diverse, with 10 different methods employed across the 27 studies. The sandwich enzyme-linked immunosorbent assay (ELISA) manufactured by Banyan Biomarkers (Banyan Biomarkers, Inc., Alachua, FL, USA) was the most frequently used platform (10 studies, 37%). The second most used (7 studies, 26%) platform was BioVendor (BioVendor, Heidelberg, Germany). In two studies, the precise analytic GFAP platform was not stated (considered as two different individual platforms that are not otherwise specified in this review). The analytic methods are shown in Table 1. Most studies used venous blood as their source for GFAP measurement, although two studies used arterial samples (note: an assumption of venous sampling was made if there was no direct reference to arterial sampling). Four studies out of the 10 that used the Banyan Biomarkers assay also analyzed GFAP breakdown products in addition to native GFAP.
Synthesized Findings
There was considerable variability in GFAP levels within the same platform and between platforms (e.g., Banyan Biomarkers vs. BioVendor). For controls (orthopedic injuries and/or healthy participants), the reported GFAP levels varied considerably across studies (adult: range of means = 0.0015–0.057 ng/mL, range of medians = 0–0.0008 ng/mL; and pediatric: range of medians = 0.01–0.03 ng/mL). Between studies, orthopedic controls did not appear to show consistently higher GFAP levels compared to healthy controls. There was only one study that reported results for both non-injured controls and non-TBI trauma controls. In this particular study (32), trauma controls had higher GFAP levels than uninjured control subjects (mean = 0.203, median = 0.216; vs. mean = 0.038, median = 0.010, respectively). The GFAP levels of the TBI patients were consistently higher compared to the controls within studies. Those with CT-positive TBIs (adult: range of means = 0.00677–2.86 ng/mL, range of medians = 0.1–1.9 ng/mL; pediatric: range of medians = 0.73–1.19 ng/mL) had higher GFAP levels than CT-negative cases (adult: range of means = 0.00007–0.26 ng/mL, range of medians = 0.0078–0.33 ng/mL; and pediatric: range of medians = 0.18–1.25 ng/mL). Figure 2 summarizes the mean/median GFAP findings of the individual studies.
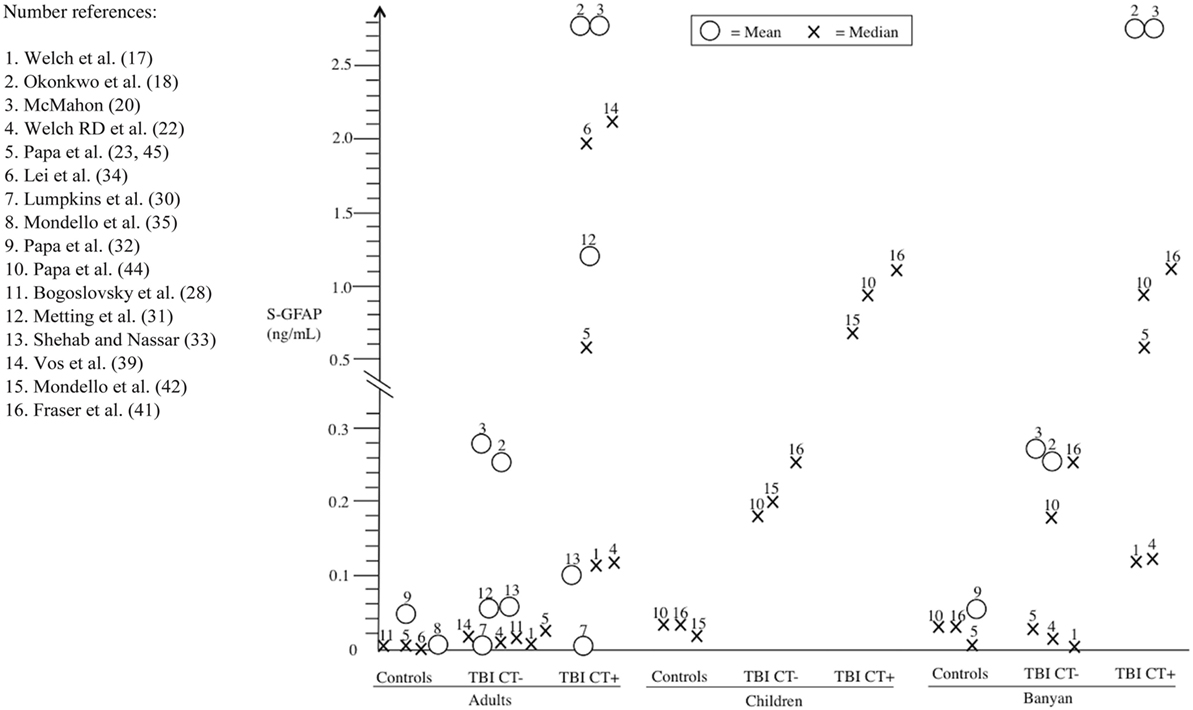
Figure 2. Serum glial fibrillary acidic protein (GFAP) findings (means and medians) from individual studies stratified into different subgroups: (i) controls, (ii) CT-negative traumatic brain injury (TBI) (TBI CT−), and (iii) CT-positive TBIs (TBI CT+). For comparison, the findings are subdivided into adult and pediatric subpopulations, and also results of the most commonly used Banyan Biomarkers (Banyan Biomarkers, Inc., Alachua, FL, USA) assay are presented separately.
