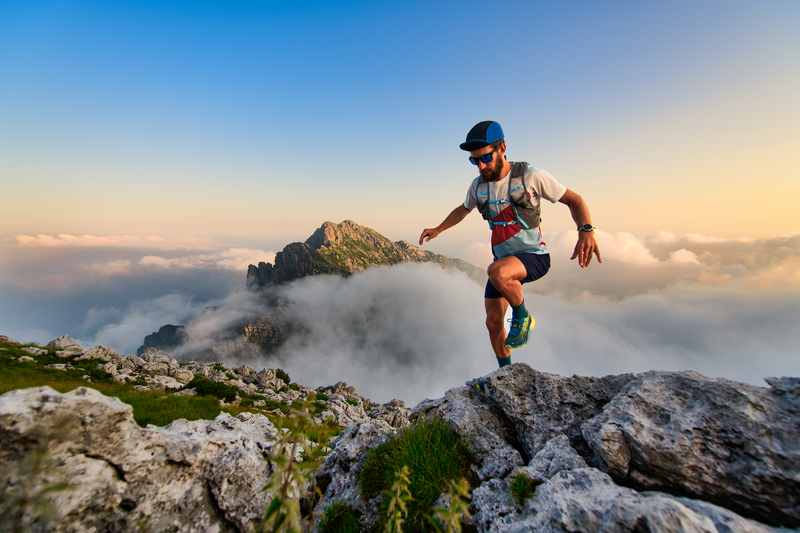
94% of researchers rate our articles as excellent or good
Learn more about the work of our research integrity team to safeguard the quality of each article we publish.
Find out more
ORIGINAL RESEARCH article
Front. Environ. Sci. , 17 February 2015
Sec. Interdisciplinary Climate Studies
Volume 3 - 2015 | https://doi.org/10.3389/fenvs.2015.00008
A commentary has been posted on this article:
Commentary: Biogeochemical analysis of ancient Pacific Cod bone suggests Hg bioaccumulation was linked to Paleo sea level rise and climate change
Deglaciation at the end of the Pleistocene initiated major changes in ocean circulation and distribution. Within a brief geological time, large areas of land were inundated by sea-level rise and today global sea level is 120 m above its minimum stand during the last glacial maximum. This was the era of modern sea shelf formation; climate change caused coastal plain flooding and created broad continental shelves with innumerable consequences to marine and terrestrial ecosystems and human populations. In Alaska, the Bering Sea nearly doubled in size and stretches of coastline to the south were flooded, with regional variability in the timing and extent of submergence. Here we suggest how past climate change and coastal flooding are linked to mercury bioaccumulation that could have had profound impacts on past human populations and that, under conditions of continued climate warming, may have future impacts. Biogeochemical analysis of total mercury (tHg) and δ 13C/δ15N ratios in the bone collagen of archeologically recovered Pacific Cod (Gadus macrocephalus) bone shows high levels of tHg during early/mid-Holocene. This pattern cannot be linked to anthropogenic activity or to food web trophic changes, but may result from natural phenomena such as increases in productivity, carbon supply and coastal flooding driven by glacial melting and sea-level rise. The coastal flooding could have led to increased methylation of Hg in newly submerged terrestrial land and vegetation. Methylmercury is bioaccumulated through aquatic food webs with attendant consequences for the health of fish and their consumers, including people. This is the first study of tHg levels in a marine species from the Gulf of Alaska to provide a time series spanning nearly the entire Holocene and we propose that past coastal flooding resulting from climate change had the potential to input significant quantities of Hg into marine food webs and subsequently to human consumers.