Twenty-four (89%) studies reported a positive association between the GFAP level and traumatic lesions seen on head CT. Higher GFAP levels were related to lesion severity in the majority of studies that examined lesion severity (21, 30, 32, 34–36). Additionally, mass lesions and surgically treated lesions were associated with higher GFAP levels than diffuse lesions in all five studies where this comparison was made (21, 30, 32, 35, 36). Thirteen studies reported receiver operating curves on how well GFAP was able to discriminate between head trauma patients with positive vs. negative CT scans. The areas under the receiver operating curves varied between 0.74 and 0.98. Eight studies reported binary classification (CT-positive vs. CT-negative) test results for GFAP (Table 1). Six adult (16–18, 20, 30, 32) and two pediatric (44, 45) studies examined the sensitivity of GFAP cutoff values for identifying intracranial lesions. A pediatric GFAP cutoff value (0.15 mg/mL) was derived from these two studies (44, 45) although it should be noted that the two studies were comprised of a partially overlapping sample. The adult GFAP cutoff values were more inconsistent than the pediatric ones and ranged between 0.001 and 1.66 ng/mL. One adult study (16) established a GFAP cutoff value of 0.067 ng/mL with a sensitivity of 100% and a specificity of 55%.
Level of Evidence and Risk of Bias
No study was excluded from the review due to a significant source of bias. The Newcastle–Ottawa Scale and the level of evidence (the Oxford Center of Evidence-based Medicine) results are presented in Table 2. The mean level of evidence was 3.6 (17 studies classified as level 4 and 10 studies classified as level 3). On the Newcastle-Ottawa Scale, the average ratings for the 27 studies were as follows: selection (0–4) = 2.9, comparability (0–2) = 1.0, and outcome (0–3) = 3.0. The GRADE ranking for the level of evidence was C. The rating was based on observational studies with fairly consistent results. However, the level of evidence was downgraded, because of partly incomplete data reporting, and the absence of effect estimates.
Findings of Studies Examining Mostly Mild TBI
Screening for possible CT-positive trauma-related intracranial lesions is clinically relevant among those with mild head trauma because many of these injuries could be managed without CT imaging. Therefore, the findings of studies examining mostly mild TBI are summarized separately. There were 15 adult studies (15–23, 28–33) and 3 pediatric studies (42, 44, 45) that included mostly subjects with mild TBIs, although the samples tended to be heterogeneous and included some patients with moderate or severe TBIs, too. Additionally, the operational criteria for mild TBI were not homogenous among the included studies that examined mostly mild TBIs. This methodological heterogeneity hindered the possibility of organizing and summarizing the results in a combined manner. The research designs were not diagnostic studies of consecutive cohorts of “mild head trauma” cases that employed GFAP as the experimental diagnostic test for intracranial abnormalities compared to a CT or MRI gold standard. Of the included studies, 10 (56%) were case–control and 8 (44%) cohort studies. A total of 2,899 participants were enrolled in the studies with individual study sample sizes varying between 34 and 325 [adults: n = 2,543 (88%); pediatric: n = 356 (12%)]. Control sample sizes varied between 20 and 259 participants, for a total of 1,207 [adults: n = 1,065 (88%); pediatric: n = 142 (12%)]. Orthopedic trauma patients and healthy volunteers were the most commonly enrolled controls; other controls included patients treated for trivial reason other than head injury. Six different analytical platforms were employed across the 18 studies. The sandwich ELISA manufactured by Banyan Biomarkers was the most frequently used platform (10 studies, 56%). There was considerable variability in GFAP levels within the same platform, within the same analytic method, and between platforms and this was irrespective of the studied patient and cohort type. Seventeen (94%) of those studies reported a positive association between the GFAP level and the head CT trauma lesions. A considerable number of studies did not explicitly specify the subtypes of abnormalities that were considered as acute traumatic CT lesions (n = 6, 33%). Twelve (67%) studies reported receiver operating curves on how well GFAP discriminated CT-positive TBI patients from CT-negative ones. The areas under the receiver operating curves varied between 0.74 and 0.98. Eight (44%) studies reported binary classification (CT-positive vs. CT-negative) test results for GFAP. Six adult (16–18, 20, 30, 32) and two pediatric (44, 45) studies examined GFAP cutoff values for head CT positivity. The mean level of evidence was 3.4 (8 studies classified as level 4 and 10 studies classified as level 3). On the Newcastle–Ottawa Scale, the average ratings for the 18 studies were as follows: selection (0–4) = 3.0, comparability (0–2) = 1.3, and outcome (0–3) = 2.9. The GRADE ranking for the level of evidence was C.