The Gulf of Alaska is bordered on the west by Kodiak Island and the Alaska Peninsula and on the southeast by the Alexander Archipelago. Natural and human systems have intersected here for over 7500 years, with interactions accessible through archeological, geological and biogeochemical methods (Jordan, 2001; Gehrels, 2010; Hu et al., 2010). Faunal remains from coastal archeological sites indicate that people were focused on procuring marine resources from the time of initial settlement in the region (Yesner, 1998). Many sites display long sequences of archaeofauna, including Pacific Cod (Gadus macrocephalus). Today a commercially valuable species, Pacific Cod are significant as a component of the ecosystem and because of their broad distribution on the shelf in the North Pacific (Beamish, 2008). Their skeletal remains, preserved in archeological deposits, offer, through biogeochemical analyses, the potential to reflect past ecosystem changes and a window into climate-related impacts on marine food webs.
Stable carbon and nitrogen isotope ratios (δ13C/δ15N) in the bone collagen and other hard tissues of marine species serve as proxies for primary productivity and food web interactions. The δ13C values are linked to primary productivity by fractionation due to the photosynthetic rate in phytoplankton (Laws et al., 1995) and differing ocean productivity regimes (Bidigare et al., 1997). Temporal changes in the δ13C in whale baleen from the Bering Sea and the bone collagen of pinnipeds from the North Pacific appear to track changes in marine productivity (Schell, 2000; Hirons et al., 2001), and the former possibly indicate changes in sea-ice cover (McRoy et al., 2004). Changes in the length of the food web are reflected in the δ15N values in tissues of marine vertebrates (Minagawa and Wada, 1984), including bone collagen. Periods of very high or low productivity may alter the length of food webs by increasing or decreasing available food, and may alter the trophic level of marine organisms as measured by δ15N (Fry and Sherr, 1984; Hobson and Welch, 1992). Depending upon the trophic position of a consumer within a marine food web, exposure to Hg varies (Selvaraj et al., 1997; Hsu et al., 2006). The comparison of Hg exposure risk over time and space is an important environmental health issue for both wildlife and people (Dehn et al., 2006). In humans there is a correlation between exposure to Hg and behavioral changes, including central nervous system deficit affecting fetal development and growth of young (Walker, 2014). Mercury is readily absorbed through the respiratory and gastrointestinal tracts; it bioaccumulates and is subject to biomagnification by trophic transport from lower to higher levels (Atwell et al., 1998; Dorea, 2008; Dunlap et al., 2011; Stern et al., 2012).
Besides being emitted from various locations in temperate latitudes and via industrial enterprise, Hg is naturally present in Alaskan and Siberian mountainous formations and sediments (Sunderland et al., 2009). Mercury ores occur in orogenic belts around relatively young mountains, hot springs or volcanic regions (Rytuba, 2003). Mercury has a crustal abundance of approximately 40–80 ppb but ore deposits can exceed 0.1% mercury. Mercury may become bioavailable in the marine environment through a number of natural processes including flooding leading to microorganism methylation of biologically bound inorganic Hg (Stokes and Wren, 1987) and water-column methylation of inorganic Hg in polar waters (Lehnherr et al., 2011).
Mercury concentrations can be determined in archeological specimens of bone, hair, fur, and teeth (Gerlach et al., 2006; Outridge et al., 2009). We sampled angular bones from individual ancient Pacific Cod recovered from archeological deposits at the XMK-030 site, located on a small island in Shelikof Strait, Gulf of Alaska (Figure 1), for Hg. Twenty of these bones were analyzed for both Hg and δ13C/δ15N. An additional 25 were analyzed for just Hg and additional 8 for just ratios of δ13C/δ15N. We also analyzed Hg concentrations in muscle tissue of 63 modern Pacific Cod from the same region.
Figure 1. Figure shows the extent of the shelf area formed after deglaciation and the location of the study area. XMK-030 is a small island located in the Shelikof Strait of the Gulf of Alaska. It was home to human inhabitants for nearly 7000 years. Archeological samples discussed here were recovered from XMK-030 by archeologists from the US National Park Service. Bathymetric map courtesy of NOAA Pacific Marine Envirnmental Laboratory, inset map showing the project area drafted by M. Hilton.
The archeologically recovered bones sampled were associated with radiometrically-dated strata in the XMK-030 deposits (Table 1). The lower section of the site which is a compact sediment matrix has many stratigraphically distinct human occupation floors, some attached to shell and bone middens, with an age range from 7600 to 4100 rcy BP. These deposits are overlain by a meter of sterile Aeolian sand, in turn, capped by a three-meter-thick loose shell and bone midden deposited between 2010 and 370 rcy BP.
Table 1. Pacific Cod archeological bone samples: provenience, biogeochemical data and associated radiocarbon samples.
We measured ratios of δ13C/δ15N and Hg concentrations in an effort to identify change, if any, in marine production, food web and Hg accumulation, from the early Holocene to the present. Methods for δ13C/δ15N analysis and Hg analysis are also described elsewhere (Hirons, 2001; Rothschild and Duffy, 2005; Dunlap et al., 2011).
Bone samples were well preserved and free of humus and tissues, collagen was extracted following the procedure described in detail in Hirons (2001). Approximately 0.5 g of bone was sonicated and the lipids were removed with a methanol/chloroform procedure before being demineralized. The bone samples were allowed to demineralize in 1N HCl for approximately 4 days at 5°C; fresh acid was added to the samples every day. The remaining collagen matrix was then rinsed in deionized water until a neutral pH was reached. Each sample was heated in deionized water below boiling temperature to dissolve the collagen and precipitate the peptides. The solution was passed through a 0.45 μ filter and filtrate was dried in a lypholizer for 24 h, until the collagen had thoroughly dried.
Subsamples of each tissue (0.2–0.4 mg) were combusted and analyzed for stable isotope rations with a Thermo-Finnigan Delta Plus isotope ratio mass spectrometer. Replicability of standards and samples was ≤0.20/00 for both δ13C and δ15N. Stable isotope ratios were expressed in the following standard notation:
Where X is 13C or 15 N and Rsampleis the 13C/12C or 15N/14N respectively. Rstandard for 13C is Pee Dee Belemnite; for 15N it is atmospheric N2(air).
All sample processing was conducted at the University of Alaska Fairbanks and the mass spectrometric analysis of the samples was done at the Alaska Stable Isotope Facility.
Bones were washed with detergent free of Hg and trace metals, rinsed with r.o. water, dried in a drying oven. Samples were shipped to Frontier Geosciences, Inc. for Hg analysis. Samples were analyzed by standard Cold Vapor Atomic Fluorescence Spectroscopy after standard digestion in 70% nitric acid followed by dilution with 10% bromine chloride and reduction with tin chloride (Gerlach et al., 2006).
Muscle tissue dry weight was determined in samples which were collected, dried, and placed in 40 mL certified; pre-cleaned quartz glass sample vials. These were stored a −20°C until analysis. Samples were digested with 70% HNO3/30% H2SO4 in the vial and heated until all soft tissue was dissolved. After cooling, the digests were diluted with 10% 0.2 N BrCl. Fort, aliquots of digests were reduced with SnCl2, followed by Cold Vapor Automatic Fluorescence (CVAF) detection (Rothschild and Duffy, 2005). Calibration curves were constructed to assess the accuracy of tHg determination; certified dogfish tissue (DORM-2) form the National Research Council of Canada was analyzed. A check standard and a blank were run after every 10 samples.
The results of the δ15N isotope analysis indicate that the archeologically recovered cod showed little to no change in trophic position over time. This position is consistent with data from modern cod which feed epibenthically and/or demersally (Hobson and Welch, 1992; Yang, 2004). By contrast, carbon isotopes signatures become heavier over time, a likely result of flooding of the shelf and a transfer from an oceanic productivity regime to a shelf system (Figure 2). The increase in δ13C of organic carbon may have resulted from an increase in phytoplankton growth rates (Laws et al., 1995) or a change from pelagic to a more benthic foraging regime as sea level changed throughout the Holocene (France, 1995). For example, in the Bering Sea the productivity of the shelf sea is 20–40 times higher than the adjacent oceanic basin waters (Springer et al., 1996). The shelf sea was entirely formed by sea level rise over the early Holocene and sea level in the study area continued rising until about 4000 years ago (Mann et al., 1998), leading to an increse in coastal margin productivity (Day et al., 2007).