Discussion
Summary of Main Findings
Blood levels of GFAP were associated with acute traumatic lesions on head CT. GFAP levels usually were associated with the CT-detectible lesion severity (15–23, 28, 30–40, 42, 44, 45), with surgical lesions (i.e., mass-occupying hematomas/contusions requiring craniotomy) generally showing the highest elevations of serum GFAP (21, 30, 32, 34–36). These findings were consistent across the age spectrum. Based on our review, GFAP holds promise as a potential screening test for acute CT-detectible traumatic brain lesions. However, clearly defined cutoff values (CT-negative vs. CT-positive) for specific GFAP platforms have not been established. The literature has significant methodological limitations that do not allow us to determine the sensitivity or specificity of GFAP for identifying any CT abnormality, or clinically important CT abnormalities, following mild TBI. Well-designed diagnostic test studies for GFAP are needed.
Findings in Pediatric Samples
Children were the focus of interest in five studies (41–45). Out of these five studies only three investigated serum GFAP levels in relation to CT-negative and CT-positive TBIs (42, 44, 45). In these three pediatric studies, the results were in line with the adult findings. However, the small number of pediatric studies casts some doubt on the generalizability and applicability of these findings. Comparisons between adults and pediatric posttraumatic serum GFAP dynamics have not yet been done to our knowledge. However, normal GFAP levels of healthy children are most likely lower than those of healthy adults in the cerebrospinal fluid (48). In our review, the distribution of GFAP concentrations among adult TBI patients differed somewhat from the pediatric counterparts. The pediatric values were more consistent and the measured range was narrower than with adults (see Table 1; Figure 2). One reason for this is that only two different analytical platforms were used in the positive pediatric studies. In the light of the current evidence, we cannot extrapolate meaningfully as to other factors that account for the difference in adult and pediatric GFAP levels.
Negative Findings
Three (29, 41, 43) out of the 27 studies did not find any relation between acute (≤24 h post-injury) serum GFAP levels and traumatic head CT findings. Two (41, 43) of these negative studies consisted of pediatric patients with severe TBI. In the first study, Fraser et al. (41) examined whether arterial GFAP was related to traumatic lesions in a sample exclusively consisting of CT-positive severe TBIs. In the second study, Zurek and Fedora (43) compared different Marshall score grades to serum GFAP (Marshall grade distributions not available) and found no relation between GFAP level and Marshall grades. These two negative pediatric studies did not examine GFAP levels in relation to head CT positivity and negativity. They only considered CT-positive cases. Furthermore, among severe TBIs the identification of intracranial traumatic lesions with serum GFAP is not clinically relevant because these patients always require an emergency head CT as part of their routine management. In the only negative adult study, Buonora and co-authors (29) did not find an association between CT-detectible intracranial trauma lesions and GFAP in a case–control study consisting of mild to severe TBIs. The null finding was likely because most of the GFAP levels were below the lower limit of quantification (0.27 ng/mL) and detection (0.21 ng/mL) for their assay. The lower limits of quantification and detection of Buonora’s study were multiple times higher than in other studies.
Methodological Considerations
We were not able to extrapolate cutoff values or percent increases in GFAP that consistently predict intracranial pathology based on the data presented in the articles. There was considerable variability in measured GFAP levels that was likely related to the analytic GFAP platform employed. Considerable variability in GFAP levels between studies employing the same GFAP platforms was also apparent not only in the TBI groups, but in the orthopedically injured and even within the normal healthy control samples. Time after injury may also be a confounding factor. GFAP was measured from as early as 15 min (40) and as late as to 24 h after TBI within individual studies. GFAP temporal dynamics were examined by only two studies (22, 23). In one study, patients with a positive head CT showed an average GFAP elevation of 3.7% per hour over the first 24 h compared to head trauma cases with negative CTs (22). In the other study, serum GFAP levels were reported to peak 20 h after TBI among those with intracranial lesions detected on CT, and slowly decline over 72 h following injury (23). In most of the included studies, a detailed methodology of sample processing was not reported. This hinders the ability to compare possible factors affecting the GFAP results. In two studies, blood samples were taken from an arterial line and no specific GFAP levels were reported. Whether arterial and venous GFAP levels are comparable is unknown.
Common Data Elements (CDEs) aid in harmonizing neuroimaging data across studies and sites (49). In the studies included in our review, very few utilized the National Institute of Health’s CDEs and explicitly defined which lesions were designated as traumatic. Half of the studies used the Marshall grading to classify head CT findings (15, 21, 28, 31, 34–40, 43). Overall, the studies did not clearly define which intracranial lesions were ascertained as acute TBI abnormalities. For example, some studies [e.g., Mondello et al. (42)] considered skull fractures as an acute traumatic intracranial finding, whereas other studies excluded these lesions. Along with possible discrepancies in trauma lesion interpretation, the technical details of head CT imaging were poorly described. The applied slice thickness and image orientations (sagittal, axial, and coronal) were almost universally lacking. Furthermore, the interpreter (e.g., on call radiologist, neuroradiologist, neurosurgeon) of the head CT images was not stated in the majority of studies. As defined in our inclusion criteria, head CT imaging was performed within 24 h after injury in the included studies. In two studies (17, 43), neuroimaging was conducted within 6 h after injury. Hyperacute (initial hours after injury) imaging can result in false negative scans; for example, some contusions do not demarcate well in the first few hours after trauma.
GFAP in the Context of Other Diseases and Orthopedic Injuries
Glial fibrillary acidic protein elevations are not specific to TBI; other acute, destructive central nervous system lesions will also raise levels—albeit modestly so. In an exploratory study of a broad spectrum of neurological diseases, GFAP levels were very low in most patients (50). Intracerebral hemorrhage, as a result of cerebrovascular disease, is associated with elevated levels of GFAP (51, 52). Furthermore, high-grade infiltrating tumors (53, 54) and demyelinating diseases [e.g., multiple sclerosis (55)] have also been shown to result in increased serum GFAP. Chronic neurological conditions (e.g., migraines and epilepsy), however, do not appear to influence GFAP levels to a degree that would be expected to confound the usefulness of GFAP as a TBI biomarker (although there are few studies relating to chronic conditions) (50).
It has been reported that injuries outside the brain can also elevate serum GFAP concentrations (56). GFAP has been detected in non-glial and non-central nervous system cells, such as Schwann cells, chondrocytes, fibroblasts, myoepithelial cells, lymphocytes, and liver stellate cells. These can be a source of released GFAP after extremity and bodily trauma. Only a third of the studies accounted for concurrent orthopedic injuries among TBI patients (16, 20, 29–31, 33, 35, 41). The association between GFAP and head CT findings (i.e., CT-positives had higher GFAP levels than CT-negatives of non-head injured controls, and mass lesion were related to higher GFAP levels than diffuse lesions) was present in those studies involving patients with orthopedic injury. Only two adult studies (23, 32) and two pediatric studies (44, 45) reported GFAP levels of trauma controls (median = 0.008, median = 0.03, and median = 0.3 ng/mL, respectively). The pediatric orthopedic control values were derived from mostly the same control cohort. One study (32) examined both non-injured controls and trauma controls; orthopedic trauma seemed to increase acute GFAP levels (non-injured controls: mean = 0.038, 95% CI = 0.029–0.047, median = 0.010, IQR = 0.050; vs. non-TBI trauma controls: mean = 0.203, 95% CI = 0.048–0.357, median = 0.216, IQR = 0.275). To date, this is the only study that directly compares orthopedic injury subjects to non-injured controls. Based on this limited amount of available data, there appears to be an association between head trauma and GFAP levels in studies comparing to orthopedically injured samples, but we cannot draw any solid conclusions on the effects of orthopedic injury on serum GFAP levels.
Strengths and Limitations
Our review has several strengths. We applied a comprehensive literature search protocol to both the pediatric and adult literature. Gray literature was examined separately. The reference lists of all the eligible articles were screened for potential unidentified new articles. This screening identified no new eligible articles. The literature search protocol can, therefore, be considered inclusive.
There is a potential for publication bias in our conclusions because we only reviewed published articles; studies with negative findings are less likely to be published. Additionally, non-English publications were not included. Most importantly, we could not pool data across studies and meta-analyze GFAP levels. We were unable to conduct a quantitative analysis due to the methodological heterogeneity between studies (e.g., differences in inclusion criteria and reporting of CT findings, variance in GFAP levels among assays), and constraints in the reported principal summary measures (i.e., different measures of central tendency and variance were reported, and no effect sizes were reported). Although measures were taken to gather missing data, the amount of unattainable results was considerable (e.g., GFAP levels of CT-positive cases). The review included articles that utilized partially overlapping patient and/or control samples. Principal investigators of these studies were contacted to clarify the extent of sample overlap. Unfortunately, these investigator inquiries were only partially answered. It was very difficult to determine the association between GFAP levels and CT lesions in patients with mild TBIs because the research designs often included heterogeneous injury severity samples and diverse definitions of injury severity.
Another clinically important limitation of the overall literature is that the sensitivity of the different GFAP assays to epidural hematomas, and the temporal dynamics of GFAP in relation to the evolution of an epidural hematoma, is unknown. Some epidural hematomas, especially in the early stages of evolution, are associated with only mild or modest amounts of parenchymal brain injury—and the extent to which GFAP is elevated in those cases is not known.
Classification of the Level of Evidence
Based on the classification of the Oxford Center of Evidence-Based Medicine (1 to 5), the level of evidence of the individual studies was 3 or 4 (average = 3.6). The GRADE ranking for the level of evidence is C. A major limitation in most studies was the use of a control group that may not have been representative of the case population. In general, the controls were derived from a completely different population than the cases and limited comparison was performed on the basic demographics (e.g., age, gender, prior health) of these groups (controls vs. TBIs). Case selection was also a point of concern because studies applied widely different inclusion and exclusion criteria. Additionally, very little data (i.e., age, gender, injury mechanism, injury severity, reason for exclusion) was given on the population screened for study inclusion. Thus, the extent to which the results generalize well to a broader population is unknown. The review consisted of studies conducted in multiple countries with different health-care systems. Nevertheless, the main finding that GFAP correlated with CT-detectible intracranial trauma lesions was consistent between these studies. It seems that the results are generalizable and applicable rather globally.