Figure 2. δ13C/δ15N values for Pacific cod. Figure shows δ values plotted vs. time with little to no change in δ15N and a trend to heavier δ13C values from the early Holocene (7000 rcy BP) to the more recent (ca 0.578 rcy BP). Total number of samples = 28.
The δ13C records of planktonic and benthic foraminifera from southern Bering Sea sediment cores indicated a change in ocean temperature and salinity consistent with intermediate water formation resulting from deglaciation (Gorbarenko, 1996). Carbon enriched sediment, resulting from C3 and possibly also C4 plants growing in the steppe-tundra region of the emergent shelf during glaciation, were transported across the continental shelf and Bering Sea basin, and settled to the benthos throughout the Holocene, thereby further enriching the organic carbon of the shelf community. The extent of C4 plant distribution in Beringia is unclear although there are several species present today, and there is debate as to whether they may have been more common prior to deglaciation (Wooller et al., 2007).
The results of the Hg analysis (Figure 3) show an unexpected trend from high concentrations in the early/mid Holocene (ca. 52–4600 rcy BP) to low concentrations of approximately current crustal levels after about 1000 ybp. By this time sea level has long since stabilized but this is still prior to modern global anthropogenic contamination (Outridge et al., 2009). According to Nechaev et al. (1994), northeastern Bering Sea sediments also have a heavy mineral composition predominantly from volcanic sources within the region.
Figure 3. Trend in tHg concentrations in bone of Pacific cod. There is a decline in mean tHg concentrations from pre 5000 rcy BP. Total number of specimens = 45 archaeological, 63 modern. Crust values and modern cod muscle values are included as baseline references for the archeological cod values, while acknowleging that values across tissue types and over time may not be directly correlated. Cod predating 1437 rcy BP exhibit Hg concentrations that are higher than the crust values (dashed line) for the study area (Boehm, 2001). Bars indicate ng/g for total mercury ranging from 700 to 40 ng/g.
Hg concentrations in the muscle of modern cod from the Shelikof Strait region show tissue values in the range of 0.25–0.5 ppm, in agreement with other studies (Burger and Gochfeld, 2007). While Pacific cod muscle tissue is not directly comparable to the cod bone, average Hg concentrations in the soft tissue of waterfowl may be up two times higher than in bone (Rothschild and Duffy, 2005) while other studies indicate that there is a correlation between hard tissue (teeth) and soft-tissue Hg concentrations in some mammals (Eide and Wesenberg, 1993; Outridge et al., 2000). The inclusion of the cod muscle Hg data here is intended to provide some comparative modern baseline for the archeological materials. No Hg values are available for modern cod bone and as of yet there are no comparative tissue studies for fish that include bone but, Hg mercury levels in Pacific cod are reported elsewhere in concentrations appropriate to the cod's trophic level (Burger and Gochfeld, 2007).
We suggest that concentrations of Hg in Pacific Cod have fluctuated through the Holocene in concert with major paleoclimatic events, and more recently with increased anthropogenic inputs of Hg into the environment (Outridge et al., 2009). The main driver of Hg bioaccumulation in the early Holocene is proposed to be the methylation of Hg caused by the innundation of vegetation and soils across vast tracts of land at the end of the Pleistocene and through the early Holocene as a result of sea level rise due to deglaciation. A smaller-scale example of this process occurs when rivers are dammed to form hydroelectric reservoirs (Dmytriw et al., 1995). The large freshwater impoundments flood the land and lead to high levels of Hg in fish and other species (Brinkmann and Rasmussen, 2010). In this instance, we find high levels in an important subsistence species, Pacific Cod, suggesting a potential Hg exposure to prehistoric human populations not previously recognized.