Future Directions
In the future, larger studies are needed to replicate, extend, and refine these findings. Current ongoing large-scale initiatives, CENTER-TBI (57), and TRACK-TBI (58), are important in verifying the potential of GFAP as a marker in acute TBI triage. From a practical point of view, a rapid capillary blood-based GFAP screening test would be of benefit for patient management in a pre-hospital environment (e.g., sideline assessment in sports and emergency medical services). What is lacking, and clearly needed, is an assay with clearly defined cutoff values for abnormality that has excellent sensitivity and at least good specificity in multiple clinical groups, including those with orthopedic injuries and those with a wide range of pre-existing neurological and medical problems. It is common for people with pre-existing medical, neurological, and neurodegenerative diseases to present to the emergency department with head trauma. Diagnostic studies with clear GFAP cutoffs are needed before possible clinical implementation.
Future well-designed diagnostic studies of GFAP would examine the appropriate spectrum of patients (i.e., the group the test will be applied to in a real-world setting); carefully define the “diagnosis” or condition of interest (e.g., a clinically important lesion on CT, any intracranial lesion on CT, or any traumatic lesion on MRI); apply the neuroimaging to all subjects; use independent or blind comparison with the imaging results; and present sensitivity, specificity, and likelihood ratios (and positive and negative predictive values if the prevalence of intracranial abnormalities in the sample studied is close to the prevalence of intracranial abnormalities in the population of interest). Future researchers should carefully describe their findings in a manner that allows physicians to determine if they can use the results in their work setting, whether the results apply to the patients that they see, and whether the results would actually change clinical practice (e.g., ordering fewer head CT scans). Future researchers are also encouraged to assemble and present case series involving epidural hematomas and carefully examine their GFAP levels and temporal dynamics.
Conclusion
In conclusion, GFAP is predictive of CT-positive brain damage in acute head injuries. GFAP increases within hours following injury in peripheral blood. A limited number of studies suggest the elevation may peak at 20–24 h post-injury, and thus consideration of temporal dynamics may improve diagnostic sensitivity and specificity. Although promising, at the present time there is not enough evidence to suggest that GFAP can be used clinically as a reliable discriminant of CT-positive and CT-negative brain injury. With future diagnostic research and refinements, GFAP may have the potential to be used as part of a comprehensive diagnostic algorithm to identify patients with intracranial abnormalities.
Author Contributions
TL, RR, JP, AG, WP, and GI conceived and designed the study. TL, RR, and JP completed the publication screening, data extraction, and quality assessment of the eligible publications. TL drafted the manuscript, and all authors contributed substantially to its revision. TL takes responsibility for the paper as a whole.
Conflict of Interest Statement
The authors alone are responsible for the content and writing of the paper. The authors report no competing financial interests.
Acknowledgments
The authors thank senior research librarian Debbie Booth for performing the literature search.
Supplementary Material
The Supplementary Material for this article can be found online at http://www.frontiersin.org/article/10.3389/fneur.2017.00652/full#supplementary-material.
References
1. Brenner DJ, Hall EJ. Computed tomography – an increasing source of radiation exposure. N Engl J Med (2007) 357:2277–84. doi:10.1056/NEJMra072149
2. Power SP, Moloney F, Twomey M, James K, O’Connor OJ, Maher MM. Computed tomography and patient risk: facts, perceptions and uncertainties. World J Radiol (2016) 8:902–15. doi:10.4329/wjr.v8.i12.902
3. Jagoda AS, Bazarian JJ, Bruns JJ Jr, Cantrill SV, Gean AD, Howard PK, et al. Clinical policy: neuroimaging and decisionmaking in adult mild traumatic brain injury in the acute setting. Ann Emerg Med (2008) 52:714–48. doi:10.1016/j.annemergmed.2008.08.021
4. Haydel MJ, Preston CA, Mills TJ, Luber S, Blaudeau E, Deblieux PM. Indications for computed tomography in patients with minor head injury. N Engl J Med (2000) 343:100–5. doi:10.1056/NEJM200007133430204
5. Stiell IG, Wells GA, Vandemheen K, Clement C, Lesiuk H, Laupacis A, et al. The Canadian CT Head Rule for patients with minor head injury. Lancet (2001) 357:1391–6. doi:10.1016/S0140-6736(00)04561-X
6. Mathews JD, Forsythe AV, Brady Z, Butler MW, Goergen SK, Byrnes GB, et al. Cancer risk in 680,000 people exposed to computed tomography scans in childhood or adolescence: data linkage study of 11 million Australians. BMJ (2013) 346:f2360. doi:10.1136/bmj.f2360
7. Isokuortti H, Iverson GL, Kataja A, Brander A, Ohman J, Luoto TM. Who gets head trauma or recruited in mild traumatic brain injury research? J Neurotrauma (2016) 33:232–41. doi:10.1089/neu.2015.3888
8. Smith-Bindman R, Lipson J, Marcus R, Kim KP, Mahesh M, Gould R, et al. Radiation dose associated with common computed tomography examinations and the associated lifetime attributable risk of cancer. Arch Intern Med (2009) 169:2078–86. doi:10.1001/archinternmed.2009.427
9. Kawata K, Liu CY, Merkel SF, Ramirez SH, Tierney RT, Langford D. Blood biomarkers for brain injury: what are we measuring? Neurosci Biobehav Rev (2016) 68:460–73. doi:10.1016/j.neubiorev.2016.05.009
10. Zetterberg H, Blennow K. Fluid biomarkers for mild traumatic brain injury and related conditions. Nat Rev Neurol (2016) 12:563–74. doi:10.1038/nrneurol.2016.127
11. Thelin EP, Nelson DW, Bellander BM. A review of the clinical utility of serum S100B protein levels in the assessment of traumatic brain injury. Acta Neurochir (Wien) (2017) 159:209–25. doi:10.1007/s00701-016-3046-3
12. Unden J, Ingebrigtsen T, Romner B, Scandinavian Neurotrauma C. Scandinavian guidelines for initial management of minimal, mild and moderate head injuries in adults: an evidence and consensus-based update. BMC Med (2013) 11:50. doi:10.1186/1741-7015-11-50
13. Unden L, Calcagnile O, Unden J, Reinstrup P, Bazarian J. Validation of the Scandinavian guidelines for initial management of minimal, mild and moderate traumatic brain injury in adults. BMC Med (2015) 13:292. doi:10.1186/s12916-015-0533-y
14. Calcagnile O, Anell A, Unden J. The addition of S100B to guidelines for management of mild head injury is potentially cost saving. BMC Neurol (2016) 16:200. doi:10.1186/s12883-016-0723-z
15. Honda M, Tsuruta R, Kaneko T, Kasaoka S, Yagi T, Todani M, et al. Serum glial fibrillary acidic protein is a highly specific biomarker for traumatic brain injury in humans compared with S-100B and neuron-specific enolase. J Trauma (2010) 69:104–9. doi:10.1097/TA.0b013e3181bbd485
16. Papa L, Silvestri S, Brophy GM, Giordano P, Falk JL, Braga CF, et al. GFAP out-performs S100beta in detecting traumatic intracranial lesions on computed tomography in trauma patients with mild traumatic brain injury and those with extracranial lesions. J Neurotrauma (2014) 31:1815–22. doi:10.1089/neu.2013.3245
17. Welch RD, Ayaz SI, Lewis LM, Unden J, Chen JY, Mika VH, et al. Ability of serum glial fibrillary acidic protein, ubiquitin C-terminal hydrolase-L1, and S100B to differentiate normal and abnormal head computed tomography findings in patients with suspected mild or moderate traumatic brain injury. J Neurotrauma (2016) 33:203–14. doi:10.1089/neu.2015.4149
18. Okonkwo DO, Yue JK, Puccio AM, Panczykowski DM, Inoue T, Mcmahon PJ, et al. GFAP-BDP as an acute diagnostic marker in traumatic brain injury: results from the prospective transforming research and clinical knowledge in traumatic brain injury study. J Neurotrauma (2013) 30:1490–7. doi:10.1089/neu.2013.2883
19. Diaz-Arrastia R, Wang KK, Papa L, Sorani MD, Yue JK, Puccio AM, et al. Acute biomarkers of traumatic brain injury: relationship between plasma levels of ubiquitin C-terminal hydrolase-L1 and glial fibrillary acidic protein. J Neurotrauma (2014) 31:19–25. doi:10.1089/neu.2013.3040
20. McMahon PJ, Panczykowski DM, Yue JK, Puccio AM, Inoue T, Sorani MD, et al. Measurement of the glial fibrillary acidic protein and its breakdown products GFAP-BDP biomarker for the detection of traumatic brain injury compared to computed tomography and magnetic resonance imaging. J Neurotrauma (2015) 32:527–33. doi:10.1089/neu.2014.3635
21. Posti JP, Takala RS, Runtti H, Newcombe VF, Outtrim J, Katila AJ, et al. The levels of glial fibrillary acidic protein and ubiquitin C-terminal hydrolase-L1 during the first week after a traumatic brain injury: correlations with clinical and imaging findings. Neurosurgery (2016) 79:456–64. doi:10.1227/NEU.0000000000001226
22. Welch RD, Ellis M, Lewis LM, Ayaz SI, Mika VH, Millis S, et al. Modeling the kinetics of serum glial fibrillary acidic protein, ubiquitin carboxyl-terminal hydrolase-L1, and S100B concentrations in patients with traumatic brain injury. J Neurotrauma (2017) 34(11):1957–71. doi:10.1089/neu.2016.4772
23. Papa L, Brophy GM, Welch RD, Lewis LM, Braga CF, Tan CN, et al. Time course and diagnostic accuracy of glial and neuronal blood biomarkers GFAP and UCH-L1 in a large cohort of trauma patients with and without mild traumatic brain injury. JAMA Neurol (2016) 73:551–60. doi:10.1001/jamaneurol.2016.0039
24. Liberati A, Altman DG, Tetzlaff J, Mulrow C, Gotzsche PC, Ioannidis JP, et al. The PRISMA statement for reporting systematic reviews and meta-analyses of studies that evaluate healthcare interventions: explanation and elaboration. BMJ (2009) 339:b2700. doi:10.1136/bmj.b2700
25. Wells G, Shea B, O’Connell D, Peterson J, Welch V, Losos M, et al. The Newcastle-Ottawa Scale (NOS) for Assessing the Quality of Nonrandomised Studies in Meta-analyses. (2013). Available from: http://www.ohri.ca/programs/clinical_epidemiology/oxford.asp
26. The Oxford Centre of Level of Evidence. The Oxford Levels of Evidence 2. (2017). Available: http://www.cebm.net/index.aspx?o=5653