Our data show Hg in the oldest cod bones occurs in concentrations approximating those in the flesh of modern cod. This is a previously unrecognized source of Hg to human consumers during the early Holocene. The concentrations in our samples fall to approximately the crustal level of Hg after about 1000 rcy BP (Figure 3). By this time sea level has long since stabilized to a still stand. This is still prior to modern global anthropogenic contamination (Outridge et al., 2009). These high concentrations early in the Holocene cannot be linked to any anthropogenic source of Hg. Elsewhere in the new world impacts from anthropogenic Hg associated with mining appear as early as 2400 years ago, but were localized until probably the fifteenth century (Cooke et al., 2011). Methlyation of biologically bound inorganic Hg and water-column methylation of inorganic Hg are the likely causes, driven in large part by sea level rise (Stokes and Wren, 1987; Boehm, 2001).
Mercury continues to be a health issue, and with industrial sources coupled with atmospheric transport and climate change, the potential to impact global food webs and the concomitant risk to human consumers in the coming decades are not insignificant. Based on variable projections of sea level rise under continued conditions of climate change (Grinsted et al., 2010) we suggest that Hg methylation from currently terrestrially bound sources should be factored into future projections of Hg bioavailability and impact to human and marine ecosystem health.
The authors declare that the research was conducted in the absence of any commercial or financial relationships that could be construed as a potential conflict of interest.
This research was supported by NSF Award #0525275. Holly McKinney assisted with the selection of archeological Pacific Cod remains for analysis and assisted with some of the stable isotope sample preparation.
Atwell, L., Hobson, K. A., and Welch, H. E. (1998). Biomagnification and bioaccumulation of mercury in an arctic marine food web: insights from stable nitrogen isotope analysis. Can. J. Fish. Aquat. Sci. 55, 1114–1121. doi: 10.1139/f98-001
Beamish, R. J. (ed.). (2008). Impacts of Climate and Climate Change in the Fisheries in the North Pacific. North Pacific Marine Science Organization (PICES).
Bidigare, R. R., Fluegge, A., Freeman, K. H., Hanson, K. L., Hayes, J. M., Hollander, D., et al. (1997). Consistent fractionation of C-13 in nature and in the laboratory: growth-rate effects in some haptophyte algae. Global Biogeochem. Cycles 11, 279–292. doi: 10.1029/96GB03939
Pubmed Abstract | Pubmed Full Text | CrossRef Full Text | Google Scholar
Boehm, P. D. (2001). Sediment Quality in Deposition Areas of Shelikof Strait and Outermost Cook Inlet, Final Report. Anchorage, AK: U.S. Department of the Interior, (Minerals Management Services), Alaska Outer Continental Shelf Region.
Brinkmann, L., and Rasmussen, J. B. (2010). High levels of mercury in biota of a new Prairie irrigation reservoir with a simplified food web in Southern Alberta, Canada. Hydrobiologia 641, 11–21. doi: 10.1007/s10750-009-0050-0
Burger, J., and Gochfeld, M. (2007). Risk to consumers from mercury in Pacific cod (Gadus macrocephalus) from the Aleutians: fish age and size effects. Environ. Res. 105, 276–284. doi: 10.1016/j.envres.2007.05.004