27. Guyatt GH, Oxman AD, Vist GE, Kunz R, Falck-Ytter Y, Alonso-Coello P, et al. GRADE: an emerging consensus on rating quality of evidence and strength of recommendations. BMJ (2008) 336:924–6. doi:10.1136/bmj.39489.470347.AD
28. Bogoslovsky T, Wilson D, Chen Y, Hanlon D, Gill J, Jeromin A, et al. Increases of plasma levels of glial fibrillary acidic protein, Tau, and amyloid beta up to 90 days after traumatic brain injury. J Neurotrauma (2017) 34(1):66–73. doi:10.1089/neu.2015.4333
29. Buonora JE, Yarnell AM, Lazarus RC, Mousseau M, Latour LL, Rizoli SB, et al. Multivariate analysis of traumatic brain injury: development of an assessment score. Front Neurol (2015) 6:68. doi:10.3389/fneur.2015.00068
30. Lumpkins KM, Bochicchio GV, Keledjian K, Simard JM, Mccunn M, Scalea T. Glial fibrillary acidic protein is highly correlated with brain injury. J Trauma (2008) 65:778–82; discussion 782–4. doi:10.1097/TA.0b013e318185db2d
31. Metting Z, Wilczak N, Rodiger LA, Schaaf JM, Van Der Naalt J. GFAP and S100B in the acute phase of mild traumatic brain injury. Neurology (2012) 78:1428–33. doi:10.1212/WNL.0b013e318253d5c7
32. Papa L, Lewis LM, Falk JL, Zhang Z, Silvestri S, Giordano P, et al. Elevated levels of serum glial fibrillary acidic protein breakdown products in mild and moderate traumatic brain injury are associated with intracranial lesions and neurosurgical intervention. Ann Emerg Med (2012) 59:471–83. doi:10.1016/j.annemergmed.2011.08.021
33. Shehab HA, Nassar YH. Neuromarkers as diagnostic adjuvant to cranial CT in closed traumatic brain injury patients admitted to ICU: a preliminary comparative study. Egypt J Anaesth (2010) 26:267–72. doi:10.1016/j.egja.2010.05.001
34. Lei J, Gao G, Feng J, Jin Y, Wang C, Mao Q, et al. Glial fibrillary acidic protein as a biomarker in severe traumatic brain injury patients: a prospective cohort study. Crit Care (2015) 19:362. doi:10.1186/s13054-015-1081-8
35. Mondello S, Papa L, Buki A, Bullock MR, Czeiter E, Tortella FC, et al. Neuronal and glial markers are differently associated with computed tomography findings and outcome in patients with severe traumatic brain injury: a case control study. Crit Care (2011) 15:R156. doi:10.1186/cc10286
36. Mondello S, Jeromin A, Buki A, Bullock R, Czeiter E, Kovacs N, et al. Glial neuronal ratio: a novel index for differentiating injury type in patients with severe traumatic brain injury. J Neurotrauma (2012) 29:1096–104. doi:10.1089/neu.2011.2092
37. Pelinka LE, Kroepfl A, Leixnering M, Buchinger W, Raabe A, Redl H. GFAP versus S100B in serum after traumatic brain injury: relationship to brain damage and outcome. J Neurotrauma (2004) 21:1553–61. doi:10.1089/neu.2004.21.1553
38. Pelinka LE, Kroepfl A, Schmidhammer R, Krenn M, Buchinger W, Redl H, et al. Glial fibrillary acidic protein in serum after traumatic brain injury and multiple trauma. J Trauma (2004) 57:1006–12. doi:10.1097/01.TA.0000108998.48026.C3
39. Vos PE, Jacobs B, Andriessen TM, Lamers KJ, Borm GF, Beems T, et al. GFAP and S100B are biomarkers of traumatic brain injury: an observational cohort study. Neurology (2010) 75:1786–93. doi:10.1212/WNL.0b013e3181fd62d2
40. Vos PE, Lamers KJ, Hendriks JC, Van Haaren M, Beems T, Zimmerman C, et al. Glial and neuronal proteins in serum predict outcome after severe traumatic brain injury. Neurology (2004) 62:1303–10. doi:10.1212/01.WNL.0000120550.00643.DC
41. Fraser DD, Close TE, Rose KL, Ward R, Mehl M, Farrell C, et al. Severe traumatic brain injury in children elevates glial fibrillary acidic protein in cerebrospinal fluid and serum. Pediatr Crit Care Med (2011) 12:319–24. doi:10.1097/PCC.0b013e3181e8b32d
42. Mondello S, Kobeissy F, Vestri A, Hayes RL, Kochanek PM, Berger RP. Serum concentrations of ubiquitin C-terminal hydrolase-L1 and glial fibrillary acidic protein after pediatric traumatic brain injury. Sci Rep (2016) 6:28203. doi:10.1038/srep28203
43. Zurek J, Fedora M. Dynamics of glial fibrillary acidic protein during traumatic brain injury in children. J Trauma (2011) 71:854–9. doi:10.1097/TA.0b013e3182140c8c
44. Papa L, Zonfrillo MR, Ramirez J, Silvestri S, Giordano P, Braga CF, et al. Performance of glial fibrillary acidic protein in detecting traumatic intracranial lesions on computed tomography in children and youth with mild head trauma. Acad Emerg Med (2015) 22:1274–82. doi:10.1111/acem.12795
45. Papa L, Mittal MK, Ramirez J, Ramia M, Kirby S, Silvestri S, et al. In children and youth with mild and moderate traumatic brain injury, glial fibrillary acidic protein out-performs S100beta in detecting traumatic intracranial lesions on computed tomography. J Neurotrauma (2016) 33:58–64. doi:10.1089/neu.2015.3869
46. Mayo NE, Goldberg MS. When is a case-control study not a case-control study? J Rehabil Med (2009) 41:209–16. doi:10.2340/16501977-0343
47. Marshall LF, Marshall SB, Klauber MR, Van Berkum Clark M, Eisenberg H, Jane JA, et al. The diagnosis of head injury requires a classification based on computed axial tomography. J Neurotrauma (1992) 9(Suppl 1):S287–92.
48. Rosengren LE, Ahlsen G, Belfrage M, Gillberg C, Haglid KG, Hamberger A. A sensitive ELISA for glial fibrillary acidic protein: application in CSF of children. J Neurosci Methods (1992) 44:113–9. doi:10.1016/0165-0270(92)90004-W
49. Duhaime AC, Gean AD, Haacke EM, Hicks R, Wintermark M, Mukherjee P, et al. Common data elements in radiologic imaging of traumatic brain injury. Arch Phys Med Rehabil (2010) 91:1661–6. doi:10.1016/j.apmr.2010.07.238
50. Mayer CA, Brunkhorst R, Niessner M, Pfeilschifter W, Steinmetz H, Foerch C. Blood levels of glial fibrillary acidic protein (GFAP) in patients with neurological diseases. PLoS One (2013) 8:e62101. doi:10.1371/journal.pone.0062101
51. Foerch C, Curdt I, Yan B, Dvorak F, Hermans M, Berkefeld J, et al. Serum glial fibrillary acidic protein as a biomarker for intracerebral haemorrhage in patients with acute stroke. J Neurol Neurosurg Psychiatry (2006) 77:181–4. doi:10.1136/jnnp.2005.074823
52. Zhang J, Zhang CH, Lin XL, Zhang Q, Wang J, Shi SL. Serum glial fibrillary acidic protein as a biomarker for differentiating intracerebral hemorrhage and ischemic stroke in patients with symptoms of acute stroke: a systematic review and meta-analysis. Neurol Sci (2013) 34:1887–92. doi:10.1007/s10072-013-1541-3
53. Brommeland T, Rosengren L, Fridlund S, Hennig R, Isaksen V. Serum levels of glial fibrillary acidic protein correlate to tumour volume of high-grade gliomas. Acta Neurol Scand (2007) 116:380–4. doi:10.1111/j.1600-0404.2007.00889.x
54. Jung CS, Foerch C, Schanzer A, Heck A, Plate KH, Seifert V, et al. Serum GFAP is a diagnostic marker for glioblastoma multiforme. Brain (2007) 130:3336–41. doi:10.1093/brain/awm263
55. Housley WJ, Pitt D, Hafler DA. Biomarkers in multiple sclerosis. Clin Immunol (2015) 161:51–8. doi:10.1016/j.clim.2015.06.015
56. Posti JP, Hossain I, Takala RS, Liedes H, Newcombe V, Outtrim J, et al. Glial fibrillary acidic protein and ubiquitin C-terminal hydrolase-L1 are not specific biomarkers for mild CT-negative traumatic brain injury. J Neurotrauma (2017) 34(7): 1427–38. doi:10.1089/neu.2016.4442
57. Maas AI, Menon DK, Steyerberg EW, Citerio G, Lecky F, Manley GT, et al. Collaborative European NeuroTrauma Effectiveness Research in Traumatic Brain Injury (CENTER-TBI): a prospective longitudinal observational study. Neurosurgery (2015) 76:67–80. doi:10.1227/NEU.0000000000000575
Keywords: brain injury, head injury, computed tomography, glial fibrillary acidic protein, emergency departments
Citation: Luoto TM, Raj R, Posti JP, Gardner AJ, Panenka WJ and Iverson GL (2017) A Systematic Review of the Usefulness of Glial Fibrillary Acidic Protein for Predicting Acute Intracranial Lesions following Head Trauma. Front. Neurol. 8:652. doi: 10.3389/fneur.2017.00652
Received: 23 September 2017; Accepted: 20 November 2017;
Published: 04 December 2017
Edited by:
Niklas Marklund, Lund University, SwedenReviewed by:
Bridgette D. Semple, University of Melbourne, AustraliaRamon Diaz-Arrastia, University of Pennsylvania, United States
Copyright: © 2017 Luoto, Raj, Posti, Gardner, Panenka and Iverson. This is an open-access article distributed under the terms of the Creative Commons Attribution License (CC BY). The use, distribution or reproduction in other forums is permitted, provided the original author(s) or licensor are credited and that the original publication in this journal is cited, in accordance with accepted academic practice. No use, distribution or reproduction is permitted which does not comply with these terms.
*Correspondence: Teemu M. Luoto, dGVlbXUubHVvdG9AcHNocC5maQ==