Pubmed Abstract | Pubmed Full Text | CrossRef Full Text | Google Scholar
Cooke, C. A., Balcom, P. H., Kerfoot, C., Abbott, M. B., and Wolfe, A. P. (2011). Pre-colombian mercury pollution associated with the smelting of Argentiferous Ores in the Bolivian Andes. Ambio 40, 18–25. doi: 10.1007/s13280-010-0086-4
Pubmed Abstract | Pubmed Full Text | CrossRef Full Text | Google Scholar
Day, J. W., Gunn, J. D., Folan, W. J., Alejandro, Y.-A., and Horton, B. P. (2007). Emergence of complex societies after sea level stabilized. EOS Trans. 88, 169–170. doi: 10.1029/2007EO150001
Dehn, L. A., Follmann, E. H., Thomas, D. L., Sheffield, G. G., Rosa, C., Duffy, L. K., et al. (2006). Trophic relationships in an Arctic food web and implications for trace metal transfer. Sci. Total Environ. 362, 103–123. doi: 10.1016/j.scitotenv.2005.11.012
Pubmed Abstract | Pubmed Full Text | CrossRef Full Text | Google Scholar
Dmytriw, R., Mucci, A., Lucotte, M., and Pichet, P. (1995). The partitioning of mercury in the solid components of dry and flooded forest soils and sediments from a hydroelectric reservoir, Quebec (Canada). Water Air Soil Pollut. 80, 1099–1103. doi: 10.1007/BF01189770
Dorea, J. G. (2008). Persistent, bioaccumulative and toxic substances in fish: human health considerations. Sci. Total Environ. 400, 93–114. doi: 10.1016/j.scitotenv.2008.06.017
Pubmed Abstract | Pubmed Full Text | CrossRef Full Text | Google Scholar
Dunlap, K. L., Reynolds, A. J., Gerlach, S. C., and Duffy, L. K. (2011). Mercury interferes with endogenous antioxidant levels in Yukon River subsistence-fed sled dogs. Environ. Res. Lett. 6:044015. doi: 10.1088/1748-9326/6/4/044015
Eide, R., and Wesenberg, G. (1993). Mercury contents of indicators and target organs in rats after long-term, low-level, mercury vapor exposure. Environ. Res. 61, 212–222. doi: 10.1006/enrs.1993.1065
Pubmed Abstract | Pubmed Full Text | CrossRef Full Text | Google Scholar
France, R. L. (1995). C-13 Enrichment in Benthic Compared to Planktonic Algae - Foodweb Implications. Mar. Ecol. Prog. Ser. 124, 307–312. doi: 10.3354/meps124307
Fry, B., and Sherr, E. B. (1984). Delta C-13 measurements as indicators of carbon flow in marine and fresh-water ecosystems. Contrib. Mar. Sci. 27, 13–47.
Gehrels, R. (2010). Sea-level changes since the Last Glacial Maximum: an appraisal of the IPCC Fourth Assessment Report. J. Quat. Sci. 25, 26–38. doi: 10.1002/jqs.1273
Gerlach, S. C., Duffy, L. K., Murray, M. S., Bowers, P. M., Adams, R., and Verbrugge, D. A. (2006). An exploratory study of total mercury levels in archaeological caribou hair from northwest Alaska. Chemosphere 65, 1909–1914. doi: 10.1016/j.chemosphere.2006.05.060
Pubmed Abstract | Pubmed Full Text | CrossRef Full Text | Google Scholar
Gorbarenko, S. A. (1996). Stable isotope and lithologic evidence of late-glacial and Holocene oceanography of the northwestern Pacific and its marginal seas. Quat. Res. 46, 230–250. doi: 10.1006/qres.1996.0063
Grinsted, A., Moore, J., and Jevrejeva, S. (2010). Reconstructing sea level from paleo and projected temperatures 200 to 2100. Climate Dyn. 34, 461–472. doi: 10.1007/s00382-008-0507-2
Hirons, A. C. (2001). Trophic Dynamics of Pinniped Populations in Alaksan Waters using Stable Carbon and Notrigen Isotope Ratios. Ph.D., University of Alaska Fairbanks.
Hirons, A. C., Schell, D. M., and Finney, B. P. (2001). Temporal records of delta(13)C and delta(15)N in North Pacific pinnipeds: inferences regarding environmental change and diet. Oecologia 129, 591–601. doi: 10.1007/s004420100756
Pubmed Abstract | Pubmed Full Text | CrossRef Full Text | Google Scholar
Hobson, K. A., and Welch, H. E. (1992). Determination of trophic relationships within a high arctic marine food web using delta C-13 and delta N-15 analysis. Mar. Ecol. Prog. Ser. 84, 9–18. doi: 10.3354/meps084009
Hsu, M. J., Selvaraj, K., and Agoramoorthy, G. (2006). Taiwan's industrial heavy metal pollution threatens terrestrial biota. Environ. Pollut. 143, 327–334. doi: 10.1016/j.envpol.2005.11.023
Pubmed Abstract | Pubmed Full Text | CrossRef Full Text | Google Scholar
Hu, A. X., Meehl, G. A., Otto-Bliesner, B. L., Waelbroeck, C., Han, W. Q., Loutre, M. F., et al. (2010). Influence of Bering Strait flow and North Atlantic circulation on glacial sea-level changes. Nat. Geosci. 3, 118–121. doi: 10.1038/ngeo729
Jordan, J. W. (2001). Late Quaternary sea level change in Southern Beringia: postglacial emergence of the Western Alaska Peninsula. Quat. Sci. Rev. 20, 509–523. doi: 10.1016/S0277-3791(00)00101-3
Laws, E. A., Popp, B. N., Bidigare, R. R., Mahlon, C. K., and Macko, S. A. (1995). Dependence of phytoplankton carbon isotopic composition on growth rate and [CO2)aq. Theoretical considerations and experimental results. Geochim. Cosmochim. Acta 59, 1131–1138. doi: 10.1016/0016-7037(95)00030-4
Lehnherr, I., St Louis, V. L., Hintelmann, H., and Kirk, J. L. (2011). Methylation of inorganic mercury in polar marine waters. Nat. Geosci. 4, 298–302. doi: 10.1038/ngeo1134
Pubmed Abstract | Pubmed Full Text | CrossRef Full Text | Google Scholar
Mann, D. H., Crowell, A. L., Hamilton, T. D., and Finney, B. P. (1998). Holocene geologic and climatic history around the Gulf of Alaska. Arctic Anthropol. 35, 112–131.
McRoy, C. P., Gradinger, R., Springer, A., Bluhm, B., Iverson, S., and Budge, S. (2004). “Stable isotopes reveal food web shifts due to arctic sea ice decline,” in AGU 2004 Joint Assembly, Montreal. (Montreal: EOS Transactions B34A-05.).
Minagawa, M., and Wada, E. (1984). Stepwise enrichment of N-15 along food-chains - further evidence and the relation between delta N-15 and animal age. Geochim. Cosmochim. Acta 48, 1135–1140. doi: 10.1016/0016-7037(84)90204-7
Nechaev, V. P., Sorochinskaya, A. V., Tsoy, I. B., and Gorbarenko, S. A. (1994). Clastic components in Quaternary sediments of the northwest Pacific and their paleo-oceanic significance. Mar. Geol. 118, 119–137. doi: 10.1016/0025-3227(94)90116-3
Outridge, P. M., Hobson, K. A., and Savelle, J. (2009). Long-term changes of mercury levels in ringed seal (Phoca hispida) from Amundsen Gulf, and beluga (Delphinapterus leucas) from the Beaufort Sea, western Canadian Arctic. Sci. Total Environ. 407, 6044–6051. doi: 10.1016/j.scitotenv.2009.08.018
Pubmed Abstract | Pubmed Full Text | CrossRef Full Text | Google Scholar
Outridge, P. M., Wagemann, R., and Mcneely, R. (2000). Teeth as biomonitors of soft tissue mercury concentrations in beluga, Delphinapterus leucas. Environ. Toxicol. Chem. 19, 1517–1522.
Rothschild, R. F. N., and Duffy, L. K. (2005). Mercury concentrations in muscle, brain and bone of Western Alaskan waterfowl. Sci. Total Environ. 349, 277–283. doi: 10.1016/j.scitotenv.2005.05.021
Pubmed Abstract | Pubmed Full Text | CrossRef Full Text | Google Scholar
Rytuba, J. J. (2003). Mercury from mineral deposits and potential environmental impact. Environ. Geol. 43, 326–338. doi: 10.1007/s00254-002-0629-5
Schell, D. M. (2000). Declining carrying capacity in the Bering Sea: isotopic evidence from whale baleen. Limnol. Oceanogr. 45, 459–462. doi: 10.4319/lo.2000.45.2.0459
Selvaraj, K., Jonathan, M. P., and Mohan, V. R. (1997). Effects of trace metals on human health: a case study from River Cooum, Madras, India. Eng. Geol. Environ. 1–3, 2161–2165.
Springer, A. M., McRoy, C. P., and Flint, M. V. (1996). The Bering Sea Green Belt: shelf-edge processes and ecosystem production. Fish. Oceanogr. 5, 205–223. doi: 10.1111/j.1365-2419.1996.tb00118.x
Stern, G. A., Macdonald, R. W., Outridge, P. M., Wilson, S., Chetelat, J., Cole, A., et al. (2012). How does climate change influence arctic mercury? Sci. Total Environ. 414, 22–42. doi: 10.1016/j.scitotenv.2011.10.039
Pubmed Abstract | Pubmed Full Text | CrossRef Full Text | Google Scholar
Stokes, P. M., and Wren, C. D. (1987). “Bioaccumulation of mercury by aquatic biota in hydroelectric reservoirs: a review and consideration of mechanisms,” in Lead, Mercury, Cadmium and Arsenic in the Environment, eds T. C. Hutchinson and K. M. Meema (Hoboken, NJ: Wiley and Sons Ltd.), 255–257.
Sunderland, E. M., Krabbenhoft, D. P., Moreau, J. W., Strode, S. A., and Landing, W. M. (2009). Mercury sources, distribution, and bioavailability in the North Pacific Ocean: insights from data and models. Glob. Biogeochem. Cycles 23, GB2010. doi: 10.1029/2008GB003425
Walker, C. (2014). Ecotoxicology: Effects on the Natural Environment. Boca Raton, FL: CRC Press. doi: 10.1201/b16402
Wooller, M. J., Zazula, G. D., Edwards, M., Froese, D. G., Boone, R. D., Parker, C., et al. (2007). Stable carbon isotope compositions of Eastern Beringian grasses and sedges: investigating their potential as paleoenvironmental indicators. Arct. Antarct. Alp. Res. 39, 318–331. doi: 10.1657/1523-0430(2007)39[318:SCICOE]2.0.CO;2
Yang, M. S. (2004). Diet changes of Pacific cod (Gadus macrocephalus) in Pavlof Bay associated with climate changes in the Gulf of Alaska between 1980 and 1995. Fish. Bull. 102, 400–405.
Keywords: mercury, stable isotopes, Bering Sea, coastal flooding, Holocene, climate change, sea level
Citation: Murray MS, McRoy CP, Duffy LK, Hirons AC, Schaaf JM, Trocine RP and Trefry J (2015) Biogeochemical analysis of ancient Pacific Cod bone suggests Hg bioaccumulation was linked to paleo sea level rise and climate change. Front. Environ. Sci. 3:8. doi: 10.3389/fenvs.2015.00008
Received: 09 December 2014; Accepted: 30 January 2015;
Published online: 17 February 2015.
Edited by:
Govindasamy Agoramoorthy, Tajen University, TaiwanReviewed by:
Peddrick Weis, Rutgers-New Jersey Medical School, USACopyright © 2015 Murray, McRoy, Duffy, Hirons, Schaaf, Trocine and Trefry. This is an open-access article distributed under the terms of the Creative Commons Attribution License (CC BY). The use, distribution or reproduction in other forums is permitted, provided the original author(s) or licensor are credited and that the original publication in this journal is cited, in accordance with accepted academic practice. No use, distribution or reproduction is permitted which does not comply with these terms.
*Correspondence: Maribeth S. Murray, Arctic Institute of North America, University of Calgary, ES 1040, 2500 University Drive NW, Calgary, AB T2N 1N4, Canada e-mail:bXVycmF5bUB1Y2FsZ2FyeS5jYQ==
Disclaimer: All claims expressed in this article are solely those of the authors and do not necessarily represent those of their affiliated organizations, or those of the publisher, the editors and the reviewers. Any product that may be evaluated in this article or claim that may be made by its manufacturer is not guaranteed or endorsed by the publisher.
Research integrity at Frontiers
Learn more about the work of our research integrity team to safeguard the quality of each article we publish.